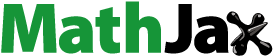
Abstract
This study involves immobilizing α -amylase onto an aniline formaldehyde condensate cross-linked polyaniline magnetic nanocomposite (AFCCLPANIMg). To our knowledge, the utility of AFCCLPANIMg with a core-shell structure in enzyme immobilization has not received sufficient attention. The kinetic parameters were determined under optimal conditions, and the immobilized enzyme had a higher Km value and a lower Vmax value than the free enzyme, owing to the enzyme’s conformational shift. The immobilized enzyme preserved 45% of its original activity after 12 cycles and 59% of its activity in 4-week storage stability testing. The thermal deactivation of the free and immobilized enzyme followed first-order kinetics. The D-values, t1/2 and z value of the immobilized enzyme indicated better thermal stability than that of the free enzyme. The immobilized enzyme’s high Ed value of 19.92 19.92 kJ mol−1 defined its strong stability and resistance to heat inactivation.
Introduction
Biomolecules, especially enzymes, have received great attention from multidisciplinary researchers due to their extraordinary activity and specificity. They are suitable for catalyzing many chemical reactions in complex environments [Citation1]. However, their commercial use is often restricted due to poor operating stability, high consumption, low controllability and non-reusability [Citation2]. The focus of research has been on methods to lower the price of enzymes and make them easier to use in commercial settings. Thus, the enzyme immobilization approach was created, in which the enzymes are linked to a water-insoluble carrier by physical or chemical means [Citation3]. These features increase the immobilized enzyme’s economic viability, decrease process control, and make it appropriate for a range of industrial reactors and processes [Citation4]. Various immobilized enzymes are often used nowadays in the field of clinical diagnostics, pharmaceutics [Citation5], agrochemical products [Citation6], cosmetics [Citation7], textile [Citation8] and in the food industry [Citation9].
Researchers have explored a wide range of carriers to immobilize enzymes [Citation10]. Nanomaterials have become indispensable in all fields of science and technology over the last several decades [Citation10]. A nanocomposite is a matrix to which nanoparticles are added to improve the unique properties of a material [Citation11]. Polymer nanocomposites are gaining popularity due to their improved performance and large surface area. Immobilization leads to automation, long-term economic operations, and recovery of higher-purity end products. Magnetite nanocomposites stand out from the crowd due to their exceptional and unusual magnetic properties [Citation12,Citation13] Magnetite nanocomposites with sizes smaller than 30 nm exhibit superparamagnetic behavior, as seen in materials with a single magnetic domain. These nanocomposites have a wide range of applications, including medication administration, sensors, and contrast agents in magnetic resonance imaging [Citation14].
α-Amylase (1,4-d-glucanglucanhydrolase, EC.3.2.1.1), one of many amylase enzymes, hydrolyzes starch to maltose by randomly cleaving the internal 1,4-linkages (endoamylase). αAmylase is an enzyme that is used in a variety of industrial processes, including the production of ethanol, paper recycling and high fructose corn syrup [Citation15–17]. Amylase can be immobilized using a variety of techniques, including adsorption, covalent bonding and entrapment in the porous matrix, microencapsulation and aggregation. Physical adsorption or ion binding is the most basic and widely used method in the immobilization process. The advantage of this approach is that it reduces interference from the inactive centre, allowing the full level of enzyme activity to be maintained [Citation18,Citation19].
Numerous materials have been explored as an effective support for immobilizing α-amylase. The immobilization of α-amylase was made possible by Khan et al. [Citation20] using the SnO2-NPs structure, which broadened the pH optimum and a higher temperature optimum for the enzyme [Citation20]. Antony et al. [Citation21] reported the immobilization of diastase α-amylase onto Template synthesized polypyrroles [Citation21]. The olibanum-bovine serum albumin@zeolitic imidazolate frameworks nanocomposite was developed by Atirolu et al. as an immobilizing support for the immobilization of α-amylase [Citation22]. Ashly et al. [Citation15] studied the immobilization of α-amylase on PANIs and investigated the kinetic parameters, thermal stability, reusability and storage stability of the enzyme under free and immobilized conditions [Citation15]. Statistical optimization of alkaline α-amylase immobilization onto nano-sized supermagnetic ironoxide nanoparticles (MNPs) was done for enhancing the cost-effective industrial use of MNP-bound α-amylase [Citation23]. The pH and temperature stability of α-amylase have been investigated by adsorption on magnetic particles coated with polyaniline [Citation24]. Many recent studies have concentrated on the fabrication of a wide range of composites with magnetic and conducting properties. Belaabed et al. [Citation25] developed hybrid organic/inorganic electromagnetic absorbent materials (EMAMs) for electromagnetic applications by dispersing magnetite (Fe3O4) and polyaniline (PANI) fillers in epoxy resin [Citation25]. Coating the Fe3O4 particles with PANI may result in a new nanocomposite with a core-shell structure that has electrical and magnetic properties. Wan’s group created PANI with ferromagnetic properties in two ways [Citation26,Citation27]. When these nanocomposites with core-shell structures were immersed in 3 mol L−1 H2SO4 aqueous solution, the magnetite core was destroyed, making acidic doping of these supports impossible. So they produced an aniline formaldehyde condensate crosslinked polyaniline magnetite composite (AFCCLPANIMg) by using aniline formaldehyde condensate during aniline polymerization in the presence of nanomagnetite dispersed in aniline monomer solution, wherein cross-linked PANI serves as the conductive shell and Fe3O4 serves as the magnetic core. It is suitable for usage in strong acid aqueous solutions.
