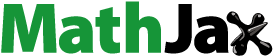
Abstract
Coronaviruses emerged three times in the last two decades and became a source of concern globally. Humulus lupulus plant has been used widely in medical science. Our objective in this study was to determine the effects of the crude extract of H. lupulus in inhibiting MERS-CoV and SARS-CoV-2 viruses’ replication in vitro using Vero E6 cell lines and predict the antiviral activity of its identified compounds against the receptor binding (RBD) protein of both viruses in silico. We determined the concentration of the extract that induced less than 50% cell toxicity (CC50), and the antiviral activity based on IC50 and plaque reduction assay. We used molecular docking simulation to predict the potential of known active compounds in H. lupulus to inhibit the RBD protein. H. lupulus extract showed very low toxicity on Vero E6 cells with CC50= 23.25 µg/µL and antiviral activity toward MERS-CoV and SARS-CoV-2 with IC50= 0.18 and 0.9 µg/µL, respectively. The crude extract showed inhibition rate of 84.6% with MERS-CoV and 80% with SARS-CoV-2. In silico analysis predicted the compounds 5′-prenylxanthohumo, xanthogalenol, dehydrocycloxanthohumol hydrate, 6-prenylnaringenin, isoxanthohumol, catechin gallate, epicatechin gallate, 8-prenylnaringenin and xanthohumol to inhibit MERS-CoV and SARS-CoV-2 invasion of host cells by interfering with viral spike protein and the host cell receptor recognition process. Drug likeness and toxicity risk prediction analysis confirmed their capability as potential drugs. Based on our findings, isolation, purification and testing of the suggested active compounds could lead to novel anti-coronavirus drugs.
Introduction
Respiratory infections are a significant source of morbidity and mortality worldwide. One of the major causes of respiratory diseases in humans are coronavirus infections. In the last three decades, the Coronaviridae family has witnessed newly emerging viruses every decade, beginning with Severe Acute Respiratory Syndrome Coronavirus 1 (SARS-CoV) (2002–2003), then Middle–East Respiratory Syndrome Coronavirus (MERS-CoV) (2012) epidemic, and finally, the Severe Acute Respiratory Syndrome Coronavirus 2 (SARS-CoV-2) (2019) pandemic [Citation1].
MERS-CoV is a zoonotic respiratory disease that is known to circulate in camels, bats and humans. The MERS-CoV virus was first detected in kingdom of Saudi Arabia in April 2012, and is still circulating there, with the last World Health Organization (WHO) report of 4 more cases as of 31st October 2022 [Citation2,Citation3]. Since the first reported case, 2600 cases have been reported to the WHO in 27 countries. South Korean MERS-CoV was first detected in 2015 and formed around 15% of the total number of cases [Citation4]. The fatality rate among reported cases represents more than 35% of infected cases, and the majority of infection and death cases are reported from the Kingdom of Saudi Arabia [Citation3]. Many MERS-CoV-specific vaccines and therapies are in development, but there are still no available vaccines or specific treatments.
Coronavirus disease 2019 (COVID-19) was identified as a new disease in 2019, and led to the spread of SARS-CoV 2 and the start of a new pandemic. SARS-CoV-2 was first detected in December 2019 in Wuhan, China, and spread rapidly across the world, creating an urgent public health crisis with nearly 525 million infected worldwide. So far, about 6.5 million (1.25%) of the patients with confirmed SARS-CoV-2 infection died. SARS-CoV-2 causes infections of not only the upper and lower respiratory tract [Citation5,Citation6], but also affects the other organs, such as eyes, brain, intestine, liver and kidneys of infected people [Citation7–9].
Hops are the seed cones of the plant Humulus lupulus, which belongs to family Cannabaceae. The family includes about 170 species grouped in 11 genera, including Cannabis (hemp, marijuana), Humulus (hops) and Celtis (hackberries) [Citation10]. Representatives of this family contain pharmaceutically active compounds, such as humulone, lupulone and xanthohumol. H. lupulus and its polyphenolic components have interesting biological properties, including antioxidant, antimicrobial, anti-inflammatory and chemo-preventive activities. While the antimicrobial properties of crude hop extracts and their ingredients are well-known, little is known about their antiviral properties [Citation11–16].
In this study, we tested the antiviral activity of the total extract of Hop (H. lupulus) against two coronaviruses, SARS-CoV-2 and MERS-CoV, using in vitro assays. Then, we performed in silico studies for the known active ingredients against SARS-CoV-2 and MERS-CoV targeting the surface spike glycoprotein, because entry inhibitors could reduce viral ability to infect new cells and thus reduce the viral transmission within a population, making them useful for both therapeutic and preventive purposes [Citation17].
Materials and methods
Viruses
MERS-CoV (MERS-CoV-NRCE-HKU270/2013) [Citation18] and SARS-CoV-2 (hCoV-19/Egypt/NRC-03/2020) [Citation19] viruses were used to determine the antiviral activity of the water extract of H. lupulus under conditions of biosafety level 3 (BSL-3) at the National Research Centre (NRC) in Egypt.
SARS-CoV-2 strain hCoV-19/Egypt/NRC-03/2020 (GISAID accession number: EPI_ISL_430819) was isolated in Vero-E6 cells (ATCC No. CRL-1586) from an oropharyngeal swab specimen collected from a 34-year-old Egyptian woman on 18 March 2020. MERS-CoV strain (MERS-CoV-NRCE-HKU270/2013) was isolated in NRC from infected camels in Egypt. Both viruses were purified using plaque assay and passaged twice in Vero-E6 cells to generate Passage 2 virus stock in the presence of L-1-tosylamido-2-phenylethyl chloromethyl ketone (TPCK)-treated trypsin at the Center of Scientific Excellence for Influenza Viruses (CSEIV, NRC).