Although a number of studies have been reported on aniline formaldehyde cross linked polyaniline- magnetite composite for different applications, nonetheless, their utility in immobilization studies has not been explored widely to date [Citation28,Citation29]. In the present study, AFCCLPANIMg composite with a core-shell structure was used as a support for immobilizing α-amylase. The aim of our study was to investigate the thermal stability, thermodynamic characteristics and thermal deactivation kinetics, reusability and storage stability of immobilized enzyme – the parameters essential for the enzyme to function as an efficient industrial catalyst.
Materials and methods
Materials
Diastase α-amylase (1,4 α-D-gluconglucanohydrolase, E. C. 3.2.1.1) was bought from Hi-Media Laboratories Pvt. LTD., Mumbai. Soluble potato starch was obtained from S.D. Fine Chem. Ltd., Mumbai. The other additional reagents utilized in the present investigation were all of analytical quality and were employed without any prior treatment. Aniline formaldehyde condensate cross linked polyaniline magnetite (AFCCLPANIMg) nanocomposite was synthesized following a standard procedure [Citation30] with slight modifications by in-situ polymerization of acidic solution containing magnetite, in the presence of ammonium peroxydisulfate as an oxidative polymerizing agent [Citation31], and was previously characterized to serve as the support for immobilizing diastase α-amylase [Citation31].
Immobilization of α-amylase
The enzyme α-amylase was immobilized onto the nanocomposite AFCCLPANIMg by mixing nanocomposite with equal amounts of enzyme solution and buffer under optimal immobilization conditions [Citation32]. For this, 0.05 g of support was mixed with 1 mL of 0.2 mol L−1 sodium phosphate buffer of pH 7 for five minutes. Then the free amylase was dissolved in distilled water to make a solution of concentration 1 mg mL−1 and it was added to the support-buffer mixture in sufficient volume in a ratio of 1 mg of enzyme per 1 gram of support. The mixture was kept in a shaking incubator for 1 h at 40 °C. The immobilized enzyme was filtered, washed with the phosphate buffer of pH 7, air dried at room temperature for 24 h and stored at 4 °C in a refrigerator for further studies.
Activity determination of the free and immobilized enzyme
Using 1% starch as a substrate, Fuwa’s method was used to determine the activity of the immobilized and free enzymes [Citation33]. In this hydrolytic assay study, we investigated the hydrolysis of starch substrate. The residual starch was determined using an iodine-potassium iodide reagent. In a standard assay, the mixture consisted of 1 mL of free α-amylase solution (or an analogous equivalent quantity of immobilized biocatalysts) in 1 mL of the buffer of the desired pH (0.2 mol L−1 sodium acetate buffer for pH values 4.0–5.5, 0.2 mol L−1 sodium phosphate buffer for pH 6.0–8.0 and 0.2 mol L−1 glycine buffer for pH 8.5 − 9.0). The assay reaction was started by the addition of 1 mL of 1% starch solution as a substrate and was shaken thoroughly in a shaking incubator at 40 °C for 20 min. Afterward, 1 mL of the above solution was withdrawn and added to a test-tube containing 0.3 mL HCl and to stop the reaction. Finally, 0.1 mL iodine-potassium iodide reagent was added, which gives a violet color to the unreacted starch, and was diluted with 30 mL distilled water. The absorbance of this pale violet colored solution was instantly measured at 650 nm using a Thermo scientific Evolution™ 201 UV-Visible Double Beam Spectrophotometer. The enzyme activity unit (U) is expressed in terms of amount of enzyme catalyzing the transformation of 1 μmol of starch into product in 1 min at the given temperature and under the assay conditions.
Consequently, EquationEquation (1)(1)
(1) was used to compute the activity (U mL−1).
(1)
(1)
where A650 sample is the absorbance for the starch digested with the enzyme, as opposed to A650 control, which is the absorbance obtained from the starch without the addition of an enzyme.
The relative activity (%) was expressed as the ratio between the activity of every sample and the maximum activity of sample.
Determination of protein content
The Folin and Lowry technique was used to assess the total protein content for the assay of free amylase and immobilized biocatalysts [Citation34]. The protein content employed for immobilization was first determined. Following immobilization, the enzyme was thoroughly filtered and washed with buffer. The filtrate and washings were collected to determine the amount of unbound enzyme using Folin Ciocaltaue’s reagent by measuring the absorbance at 660 nm in a Thermo Scientific Evolution™ 201 UV-Visible Double Beam Spectrophotometer. Thus, the amount of protein immobilized on AFCCLPANIMg composites is the difference between the original amount of proteins used to load and the amount of protein that remained unbound.
Determination of immobilization yield, activity yield and immobilization efficiency
The immobilized enzyme performance can be expressed as the immobilization yield, activity yield and immobilization efficiency (IE) percentage. Immobilization yield percentage (IY%) was calculated using EquationEquation (2)(2)
(2) by comparing the protein content of the supernatant before and after immobilization [Citation15]:
(2)
(2)
Where C1 is the protein concentration used for immobilization and C2 was the protein concentration in the supernatant following immobilization.
The activity yield percentage was determined by measuring the activity of the immobilized enzyme and the activity of the initial enzyme used in the immobilization reaction as in EquationEquation (3)(3)
(3) [Citation15]:
(3)
(3)
The immobilization efficiency (IE) was calculated using EquationEquation (4)(4)
(4) :
(4)
(4)
Effect of pH and temperature on enzyme activity
The reaction pH has an influence on the activity of the free enzyme and immobilized enzyme and its variation was studied for a pH range of 4–9 at room temperature using 0.2 mol L−1 sodium acetate buffer for pH values 4.0 − 5.5, 0.2 mol L−1 sodium phosphate buffer for pH 6.0–8.0 and 0.2 mol L−1 glycine buffer for pH 8.5 − 9.0.