Cell line
The African green monkey kidney (Vero E6) cells were kindly provided by Dr. Richard Webby, St. Jude Children’s Research Hospital, Department of Virology and Molecular Biology, USA. Cells were cultivated as a confluent monolayer in 75 cm2 tissue culture flasks in Dulbecco’s Modified Eagle’s Medium (DMEM)_ supplemented with Gibco™ Antibiotic-Antimycotic (100X) (cat. no. 15240062, ThemoFisher Scientific, USA). This mixture is composed of 10,000 U of penicillin G sodium, 10,000 µg of streptomycin sulfate and 25 µg of amphotericin B in 100 mL. Then we added 10% fetal bovine serum (FBS). Vero E6 cells were propagated till confluence for several passages, harvested in aliquots, and then stored in liquid nitrogen until use.
Plant material
H. lupulus whole plants were purchased from a herbal store in Riyadh, Saudi Arabia. The plants were identified and characterized by Prof. Abdo Marei Hamed, Professor of Plant Ecology, Botany Department, Al-Azhar University.
Plant extract
The water extract of H. lupulus was prepared according to the established protocol at CSEIV as previously described [Citation20]. In this study, 3 g of H. lupulus herbal powder from the dried whole plant was weighed and mixed with 40 mL of distilled water and boiled for 2-3 min. The boiled extract was covered and left to cool at room temperature in sterile conditions. The extract was then purified by centrifugation at 3000 rpm for 3 min to obtain the extract in a pure non-turbid form, and then the water was evaporated in sterile conditions. Finally, the dried extract was weighed and resolubilized in an equal weight/volume (W/V) of distilled water.
Virus titration and median tissue culture infectious dose (TCID50)
To determine the virus dose capable of infecting 50% of the cells (TCID50), Vero E6 cells were growing in a 96-well plate (100 µL/well at a density of 3 × 105 cells/mL) in DMEM supplemented with 10% FBS and 2% antibiotic/antimycotic mixture, and incubated overnight at 37 °C with 5% CO2. At post confluence, 1 log serial dilutions of the virus stock (in replicates), were prepared in infection/maintenance media (DMEM (BioWhittaker, Walkersville, MD, USA), supplemented with 4% Bovine serum albumin (BSA), 2% antibiotic antimycotic mix, and TPCK). The dilutions were used in infecting cell monolayers (8 replica). The virus inoculum was then removed after 1 h post infection and replaced with 160 µL of infection media in each well, incubated for 72 h. The TCID50 value was calculated using the Reed and Muench method by observing and counting the cytopathic effect (CPE) in each well, calculated as the rounding and detachment of infected cells [Citation21,Citation22]. Results of virus’s titration were confirmed with staining and OD reading as follows, infected and control cells were treated with 10% formaldehyde for fixation and incubated for 2 h at room temperature. After washing, cells were stained with (0.5%) crystal violet solution and visualized by dissolving the stain with absolute methanol. An ELISA reader was used to measure the optical density (OD) of the 96-well plate at 540 nm and the reference OD at 620 nm. The virus 50% TCID was determined in accordance with the cell control.
Cytotoxicity assay (CC50)
The cytotoxicity was determined according to Feoktistova et al. [Citation23,Citation24] and Mahmoud et al. [Citation23,Citation24] with minor modifications. In a 96-well plate, infection medium was distributed to all wells from row 2 to 12, and 180 µL to wells in row 1. Then 20 µL of prepared stock solution of the plant extract was added to row 1 (from A1 to H1) to obtain a 10-fold dilution (1:10) of the stock plant extract in the first row, then 2-fold serial dilutions were prepared in column 2 to column 10. Therefore, 8 replicas for each dilution were prepared.
Vero-E6 cells were cultivated in 96-well tissue culture plates 24 h prior to treatment. Then 50 µL of each dilution was used to treat the Vero-E6 monolayers at 90% confluency, with incubation for 48–72 h. Cells were then fixed by formaldehyde and stained with crystal violet as previously described [Citation23]. The cytotoxicity was calculated using the following equation: Cytotoxicity = (OD of cells without treatment − OD of cells with treatment)/(OD of cells without treatment) × 100. The measurements were performed at λmax 540 nm with 690 nm as a reference wavelength using a multi-well plate reader.
This was applied also for hydroxychloroquine and favipiravir as positive controls; they were obtained as raw active ingredients from the Egyptian Medicines Authority pharmacy. Each of them was prepared at a concentration of 1 mg/mL and dilutions were made. The stock solutions of the two drugs were prepared in dimethyl sulfoxide (DMSO), 150 µg/mL.
Antiviral activity (IC50)
The procedure was performed as previously described with small modifications [Citation25,Citation26]. Different concentrations of the plant extract along with hydroxychloroquine and favipiravir (as control drugs) were prepared (100 µL) and each dilution was then mixed with 100 TCID50 (100 µL of dilution 103.25) of the SARS-CoV-2 virus (virus titer was TCID50 = 10 5.25/mL), or with 100 TCID50 (100 µL of dilution 102.75) of the MERS-CoV virus (virus titer was TCID50 = 10 4.75/mL) . Then, the mixture was incubated for 1 h at 37 °C. Confluent monolayers of Vero E6 cells in a 96-well plate were treated with 100 µL of the mix (8 replica) and incubated for 72 h at 37 °C with 5% CO2. Cells were then treated with 10% formaldehyde for fixation then stained with crystal violet staining solution (1% crystal violet and 20% methanol in distilled water). OD was measured as previously described. The mean and the standard error of the mean were calculated for at least three independent experiments, and the antiviral activity was calculated according to the following equation:
where ODtv is the absorbance of the tested extract with virus-infected cells; ODcv is the absorbance of the virus-infected cells only; ODcd is the absorbance of the control cells.