By adjusting the temperature between 30 °C and 70 °C, the best temperature for the maximum activity of the free and immobilized enzyme was investigated at pH 7. The log of the relative activity of the measured temperature was plotted against 1/T (Kelvin) for the free and immobilized samples, and the activation energy Ea was calculated from the slope of the Arrhenius plot in accordance with EquationEquation (5)(5)
(5) , where R is the gas constant (8.314 kJ/mol) and T is the absolute temperature (Kelvin).
(5)
(5)
The temperature coefficient value (Q10) was derived using EquationEquation (6)(6)
(6) , where Ea is the enzyme’s activation energy (J/mol) and Q10 is the rate of an enzymatic catalysis process changing for every 10 °C rise in temperature [Citation35].
(6)
(6)
Kinetic studies of free and immobilized α-amylase
Enzymatic tests were performed with substrate concentrations ranging from 0.2 to 1.0 mg mL-1 at optimal temperature and pH to evaluate the kinetic parameters, Michaelis constant (Km), and maximum rate (Vmax) for free and immobilized enzyme. The Lineweaver - Burk plot was used to compute the Michaelis constant (Km) and the maximum rate (Vmax) using EquationEquations (7)(7)
(7) and Equation(8)
(8)
(8) , respectively:
(7)
(7)
(8)
(8)
The turnover number and specificity constant of free and immobilized enzyme was calculated using EquationEquations (9)(9)
(9) and Equation(10)
(10)
(10) [Citation36]:
(9)
(9)
(10)
(10)
Determination of thermal stability parameters
The enzyme samples were subjected to different temperatures (30–70 °C) at optimum pH for a pre-incubation period of 1 h in the absence of substrate, and the residual activity was then measured with starch as the substrate. This illustrated the heat resistance of the enzyme.
The stability of free and immobilized enzymes was also studied by pre-incubating the enzyme at optimum temperature for various time intervals (0–120 min) in the absence of substrate and then assessing the enzyme activity using starch as the substrate.
The enzyme stability profile, which is described by thermal deactivation research, shows how residual activity varies over time during the pre-incubation phase and is depicted in terms of initial enzyme activity. Amylase was incubated at temperatures of 50, 60 and 70 °C for intervals of time (30, 60, 90 and 120 min) to investigate the degree of thermal inactivation of free and AFCCLPANIMg immobilized amylase [Citation37]. The samples were then cooled to the optimal temperature, and the residual enzyme activity was evaluated with starch substrate using the methods described by Ahmed et al. [Citation38] and Abdel-Naby et al. [Citation39].
The thermal deactivation parameters and thermodynamic constants were computed using the thermal stability profile data. The thermal deactivation rate constant for the deactivation process of the enzyme was obtained from the slope of the regression graph plotted between log relative activity % against preincubating time at the temperatures used for inactivation as given by EquationEquation (11)(11)
(11)
(11)
(11)
The apparent half-lives of immobilized enzymes, t1/2, defined as the time required to achieve 50% residual activity, were calculated using EquationEquation (12)(12)
(12) :
(12)
(12)
The preincubation periods necessary for the enzyme to preserve 10% of its residual activity, as calculated by EquationEquation (13)(13)
(13) , are known as D-values, or decimal reduction time:
(13)
(13)
The temperature sensitivity parameter z-value, which indicates the temperature needed to drop the D-value by one logarithmic cycle, may be calculated using the slope of the plot produced between log D versus temperature (◦C) in accordance with EquationEquation (14)(14)
(14) .
(14)
(14)
The Arrhenius plot was used to describe the temperature dependence of kd, and the deactivation energy (Ed) was calculated using the Arrhenius EquationEquation (15)(15)
(15) :
(15)
(15)
Where the universal gas constant, R, is equal to 8.314 J mol−1 K−1, and is the Arrhenius constant.
By analyzing the slope of the Arrhenius plot between ln kd and the reciprocal of temperature, 1/T (K), EquationEquation (16)(16)
(16) was used to determine the activation energy for deactivation (Ed).
(16)
(16)
Estimation of thermodynamic parameters
EquationEquations (17–19) use the values of the deactivation rate constant, kd, and deactivation energy, Ed, to determine the thermodynamic parameters such as the free energy (ΔG0, kJ mol−1), change in enthalpy (ΔH0, kJ mol−1) and entropy (ΔS0, J mol−1 K−1) for thermal deactivation of the enzyme:
(17)
(17)
(18)
(18)
(19)
(19)
Where T is the absolute temperature (K), R is the gas constant (8.314 J mol− 1 K− 1) and h is the Planck constant (11.04 × 10−36 J min).
Reusability and storage stability
The reusability of the immobilized enzyme was tested using a batch experiment with fixed times for each cycle. At the optimal pH and temperature, the immobilized enzyme’s residual activity was assessed at regular intervals. At the end of each cycle, the immobilized enzyme was removed, washed with buffer, and a substrate solution was added to start a new cycle. The process is repeated after each count. After many months of storage at 4 °C, the activity of immobilized enzymes in buffer solution was assessed. Regular intervals were used to repeat the test. The % activity was obtained by comparing the final activity to the initial one.
Results
Optimization of immobilization of free enzyme
The α-amylase was immobilized onto the AFCCLPANIMg nanocomposite by maintaining immobilization parameters at optimum conditions: immobilization pH = 7, immobilization temperature = 40 °C, contact time between the support and enzyme = 1 h, initial protein amount =10.1 mg. The immobilization yield, specific activity yield, and immobilization efficiency of the immobilized enzyme were evaluated [Citation40,Citation41] ().
Table 1. Immobilization yield, activity yield, and immobilization efficiency of immobilized enzyme.
Effect of pH and temperature on enzyme activity
The effect of pH on the activity of α-amylase was tested in the pH range of 4 to 9 at 40 °C (). The free enzyme showed its highest level of activity at pH of 5.5, whereas the immobilized enzyme showed an alkaline shift of 0.5 unit and exhibited maximum activity at pH 6. Also the curve obtained for immobilized enzyme was broader than the free enzyme, which is in agreement with the previous reported works that immobilization enhances the stability and preserves activity of enzyme across a wider pH range [Citation24,Citation41].
Figure 1. Effect of reaction pH on relative activity (%) of free α-amylase (FE) and α-amylase immobilized on AFCCLPANIMg composite (IE). Reaction conditions: Temperature 40 °C, starch concentration 1% w/v, reaction time 20 min.
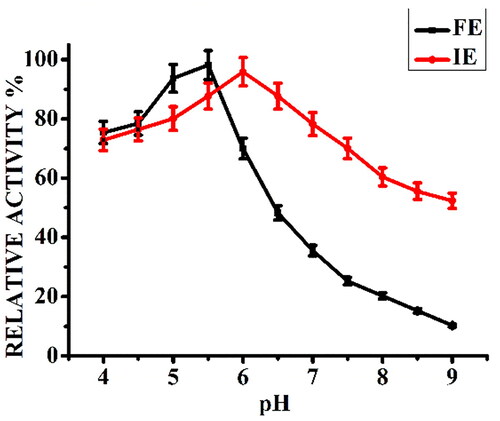
To determine the optimal temperature of the enzyme, the enzyme-starch mixture in phosphate buffer (pH 7) was incubated at reaction temperatures ranging from 30 to 60 °C and the enzyme activity was measured. The temperature dependence curve of free enzyme and immobilized enzyme () showed that the relative activity of 86% exhibited by immobilized enzyme was comparable with that of the free enzyme, but shifted its optimal temperature from 50 °C to 40 °C.
Figure 2. Effect of reaction temperature on relative activity (%) of free α-amylase (FE) and α-amylase immobilized on AFCCLPANIMg composite (IE). Reaction conditions: optimum pH, starch concentration 1% w/v, reaction time 20 min.
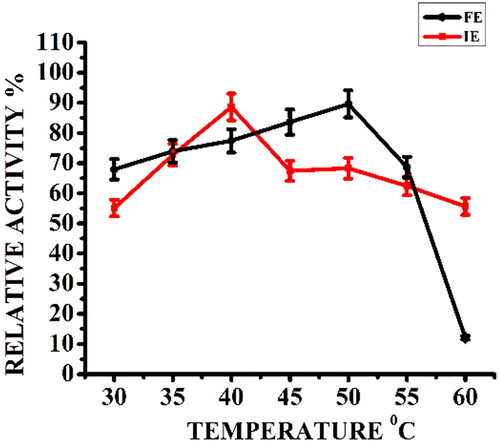
The Arrhenius equation of an enzyme catalysed reaction below its inactivation temperature expresses the temperature dependence of the rate constant of that reaction. The corresponding Arrhenius plot of ln k against 1/T is used to compute the activation energy (Ea) as denoted by EquationEquation (5)(5)
(5) . Thus the activation energy of immobilized amylase and free amylase for the hydrolysis of the starch substrate can be obtained from the Arrhenius plot for free enzyme and immobilized enzyme shown in . The free enzyme’s activation energy (Ea = 11.05 kJ mol−1) was lower than that of the immobilized enzyme (Ea = 36.41 kJ mol−1).
Figure 3. Arrhenius plot for free α-amylase (FE) and α-amylase immobilized on AFCCLPANIMg composite (IE). Reaction conditions: optimum pH, starch concentration 1% w/v, reaction time 20 min.
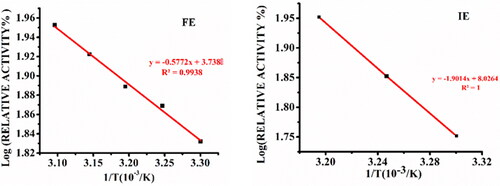
The temperature quotient (Q10), which is the proportion by which the rate increases when the temperature raises by 10 °C, was used to express how temperature affects the reaction rate. Utilizing the Dixon and Webb equation, Q10 was determined [Citation42]. Enzymatic reactions employing both free and immobilized enzymes produced Q10 values between 1 and 2 ().
Table 2. Q10 of free enzyme and immobilized enzyme.
Kinetic parameters
By performing starch hydrolysis at optimum temperature and pH for substrate concentrations ranging from 0.2 to 1.00 mg/mL, the kinetic characteristics of free and immobilized enzymes were examined. The Michaelis-Menten constant (Km) value represents the enzyme’s affinity for the substrate, the maximum rate of the enzymatic reaction (Vmax). The kinetic parameters, Km, Vmax are obtained from the Lineweaver-Burk plot shown in . The turnover number (Kcat) value represents the number of molecules of substrate transformed into product per minute per enzyme molecule. The Km, Vmax, turnover number, Kcat and catalytic efficiency Kcat/Km of free and immobilized amylase were calculated ().
Figure 4. Lineweaver Burk plot for free α-amylase (FE) and α-amylase immobilized on AFCCLPANIMg composite (IE). S0 is the initial substrate concentration and Vo is the reaction rate. Reaction conditions: optimum pH, temperature 40 °C, reaction time 20 min.
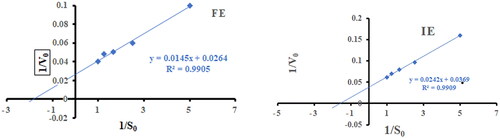
Table 3. Comparison of kinetic parameters of free and immobilized enzyme.