Virus titration by plaque assay
Virus titration was performed in 6-well cell culture plates seeded with 105 cells/mL Vero-E6 cells as previously described [Citation27,Citation28]. Ten-fold serial dilutions of SARS-CoV-2 and MERS-CoV virus stocks were prepared, then used to infect the cells. After 60 min of incubation, the inoculum was removed and semisolid overlayer was added; it was prepared by mixing 2x infection medium mixed with 2x agarose (to obtain a final concentration of 1x). Then, 3 mL of this mix were added to each well. Plates were then incubated at 37 °C for 72 h in 5% CO2. Formalin (10%) was added for 2 h, and then staining was performed with 0.1% crystal violet. Finally, the plaques were counted, and the plaque-forming units (PFU) were calculated.
Plaque reduction assay
The plaque reduction assay was carried out for the plant extract and hydroxychloroquine and favipiravir according to the method described by Kandeil et al. [Citation27]. Vero E6 cells (105 cells/mL) were cultivated in six-well plates for 24 h at 37 °C. The MERS-CoV or SARS-CoV-2 virus stocks were diluted to give countable plaques at 103 dilution and mixed with the nontoxic concentrations of the tested extract based on the cytotoxicity assay. The samples were incubated for 1 h at 37 °C before being added to the Vero E6 cells. The growth medium was removed from the cell culture plates, and then mixtures of the virus and plant extract were added (100 µL/well). After 1 h of virus adsorption, 3 mL of DMEM supplemented with 2% agarose was added onto the cell monolayer [Citation29] and the plates were left to solidify. Then, the plates were incubated at 37 °C for 3 days until the formation of viral plaques. The samples were fixed with formalin and visualized using crystal violet staining. Calculations were performed as follows:
Mechanism of action against SARS-CoV-2
To determine the step of the replication cycle of the virus at which the extract shows strongest antiviral activity, we studied the possible mechanism of hCoV-19/Egypt/NRC-03/2020 SARS-CoV-2 virus inhibition by the Hop water extract, in reference to three different assumed mechanisms. H. lupulus aqueous extract was tested at four different concentrations: 0.375, 0.75, 1.5 and 3 µg/mL, as previously described [Citation27]. The percentage of reduction in plaque formation was calculated compared to the control wells; where the virus control was untreated Vero E6 cells that were directly infected with the hCoV-19/Egypt/NRC-03/2020 SARS-CoV-2 virus.
Viral replication [Citation30]
The tested virus was diluted to give 104 PFU/well, applied directly to the cells (Vero E6), and then incubated for 1 h at 37 °C. Unabsorbed viral particles were removed by washing and the extract was applied individually at different concentrations ranging from 0.375 to 3 μg/μL. After 1 h of contact time, about 3 mL of DMEM supplemented with 2% agarose was added to the cell monolayer and visualized as previously described [Citation30].
Viral adsorption [Citation31]
Concentrations ranging from 0.375 to 3 μg/µl of the water extract were co-incubated with the cell monolayer for 90 min at 4 °C. The unabsorbed extract was removed. Then SARS-CoV-2 Virus at 104 PFU/well was further incubated with the pretreated cells for 1 h before adding the agarose/media overlayer. fixation, staining and visualization were performed as previously described [Citation31]. The percentage of reduction in plaques formation was calculated as mentioned above, in comparison to the control wells; where the untreated Vero E6 cells were directly infected with the 2020 SARS-CoV-2 virus.
Virucidal effect [Citation32]
SARS-CoV-2 virus at 104 PFU/well dilution was mixed with the different concentrations of the extract. After 1 h of contact time, 100 μl of each mixture was added to the Vero E6 cell monolayer. After 1 h of incubation, 3 ml of DMEM were overlaid and left to solidify. Incubation was done at 37 °C to allow formation of viral plaques. The plaques were fixed via 10% formalin solution for 2 h.
Molecular docking
AutoDock Vina [Citation33] was used. A total of 180 compounds reportedly identified in H. lupulus water extract were docked into the active site of the MERS-CoV receptor binding domain (RBD) (PDB ID: 4l72) and SARS-CoV-2 receptor binding domain (RBD) (PDB ID: 6LZG) [Citation34]. The chemical structures of the compounds were downloaded from the PubChem [Citation29,Citation35] database as SDF files and converted to PDB files using Pymol [Citation29]. The crystal structure of RBD was downloaded from the protein data bank [Citation36] and prepared by removing the co-crystallized ligands and water molecules. The grid box for docking simulations was constructed in such a way that it encompassed the RBD residues of interest using the graphical user interface program AutoDock 4.2 [Citation37]. Auto Dock Vina’s docking technique was utilized to find the optimal docked configuration between the ligands and the RBD protein. For each ligand, a maximum of nine complexes were examined throughout the docking procedure. Visualization and analysis of the interaction between the RBD residues and ligands were presented using Pymol and LigPlot+ [Citation29].
Sequence alignment
The primary sequences of MERS-CoV (ALJ54515.1) and SARS-CoV 2 (QII57161.1) were downloaded from the NCBI database [Citation38,Citation39] and aligned using Multalin software [Citation40] at default parameters for the identification of conserved amino acid residues. The ESPript webserver was utilized to highlight the sequence similarities [Citation40].
Drug-likeness analysis
The Lipinski rules of five were applied to assess the drug-likeness of the identified compounds in H. lupulus. The best docked compounds were submitted as SDF files to the SwissADME website to calculate their physiochemical properties [Citation38,Citation39].
Toxicity risk assessment
In order to show that the proposed chemicals would be less harmful to ingest, we also performed toxicity prediction on them. ProTox-II, a virtual laboratory for the forecast of small molecule toxicities, was used to conduct the investigation. The server received the chemicals’ Canonical Smiles, which produced data displaying the toxicity prediction, including LD50 and toxicity classes [Citation35].
Results
Cytotoxicity and antiviral activity
The water extracts of H. lupulus plant showed a broad range of safe doses for the Vero E6 cells with a CC50 of 23.255 µg/µL. The safe concentrations were then used to test the antiviral activity based on IC50, which was calculated by comparing the treated infected cells to untreated virus infected cells. The IC50 values of the water extracts of H. lupulus plant against MERS-CoV and SARS-CoV-2 were 0.186 µg/µL and 0.941 µg/µL, with selectivity index (SI) values of 125 and 24.7, respectively. As shown in , the extract had higher therapeutic potential in terms of SI against both SARS-CoV-2 and MERS-CoV than the control drugs (favipiravir and hydroxychloroquine). Hydroxychloroquine showed SI values of 21.7 against SARS-CoV-2 and 18.1 against MERS-CoV, while favipiravir showed SI values of 1.6 (SARS-CoV-2) and 3.32 (MERS-CoV), .
Figure 1. Cytotoxicity and inhibitory effect of H. lupulus crude extract on Vero E6 cells. EC50 for MERS-CoV and SARS-CoV-2.
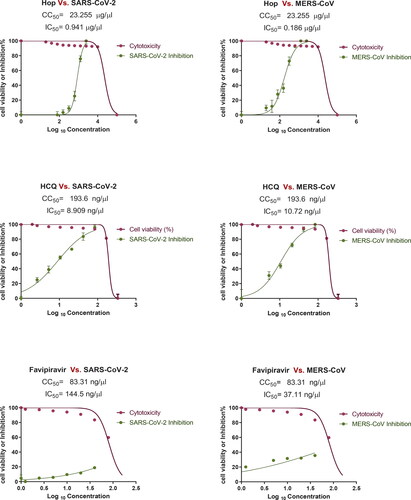
Table 1. Inhibitory effect of Hop extract against MERS-CoV and SARS-CoV-2 in VERO E6 cell line.
Antiviral activity by plaque reduction assay
The plaque reduction assay was also used to confirm the antiviral activity of the plant extract as compared to the control drugs hydroxychloroquine and favipiravir. The water extract of H. lupulus was tested at more than 90% viability safe concentrations (0.375, 0.75, 1.5, and 3 µg/µL). The extracts at 3 µg/µL showed 84.6% viral inhibition against MERS-CoV and 80% viral inhibition against SARS-CoV-2 ( and ). While hydroxychloroquine showed 100% and 85% inhibition against SARS-CoV-2 and MERS-CoV, favipiravir showed 27% inhibition against SARS-CoV-2.
Figure 2. Inhibitory effect of Hop extract against MERS-CoV and SARS-CoV-2 by plaque reduction assay.
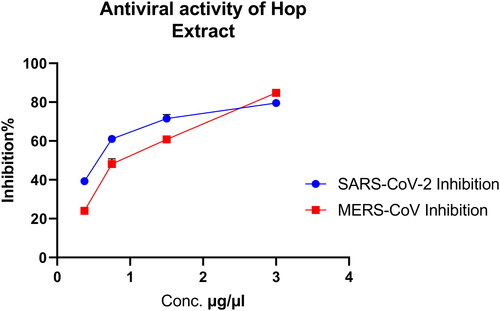
Table 2. Inhibitory effect of Hop extract against MERS-CoV and SARS-CoV-2.
Mechanism of action against SARS-CoV-2
Safe doses of hop extract were then used to determine the mechanism of inhibiting the SARS-CoV-2 virus. Virucidal mechanism showed 80% to 45% inhibition, while replication showed 70% to 27% and adsorption showed 66% to 25% inhibition ().
Molecular docking
and show the binding of ligands to the amino acid residues of MERS-CoV RBD and SARS-CoV 2 RBD . With a binding energy of −7.3 Kcal/mol, 5′-prenylxanthohumol was the best docked compound to MERS-CoV RBD. Among the best H. lupulus compounds for binding to the MERS-CoV RBD interface were (2,5-dimethylanilino) naphtho-1,2-quinone, epicatechin gallate, catechin gallate, xanthogalenol, adlupulone, 6-prenylnaringenin, quercitrin and isoxanthohumol (). Catechin gallate was the best-connected compound to the SARS-CoV 2 RBD interface residues with binding energy (−7.4 Kcal/mol). Moreover, some compounds were predicted to inhibit SARS-CoV 2 RBD attachment to hACE2, such as quercitrin, rutin, 8-prenylnaringeninm epicatechin gallate, dehydrocycloxanthohumol hydrate, xanthohumol, 5′-prenylxanthohumol and isoxanthohumol. Sequence alignment of the RBD of the two viruses () suggests that some compounds such as catechin gallate, quercitrin, epicatechin gallate, 5′-prenylxanthohumol, dehydrocycloxanthohumol hydrate and isoxanthohumol are predicted to be active against both viruses.