Thermal stability assay
The economic worth and industrial applicability of enzymes are mostly determined by enzyme stability. To study the thermal stability of the free and immobilized forms in this work, a range of preincubation temperatures and time intervals were applied. The results indicated that the immobilization step contributed to enhancing the amylase thermal stability ().
Figure 5. Thermal stability of free α-amylase (FE) and α-amylase immobilized on AFCCLPANIMg composite (IE). The enzymes were incubated at various temperatures from 30 to 70 °C for 1 h in the absence of substrate (a). The enzymes were incubated at optimum temperature for various time from 0-120 min in the absence of substrate (b). In both cases, Reaction conditions: optimum pH, temperature 40 °C, time 20 min, starch concentration 1% w/v.
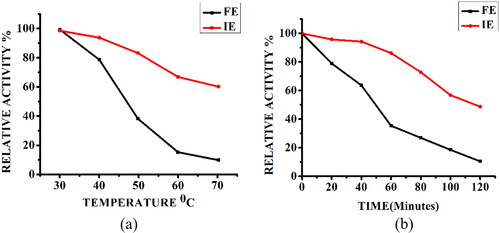
Thermal deactivation of immobilized and free enzyme
The occurrence of thermal deactivation processes at high temperature is a serious issue with the use of enzymes as industrial catalysts. The process of heat deactivation causes conformational changes in the protein structure, which may have an unfavorable effect on their specific activity. It is crucial to understand the deactivation kinetics and thermodynamic characteristics of industrial enzymes.
The plot of log relative activity % vs. time () was fitted to a straight line with good R2 values, indicating that first-order kinetics governs the thermal deactivation of free and immobilized enzymes at different pre-incubation time intervals [Citation37,Citation43]. The stability of enzymes is primarily described by kinetic and thermodynamic stability [Citation44]. The latter evaluates the changes in Gibbs free energy (ΔGo), change in enthalpy (ΔHo) and change in entropy (ΔSo) between the enzyme and deactivated versions of the enzyme. The former () affects the resistance to thermal deactivation and is reported as the enzyme half-life (t1/2), decimal reduction time (D) (), thermal deactivation rate constant (kd), temperature sensitivity parameter (z). Analyzing these thermodynamic variables () helps to explain how the enzyme reacts with a certain substrate molecule. The activation energy for thermal inactivation (Ed) of free and immobilized enzymes was calculated using the slope of the Arrhenius plot of thermal deactivation () and presented in .
Figure 6. Thermal stability of free α-amylase (FE) and α-amylase immobilized on AFCCLPANIMg composite (IE) by incubating the enzyme at 50 °C, 60°Cand 70 °C for various preincubation time upto120 minutes in the absence of substrate. In both cases, Reaction conditions for activity determination: optimum pH, temperature 40 °C, time 20 min, starch concentration 1% w/v.
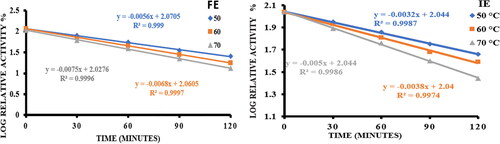
Figure 7. Temperature dependence of the decimal reduction of free α-amylase (FE) and α-amylase immobilized on AFCCLPANIMg composite (IE). Reaction conditions: optimum pH, starch concentration 1% w/v, reaction time 20 min.
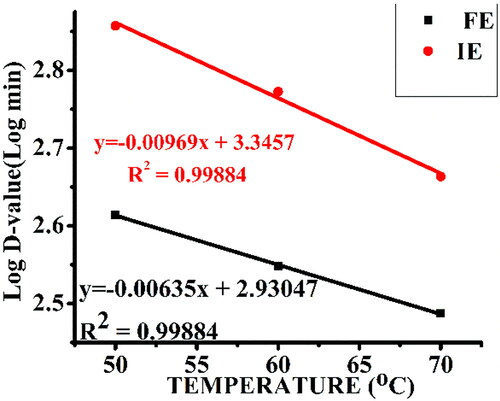
Figure 8. Arrhenius plot of thermal deactivation of free α-amylase (FE) and α-amylase immobilized on AFCCLPANIMg composite (IE). Reaction conditions: optimum pH, starch concentration 1% w/v, reaction time 20 min.
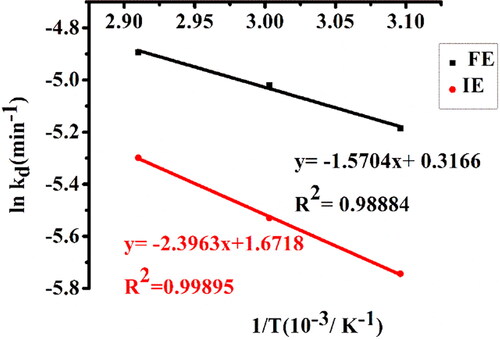
Table 4. Kinetic parameters for thermal deactivation of free enzyme and enzyme immobilized on AFCCLPANIMg composite.
Table 5. Thermodynamic parameters for thermal deactivation of free enzyme and enzyme immobilized on AFCCLPANIMg composite.
Reusability and storage stability
Enzyme storage stability and reusability are crucial industrial criteria for their practical usage [Citation24]. The reusability of immobilized α-amylase over 12 reaction cycles at optimum pH and temperature in our study is shown in [Citation15].
Figure 9. Reusability (a) and storage stability (b) of immobilized inzyme. (a) Reusability of immobilized enzyme for 12 reaction cycles; Reaction conditions: optimum pH, temperature 40 °C, starch concentration 1% w/v, reaction time 20 min; the relative activity of the first run was taken as 100%. (b) Storage stability of immobilized enzyme (stored at 4 °C) for four weeks; Reaction conditions: optimum pH, temperature 40 °C, starch concentration 1% w/v, reaction time 20 min; the relative activity of the initial week was taken as 100%.