Figure 4. 3 D poses and 2 D intermolecular contact between the top 5 compounds and MERS-CoV RBD. Note: In the 3D figures, the RBD protein is presented as a carton model and the ligands are presented as green sticks, and in the 2D plot, the black ball indicates the carbon atom, the red ball indicates the oxygen atom, the blue ball indicates the nitrogen atom, the red eyelash lines indicate the hydrophobic interaction between ligands and, and the green dashed string indicates the hydrogen bond formed between ligands and the amino acid residues of MERS-CoV RBD.
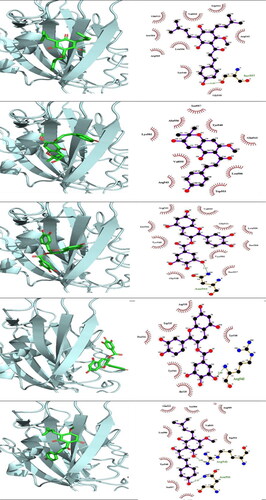
Figure 5. 3 D poses and 2 D intermolecular contact between the top 5 compounds and SARS-CoV 2 RBD. Note: In the 3D figures, the RBD protein is presented as a carton model and the ligands are presented as green sticks, and in the 2D plot, the black ball indicates the carbon atom, the red ball indicates the oxygen atom, the blue ball indicates the nitrogen atom, the red eyelash lines indicate the hydrophobic interaction between ligands and, and the green dashed string indicates the hydrogen bond formed between ligands and the amino acid residues of SARS-CoV 2 RBD.
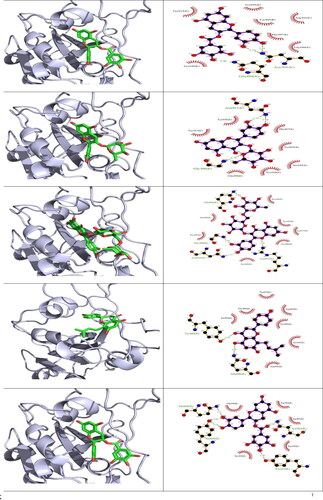
Table 3. Binding affinity, hydrogen bonds and hydrophobic interactions of compounds isolated from H. lupulus docked into SARS-CoV-2 and MERS-CoV RBD protein.
Drug likeness and toxicity prediction
Drug-likeness analysis is used to facilitate the development of novel drug compounds that obey the following Lipinski’s rules: ≤ 500 molecular weight (MW), ≤ 5 hydrogen bond donors (HBD), ≤ 10 hydrogen bond acceptors (HBA), ≤ MlogP 4.15 lipophilicity. The findings of this investigation are summarized in . The compounds 5′-prenylxanthohumo, xanthogalenol, dehydrocycloxanthohumol hydrate, 6-prenylnaringenin, adlupulone, 8-prenylnaringenin and xanthohumol showed no violation to the five parameters. Catechin gallate and epicatechin gallate only violated one parameter. In contrast, there was violation of more than two parameters for quercitrin (MW = 448.38, HBD = 11, HBA = 7) and for rutin (MW 610.52, HBD = 16, and HBA = 10), which make these compounds unfavorable for oral drug delivery. The compounds 5′-prenylxanthohumo, xanthogalenol, dehydrocycloxanthohumol hydrate, 6-prenylnaringenin, adlupulone, 8-prenylnaringenin, xanthohumol, catechin gallate and epicatechin are thought to be well absorbed by the body, which could lead to sufficient absorption by the gastrointestinal tract.
Table 4. Candidate compounds analyzed utilizing the ProTox-II server for their toxicity properties.
Toxicity risk is assessed using ProTox-II, which employs predictive models built from data from both in vitro and in vivo experiments. The toxicity risk assessment values are shown in . The LD50 for 5′-prenylxanthohumo, dehydrocycloxanthohumol hydrate and xanthohumol was 3800 mg/kg, and 5000 mg/kg for quercitrin and rutin, placing them in class 5, indicating that they may be toxic if consumed (2000 < LD50 ≤ 5000). The LD50 values of Xanthogalenol, catechin gallate and epicatechin gallate were in the 1000 mg/kg range, and those of 6-prenylnaringenin, 8-prenylnaringenin, and isoxanthohumol were in the 2000 mg/kg range, indicating that their toxicities were in the range of 300 < LD50 ≤ 2000. The LD50 of adlupulone is estimated at 260 (mg/kg) in class 3 (50 < LD50 ≤ 300). Adlupulone is the only one that is predicted to be very toxic, according to the toxicity prediction study.
Discussion
Highly pathogenic coronaviruses, especially MERS-CoV and SARS-CoV-2, remain a major global human health issue, due to the risk of a major pandemic. Therefore, studies on inhibitors or vaccines of MERS-CoV and SARS-CoV-2 have attracted worldwide attention. The use of antivirals from plant extracts is one of the effective and most accessible solutions during the current SARS-CoV-2 pandemic, and many efforts have focused on antivirals from plant extracts as potentially effective and accessible solutions [Citation20,Citation41–44]. Many previous studies have reported that plants are rich sources of simple phenols, tannins, alkaloids, saponins, lignans, anthocyanins, carotenoids, carbohydrates, flavonoids, isoflavones, phenolic acids and many more compounds. These phytochemical compounds have been proven to be responsible for multiple antimicrobial and antiviral activities [Citation42–44].