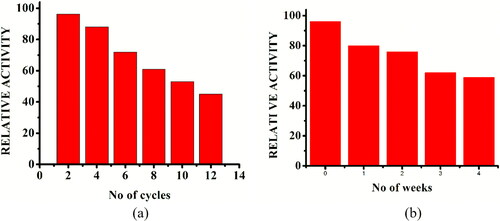
To assay the storage stability, the immobilized and free enzymes were kept in buffer at 4 °C. The free enzyme completely lost its activity within 2 days, whereas the immobilized enzymes preserved higher activity. The catalytic activity of immobilized enzyme was assessed at 7-day intervals [Citation10]. It retained 76% of its activity after 2 weeks and 59% after 4 weeks (), suggesting that the immobilized enzyme showed improved storage stability. This trend indicated that immobilization of α-amylase onto AFCCLPANIMg composite succeeded to reduce the natural loss of enzyme activity and to increase the enzyme’s storage stability [Citation10].
Discussion
The enzyme is immobilized onto AFCCLPANIMg by maintaining the optimum immobilizing conditions and evaluated the immobilization yield, specific activity yield, and immobilization efficiency. The immobilization yield is found to be 91.09%, Specific activity yield 83.09% and immobilization efficiency 91.21%.The amount of physically adsorbed α-amylase was 9.2 mg g−1 of AFCCLPANIMg. The binding capacity of the polymeric matrix is an essential element for effective immobilization. In some α-amylase immobilization studies, the binding ability of the matrix is fluctuating due to the produced materials’ unique features. Using prepared metal oxide nano composites, ZnO-Fe3O4 and ZnO-PANI, the amount of immobilized α-amylase was 0.38 mg g−1 and 0.41 mg g−1 support, respectively [Citation45]. The immobilized enzyme demonstrated an alkaline shift in the area of optimal pH. This may be related to the positive charges on the composite’s amine group, which may have led to a drop in the H+ concentration in the microenvironment around the immobilized enzyme. The immobilized enzyme’s structural configuration may alter as a result, increasing the enzyme’s activity at higher pH levels. One of the reasons for the greater pH stability displayed by immobilized enzyme could be the multipoint attachment of the enzyme to the surface of the support employed for immobilization, which allows the enzyme to resist the severe structural changes with change in pH [Citation41].
It is possible that a change in the structural integrity of the enzyme structure, which promoted improved amylase activity below 50 °C, is what caused the decrease in the optimal temperature by immobilization. Alternatively, the protein structure may have been altered by the attachment to the support, and higher temperatures may have resulted in the denaturation of the enzyme due to the breakdown of non-covalent intramolecular interactions [Citation10]. The immobilized enzyme exhibited a broad and stable temperature profile and retained more than 50% activity even at 60 °C. This can be ascribed to the protective effect provided to the enzyme by the support at higher temperatures where denaturation occurs [Citation46].
The higher activation energy of immobilized amylase (Ea = 36.41 kJ mol−1), which is almost 70% more than that of free amylase (Ea = 11.05 kJ mol−1), may be attributed to the prolongation of enzyme and substrate contact and to the changes in the structural conformation of the enzyme by immobilization, which in turn decreases the catalytic efficiency [Citation47]. This is in agreement with the previously reported work [Citation48].
The impact of a 10 °C increase in temperature on the rate of a chemical reaction is known as the temperature coefficient (Q10). In our study, the Q10 value of both free enzyme and immobilized enzyme at 30 °C and 40 °C was around 1.1 and 1.5, respectively, indicating that temperature changes have no influence on the tertiary structure of the enzyme up to the optimal temperature [Citation49].
Immobilization of enzymes may have an impact on the microenvironment as well as conformation. The first refers to the structural alterations that the enzyme molecule undergoes and the steric effects brought on by its near contact to the carrier’s surface, while the second refers to mass transfer restrictions. Evaluation of enzyme kinetic parameters will help us to understand the rate of mass transport of substrates into the biocatalyst from the bulk reaction media and the rate of mass transport of products out of the biocatalyst back into the bulk reaction medium. In our study, we have estimated the kinetic constants using Lineweaver-Burk plot and From the result, it is clear that immobilization has led to a decrease in Vmax and an increase in Km. This is an indication of lower affinity of enzyme for substrate molecules due to conformational changes in the enzyme caused by excessive adsorption of enzymes on the support, resulting in reduced accessibility of substrate molecules to the enzyme’s active site [Citation35,Citation50].
The lower turnover number, Kcat of immobilized enzyme was 28% lower than that of the free enzyme, indicating that immobilized amylase requires more time than free enzyme to convert one substrate molecule into products [Citation51]. The lower value of Kcat/Km for immobilized enzyme denotes the lower catalytic efficiency of immobilized enzyme [Citation52].
In the thermal stability assay, the immobilized enzyme was found to be more stable than the free enzyme. The higher activity of the immobilized enzyme at high preincubation temperature and preincubation time is due to the high thermal stability which is attained by the conformational integrity of the enzyme structure by immobilization with rigid support [Citation42].
Both free and immobilized enzymes were subjected to thermal deactivation studies with respect to various pre-incubation times and pre-incubation temperatures. A lower value of the kinetic constant of enzyme deactivation (kd) for the immobilized enzyme indicates that the immobilization procedure increased the thermal stability of α-amylase [Citation53]. The kd of both free and immobilized amylase was found to increase with temperature, which was in agreement with the fact that the stability of enzyme decreased with temperature [Citation54]. From the kd value, the speed of the thermal deactivation was found to increase by 99% for free enzyme and only by 18% for immobilized enzyme upon increasing the temperature of deactivation from 50 °C to 60 °C. This is because the denaturation of protein is more pronounced for a free enzyme with an increase in temperature.
For both free and immobilized enzymes, t1/2 decreased with temperature, confirming the lower stability of the enzyme at a higher temperature [Citation53]. The values of t1/2 and D were 75%, 79% and 50% significantly higher for the immobilized enzyme at 50 °C, 60 °C and 70 °C, respectively, indicating its better thermal stability than the free enzyme.
The z-values (based on the slope of the temperature dependence plot of the decimal reduction of free enzyme and immobilized enzyme) were 157.48 for the free enzyme and 103.20 for the immobilized enzyme (). Large z-values of the free enzyme indicate that the free enzyme is more sensitive to the duration of heat treatment than the increase in temperature. The small z-values of the immobilized enzyme are due to its greater sensitivity to an increase in temperature rather than its duration as a result of the immobilization process [Citation32].
The activation energy for thermal inactivation (Ed) of free and immobilized enzymes was calculated using the slope of the Arrhenius plot of thermal deactivation () and presented in . According to the findings, the Ed of immobilized enzymes was 52% greater than that of free enzymes, demonstrating strong stability and resistance to heat inactivation of the enzyme by the method of immobilization. A higher Ed value indicates that more energy is required to denature the enzyme.
The ΔH° values of both immobilized and free enzymes decreased with an increase in temperature, showing that less energy is required to denature the enzyme, which leads to considerable conformational changes at high temperatures. Also, the higher ΔH° values of the immobilized enzyme compared to the free enzyme at the same temperature range indicate the high stability of immobilized to free enzyme [Citation54,Citation55].
The Gibbs free energy change (ΔG°) provides information on the enzyme’s stability as well as the spontaneity of the enzymatic process catalyzed under different temperatures. The positive ΔG° values for thermal deactivation in the present investigation () indicate that the processes were not spontaneous, but endergonic. An increase in the ΔG° value with increasing temperature is observed for both free and immobilized enzyme, but it is greater for immobilized enzymes [Citation56]. Since positive ΔG° denotes resistance toward thermal unfolding, a higher value for immobilized enzymes, 1.0-fold higher than that of free enzyme, designates it as more stable than free enzyme [Citation57]. Protein stability is closely connected to ΔG° values, with high and positive ΔG° values indicating the non-spontaneity of the enzyme’s heat denaturation reaction (higher thermal stability) [Citation58].
Thermal deactivation of the free enzyme and immobilized enzyme resulted in negative entropy in the present study, indicating that the randomness or disorder during the transition from the native state to the denatured state of the free and immobilized enzyme decreases [Citation54,Citation58], indicating enzyme aggregation. The less negative values of entropy for immobilized enzyme agree with less aggregation of the enzyme due to the more ordered structure as a result of immobilization [Citation59].
The reusability and storage stability study of immobilized enzyme revealed its operational stability. In a robust nanobiocatalyst, immobilized enzyme retained 72% of its activity after 6 cycles and 45% after 12 cycles [Citation10], showing that the immobilized enzyme had durable catalytic activity under repeated use. The immobilized α-amylase onto AFCCLPANIMg composite can be readily removed from the reaction mixture using an external magnetic field and consequently can be reused for the hydrolysis of starch [Citation10]. The stability profile of the immobilized enzyme in this study revealed that the operational stability is comparable to that of the previously reported work in which α-amylase immobilized onto PANI coated magnetic particles exhibited 55% after 9 cycles [Citation24]. The operation stability of immobilized amylase in the study was higher than in the previously reported work in which α-amylase immobilized on PANI powder by physical adsorption exhibited just 20% activity after 10 cycles whereas lower than the α-amylase covalently immobilized on PANI powder [Citation15].
Immobilized enzyme retained 76% of its activity after 2 weeks and 59% after 4 weeks, suggesting that the immobilized enzyme exhibits improved storage stability. This could be because diastase-amylase, a multimeric metalloenzyme, has enhanced its stability. Immobilization can increase the enzyme structural rigidity by stabilization against denaturation and/or subunit dissociation, thus reducing the inactivation caused by conformational changes and chemical inactivation during storage [Citation60]. Immobilization can also help to recover the immobilized biocatalyst from the reaction medium more effectively because of its magnetic property [Citation24]. Inactivation of the enzyme owing to decreasing binding strength between the enzyme and the support leading to leaching of enzymes from the support after continuous use, enzyme denaturation and product inhibition may be the reason behind the loss in activity of immobilized amylase [Citation18,Citation24].
Conclusions
α-Amylase was successfully immobilized onto AFCCLPANIMg nanocomposite. The optimum values of pH and temperature of α-amylase in magnetite nanocomposite were shifted most likely due to conformational changes of the enzyme, and this could increase the applicability of the enzyme. The Vmax/Km values showed that the substrate affinity of immobilized amylase was lower than that of free amylase. The immobilized enzyme showed promising storage stability and reusability. These findings show that AFCCLPANIMg was an effective support for α-amylase immobilization. The kinetics and thermodynamic parameters of thermal deactivation of enzyme immobilized onto AFCCLPANIMg nanocomposite were found. The study revealed the thermal stability of the immobilized enzyme over the free enzyme and that the AFCCLPANIMg nanocomposite immobilized enzyme is tailored to the requirements of an industrial catalyst.
Author contributions
MA: performed the experiments, curated data, wrote the original draft, edited and revised the manuscript;
DTM: edited and revised the manuscript; PVM: supervised the work, editing and revision.
Supplemental Material
Download MS Word (105.8 KB)Acknowledgments
The authors are thankful to Cochin University of Science and Technology for providing facilities for this work.
Disclosure statement
The authors declare that they have no known competing financial interests or personal relationships that could have appeared to influence the work reported in this paper.