Many previous studies have shown that the H. lupulus extract contains many antimicrobial promising compounds, which could also act as candidates to combat SARS-CoV-2 and MERS-CoV [Citation11–16]. H. lupulus cones represent an important source of phenolic compounds, some of which are reported to produce inhibitory effects against several viral infections [Citation45]. Among the polyphenols identified in the HOP extract, rutin and quercetin are reported to inhibit the influenza infection in animal models, as well as the viral neuraminidase activities in vitro [Citation46], and syringic acid and gallic acid as potent anti-influenza compounds [Citation47]. This evidence supports our hypothesis about the possible involvement of the phenolic constituents in the antiviral properties of the HOP extract. Rutin, syringic acid and gallic acid appear to be the potential bioactive constituents, although the contribution of the whole phytocomplex cannot be excluded. Altogether, these compounds can act by both interfering with the virus life cycle and reinforcing the defenses of host cells, mainly counteracting the redox imbalance required for the viral infection establishment.
To the best of our knowledge, no study has examined the antiviral activity of H. lupulus extract on SARS-CoV-2 and MERS-CoV. Here, we tested the H. lupulus extract on SARS-CoV-2 and MERS-CoV, with promising results that were consistent with previous research on many different viruses. In this work, a preliminary screening was conducted to estimate the antiviral effect of H. lupulus against SARS-CoV-2 and MERS-CoV compared to control antivirals hydroxychloroquine and favipiravir. Before screening for their antiviral activity, cytotoxicity assay was first assayed by crystal violet staining assay on Vero E6 cells, which revealed a broad range of safe doses for Vero E6 cells. The selectivity index of the extract against MERS-CoV and SARS-CoV-2 was SI = 125 and 24.7 in the IC50 assay, which indicates the extract was more efficient against MERS-CoV2. SI of the extract was comparable to that of hydroxychloroquine (HCQ) on SARS-CoV-2 and around 10-fold higher in the case of MERS-CoV.
The plaque reduction assay suggested that the extract inhibited the plaque formation. The inhibition rate ranged from 40 to 80% against SARS-CoV-2, and from 23 to 85% against MERS-CoV.
The three examined modes of action (viral replication inhibition, viral adsorption inhibition or virucidal action) suggested a strong capacity of the extract to suppress viral replication, particularly the virucidal mechanism, which maintains a higher inhibitory capacity at lower concentrations. In silico studies of the previously proven active constituents of the hop extract indicated that the compounds 5′-prenylxanthohumo, xanthogalenol, dehydrocycloxanthohumol hydrate, 6-prenylnaringenin, isoxanthohumol, catechin gallate, epicatechin gallate, 8-prenylnaringenin and xanthohumol revealed potentially higher antiviral activity against SARS-CoV-2 and MERS-CoV viruses by inhibiting the viral entry process with low risk of toxicity and access to drugs with similar effects. These active ingredients formed one or more hydrogen bonds plus other interactions with the amino acids of RBD of MERS-CoV, at the amino acids essential for binding to the dipeptidyl peptidase 4 (DPP4) receptor binding region, especially the hydrogen bond with Arg542. This means interference with the binding of the RBD to the receptor and thus inhibiting the virus entry [Citation34,Citation48]. They also showed strong multiple hydrogen bonds formation with SARS-CoV-2 RBD amino acids, especially 496, 498, and 501 responsible for binding with the receptor-binding motif (RBM) of the ACE2 receptor, and thus inhibiting the viral ability to infect the cells [Citation49,Citation50].
The in-silico study revealed some compounds with potential antiviral activity that could be used against both viruses. The isolation and examination of the suggested compounds separately is needed, and further investigation is required in upcoming research.
Further studies need to determine the most efficient plant cultivation conditions and to purify the active constituents. Additional research is also required to address the role of each active compound in viral inhibition in vitro and in vivo as well as to precisely quantify and determine the chemical composition of the plant extract.
Conclusions
SARS-CoV-2 is a highly contagious virus for which a widely distributed commercial antiviral drug is not yet available. The three approved antiviral drugs for SARS-CoV-2 are expensive with very limited availability in many countries. The extracts of H. lupulus are used in many applications in pharmacy and food industry. The extract we tested here is a simple whole-plant boiled extract. The crude extract showed high safety levels and anti-corona virus efficiency by inhibiting the viral ability to infect and replicate inside the host cells. In silico studies predicted the active constituents prenylxanthohumol, xanthogalenol and quercetin to bind to the receptor-binding domain (RBD) of the spike proteins of both corona viruses. More in vitro and in vivo investigations are needed to determine the exact active ingredients of the extract and their specific mode of action.
Author contributions
Conceptualization, T.B. and M.A.; methodology, T.B. and A.M.; software, A.A.; validation, A.A., T.B. and M.A.; investigation, T.B., A.M., A.T., Y.M., M.G., and M.A.; writing—original draft preparation, T.B., A.M., A.T., Y.M., M.G., A.A., M.A., and R.B.; writing—review and editing, Y.M., T.B., A.A.; visualization, A.A.; supervision, T.B.
Acknowledgments
Thamer Ahmed Bouback offers sincere thanks, appreciation, and gratitude to Professor Mohamed Ahmed Ali for providing all necessary logistics facilities for this work. Thamer Ahmed Bouback would also like to thank Dr. Ahmed Kandeil, Brother and instructor, and all researchers and workers at the Center for Scientific Excellence at the National Research Center (NRC), Egypt. Many thanks to Bostan Al Hakeem Shop for giving us advice related to herb usage.
Data availability statement
Data sharing not applicable: All data that support the findings reported in this study are available from the corresponding author [T. B.] upon reasonable request.
Disclosure statement
No potential conflict of interest was reported by the author(s).
References
- Schwartz DA. An analysis of 38 pregnant women with COVID-19, their newborn infants, and maternal-fetal transmission of SARS-CoV-2: maternal coronavirus infections and pregnancy outcomes. Arch Pathol Lab Med. 2020;144(7):799–805.
- de Groot RJ, Baker SC, Baric RS, et al. Middle east respiratory syndrome coronavirus (MERS-CoV): announcement of the coronavirus study group. J Virol. 2013;87(14):7790–7792.
- World Health Organization. Disease Outbreak News; Middle East respiratory syndrome coronavirus (MERS-CoV) – Saudi Arabia. 2022. Available from: https://www.who.int/emergencies/disease-outbreak-news/item/2022-DON422.
- KDCA. Middle East Respiratory Syndrome (MERS). Policy & Services 2018. 2018. Available from: https://www.kdca.go.kr/contents.es?mid=a30329000000.
- Diamond MS, Kanneganti T-D. Innate immunity: the first line of defense against SARS-CoV-2. Nat Immunol. 2022;23(2):165–176.
- Banik G, Khandaker G, Rashid H. Middle east respiratory syndrome coronavirus “MERS-CoV”: current knowledge gaps. Paediatr Respir Rev. 2015;16(3):197–202.
- Jansen J, Reimer KC, Nagai JS, COVID Moonshot consortium, et al. SARS-CoV-2 infects the human kidney and drives fibrosis in kidney organoids. Cell Stem Cell. 2022;29(2):217–231.e8.
- Song E, et al. Neuroinvasion of SARS-CoV-2 in human and mouse brain. bioRxiv. 2020.
- Gavriatopoulou M, Korompoki E, Fotiou D, et al. Organ-specific manifestations of COVID-19 infection. Clin Exp Med. 2020;20(4):493–506.
- Stevens P. Angiosperm phylogeny website. 2001. Available at: http://www.mobot.org/MOBOT/research/APweb
- Di Sotto A, Checconi P, Celestino I, et al. Antiviral and antioxidant activity of a hydroalcoholic extract from Humulus lupulus. Oxid Med Cell Longev. 2018;2018:5919237.
- Abiko Y, Paudel D, Uehara O. HOPs components and oral health. J Funct Foods. 2022;92:105035.
- Blaxland J, Thomas R, Baillie L. The antibacterial effect of Humulus lupulus (HOPs) against Mycobacterium bovis BCG: a promising alternative in the fight against bovine tuberculosis? Beverages. 2022;8(3):43.
- Di Lodovico S, Menghini L, Ferrante C, et al. Hop extract: an efficacious antimicrobial and anti-biofilm agent against multidrug-resistant Staphylococci strains and Cutibacterium acnes. Front Microbiol. 2020;11:1852.
- Bocquet L, Rivière C, Neut C, et al. Antimicrobial properties of phenolic compounds from hops, Humulus lupulus L.: fight against multidrug resistance. Planta Med. 2016;81(S 01):S1–S381.
- Bocquet L, Sahpaz S, Bonneau N, et al. Phenolic compounds from Humulus lupulus as natural antimicrobial products: new weapons in the fight against methicillin resistant Staphylococcus aureus, Leishmania mexicana and Trypanosoma brucei strains. Molecules. 2019;24(6):1024.
- Seyedpour S, Khodaei B, Loghman AH, et al. Targeted therapy strategies against SARS-CoV-2 cell entry mechanisms: a systematic review of in vitro and in vivo studies. J Cell Physiol. 2021;236(4):2364–2392.
- Perera RA, Wang P, Gomaa MR, et al. Seroepidemiology for MERS coronavirus using microneutralisation and pseudoparticle virus neutralisation assays reveal a high prevalence of antibody in dromedary camels in Egypt, June 2013. Euro Surveill. 2013;18(36):pii = 20574.
- Kandeil A, Mostafa A, El-Shesheny R, et al. Coding-Complete genome sequences of two SARS-CoV-2 isolates from Egypt. Microbiol Resour Announc. 2020;9(22):e00489-20.
- GabAllah M, et al. Antiviral activity of water extracts of some medicinal and nutritive plants from the Apiaceae family. Novel Res Microbiol J. 2020;4(2):725–735.
- Kutkat O, Kandeil A, Moatasim Y, et al. In vitro and in vivo antiviral studies of new heteroannulated 1,2,3-Triazole glycosides targeting the neuraminidase of Influenza A viruses. Pharmaceuticals (Basel). 2022;15(3):351.
- Reed LJ, Muench H. A simple method of estimating fifty per cent endpoints. Am J Epidemiol. 1938;27(3):493–497.
- Feoktistova M, Geserick P, Leverkus M. Crystal violet assay for determining viability of cultured cells. Cold Spring Harb Protoc. 2016;2016(4):pdb.prot087379.
- Mahmoud DB, Ismail WM, Moatasim Y, et al. Delineating a potent antiviral activity of Cuphea ignea extract loaded nano-formulation against SARS-CoV-2: in silico and in vitro studies. J Drug Deliv Sci Technol. 2021;66:102845.
- Mostafa A, Kandeil A, A. M. M. Elshaier Y, et al. FDA-Approved drugs with potent in vitro antiviral activity against severe acute respiratory syndrome coronavirus 2. Pharmaceuticals. 2020;13(12):443.
- Seliem IA, Girgis AS, Moatasim Y, et al. New pyrazine conjugates: synthesis, computational studies, and antiviral properties against SARS-CoV-2. ChemMedChem. 2021;16(22):3418–3427.
- Kandeil A, Mostafa A, Kutkat O, et al. Bioactive polyphenolic compounds showing strong antiviral activities against severe acute respiratory syndrome coronavirus 2. Pathogens (Basel, Switzerland). 2021;10(6):758.
- Tobita K. Permanent canine kidney (MDCK) cells for isolation and plaque assay of Influenza B viruses. Med Microbiol Immunol. 1975;162(1):23–27.
- Janson G, Zhang C, Prado MG, et al. PyMod 2.0: improvements in protein sequence-structure analysis and homology modeling within PyMOL. Bioinformatics. 2017;33(3):444–446.
- Kuo Y-C, Lin L-C, Tsai W-J, et al. Samarangenin B from Limonium sinense suppresses herpes simplex virus type 1 replication in vero cells by regulation of viral macromolecular synthesis. Antimicrob Agents Chemother. 2002;46(9):2854–2864.
- Zhang J, Zhan B, Yao X, et al. Antiviral activity of tannin from the pericarp of Punica granatum L. against genital herpes virus in vitro. Zhongguo Zhong Yao Za Zhi. 1995;20(9):556–558, 576.
- Schuhmacher A, Reichling J, Schnitzler P. Virucidal effect of peppermint oil on the enveloped viruses herpes simplex virus type 1 and type 2 in vitro. Phytomedicine. 2003;10(6-7):504–510.
- Trott O, Olson AJ. AutoDock vina: improving the speed and accuracy of docking with a new scoring function, efficient optimization, and multithreading. J Comput Chem. 2010;31(2):455–461.
- Wang N, Shi X, Jiang L, et al. Structure of MERS-CoV spike receptor-binding domain complexed with human receptor DPP4. Cell Res. 2013;23(8):986–993.
- Połeć K, Barnaś B, Kowalska M, et al. The influence of the essential oil extracted from HOPs on monolayers and bilayers imitating plant pathogen bacteria membranes. Colloids Surf B Biointerfaces. 2019;173:672–680.
- Maietti A, Brighenti V, Bonetti G, et al. Metabolite profiling of flavonols and in vitro antioxidant activity of young shoots of wild Humulus lupulus L. (HOP). J Pharm Biomed Anal. 2017;142:28–34.
- Ligor M, Stankevičius M, Wenda-Piesik A, et al. Comparative gas chromatographic–mass spectrometric evaluation of hop (Humulus lupulus L.) essential oils and extracts obtained using different sample preparation methods. Food Anal Methods. 2014;7(7):1433–1442.
- Daina A, Michielin O, Zoete V. SwissADME: a free web tool to evaluate pharmacokinetics, drug-likeness and medicinal chemistry friendliness of small molecules. Sci Rep. 2017;7(1):42717–42713.
- Lipinski CA, Lombardo F, Dominy BW, et al. Experimental and computational approaches to estimate solubility and permeability in drug discovery and development settings. Adv Drug Delivery Rev. 1997;23(1–3):3–25.
- Banerjee P, Eckert AO, Schrey AK, et al. ProTox-II: a webserver for the prediction of toxicity of chemicals. Nucl Acids Res. 2018;46(W1):W257–W263.
- Guijarro-Real C, Plazas M. Potential in vitro inhibition of selected plant extracts against SARS-CoV-2 chymotripsin-like protease (3CL(Pro)) activity. Foods. 2021;10(7):1503.
- Lautié E, Russo O, Ducrot P, et al. Unraveling plant natural chemical diversity for drug discovery purposes. Front Pharmacol. 2020;11:397.
- Rates SM. Plants as source of drugs. Toxicon. 2001;39(5):603–613.
- Veeresham C. Natural products derived from plants as a source of drugs. J Adv Pharm Technol Res. 2012;3(4):200–201.
- Chen L, Dou J, Su Z, et al. Synergistic activity of baicalein with ribavirin against influenza A (H1N1) virus infections in cell culture and in mice. Antiviral Res. 2011;91(3):314–320.
- Ibrahim AK, Youssef AI, Arafa AS, et al. Anti-H5N1 virus flavonoids from Capparis sinaica Veill. Nat Prod Res. 2013;27(22):2149–2153.
- Lee J-H, Oh M, Seok J, et al. Antiviral effects of black raspberry (Rubus coreanus) seed and its gallic acid against influenza virus infection. Viruses. 2016;8(6):157.
- V. K P, Rath SP, Abraham P. Computational designing of a peptide that potentially blocks the entry of SARS-CoV, SARS-CoV-2 and MERS-CoV. PLoS One. 2021;16(5):e0251913.
- Wang Q, Zhang Y, Wu L, et al. Structural and functional basis of SARS-CoV-2 entry by using human ACE2. Cell. 2020;181(4):894–904.e9.
- Farouk A-E, Baig MH, Khan MI, et al. Screening of inhibitors against SARS-CoV-2 spike protein and their capability to block the viral entry mechanism: a viroinformatics study. Saudi J Biol Sci. 2021;28(6):3262–3269.