Additional information
Funding
References
- Wu S, Snajdrova R, Moore JC, Baldenius K, & Bornscheuer UT. Biocatalysis: enzymatic synthesis for industrial applications. Angew Chem Int Ed. 2021 60:88–119.
- Eş I, Vieira JDG, Amaral AC. Principles, techniques, and applications of biocatalyst immobilization for industrial application. Appl Microbiol Biotechnol. 2015;99:2065–2082.
- Sheldon RA, Basso A, Brady D. New frontiers in enzyme immobilisation: robust biocatalysts for a circular bio-based economy. Chem Soc Rev. 2021;50:5850–5862.
- Sanchez S, Demain AL. Enzymes and bioconversions of industrial, pharmaceutical, and biotechnological significance. Org Process Res Dev. 2011;15:224–230.
- Meghwanshi GK, Kaur N, Verma S, et al. Enzymes for pharmaceutical and therapeutic applications. Biotechnol Appl Biochem. 2020;67:586–601.
- Shang X-F, Liu YQ, Guo X, et al. Application of sustainable natural resources in agriculture: acaricidal and enzyme inhibitory activities of naphthoquinones and their analogs against Psoroptes cuniculi. Sci Rep. 2018;8:1–9.
- Heath RS, Ruscoe RE, Turner NJ. The beauty of biocatalysis: sustainable synthesis of ingredients in cosmetics. Nat Prod Rep. 2022;39:335–388.
- Khan WS, Hamadneh NN, Khan WA. Polymer nanocomposites–synthesis techniques, classification and properties. In: Di Sia P, editor. Science and applications of tailored nanostructures. Manchester (UK): One Central Press (OCP); 2016. p. 50.
- Mohammadi A, Jafari SM, Mahoonak AS, et al. Liposomal/nanoliposomal encapsulation of food-relevant enzymes and their application in the food industry. Food Bioprocess Technol. 2021;14:23–38.
- Defaei M, Taheri-Kafrani A, Miroliaei M, et al. Improvement of stability and reusability of α-amylase immobilized on naringin functionalized magnetic nanoparticles: a robust nanobiocatalyst. Int J Biol Macromol. 2018;113:354–360.
- Gubin SP. Magnetic nanoparticles. Weinheim: John Wiley & Sons; 2009.
- Liu J, Qiao SZ, Hu QH, et al. Magnetic nanocomposites with mesoporous structures: synthesis and applications. Small. 2011;7:425–443.
- Burke NAD, Stöver HDH, Dawson FP. Magnetic nanocomposites: preparation and characterization of polymer-coated iron nanoparticles. Chem Mater. 2002;14:4752–4761.
- Mushtaq A, Zhao R, Luo D, et al. Magnetic hydroxyapatite nanocomposites: the advances from synthesis to biomedical applications. Mater Des. 2021;197:109269.
- Ashly PC, Joseph MJ, Mohanan PV. Activity of diastase α-amylase immobilized on polyanilines (PANIs). Food Chem. 2011;127:1808–1813.
- Mardani T, Khiabani MS, Mokarram RR, et al. Immobilization of α-amylase on chitosan-montmorillonite nanocomposite beads. Int J Biol Macromol. 2018; 120(Pt A):354–360.
- Raul D, Biswas T, Mukhopadhyay S, et al. Production and partial purification of alpha amylase from Bacillus subtilis (mtcc 121) using solid state fermentation. Biochem Res Int 2014;2014:1–5.
- Sanjay G, Sugunan S. Acid activated montmorillonite: an efficient immobilization support for improving reusability, storage stability and operational stability of enzymes. J Porous Mater. 2008;15:359–367.
- Sanjay G, Sugunan S. Glucoamylase immobilized on montmorillonite: influence of nature of binding on surface properties of clay-support and activity of enzyme. J Porous Mater. 2007;14:127–136.
- Khan MJ, Husain Q, Ansari SA. Polyaniline-assisted silver nanoparticles: a novel support for the immobilization of α-amylase. Appl Microbiol Biotechnol. 2013;97:1513–1522.
- Antony N, Mohanan PV. Template synthesized polypyrroles as a carrier for diastase alpha amylase immobilization. Biocatal. Agric. Biotechnol. 2019;19:101164.
- Atiroğlu V, Atiroğlu A, Özacar M. Immobilization of α-amylase enzyme on a protein @metal–organic framework nanocomposite: a new strategy to develop the reusability and stability of the enzyme. Food Chem. 2021;349:129127.
- Mukherjee AK, Kumar TS, Rai SK, et al. Statistical optimization of Bacillus alcalophilus α-amylase immobilization on iron-oxide magnetic nanoparticles. Biotechnol Bioproc E. 2010;15:984–992.
- Radovanović M, Jugović B, Gvozdenović M, et al. Immobilization of α-amylase via adsorption on magnetic particles coated with polyaniline. Starch/Staerke. 2016;68:427–435.
- Belaabed B, Wojkiewicz JL, Lamouri S, et al. Synthesis and characterization of hybrid conducting composites based on polyaniline/magnetite fillers with improved microwave absorption properties. J. Alloys Compd. 2012;527:137–144.
- Wan M, Fan J. Synthesis and ferromagnetic properties of composites of a water‐soluble polyaniline copolymer containing iron oxide. J Polym Sci A Polym Chem. 1998;36:2749–2755.
- Wan M, Li J. Synthesis and electrical–magnetic properties of polyaniline composites. J Polym Sci A Polym Chem. 1998;36:2799–2805.
- Rana S, Jadhav NV, Barick KC, et al. Polyaniline shell cross-linked Fe3O4 magnetic nanoparticles for heat activated killing of cancer cell. Dalton Trans. 2014;43:12263–12271.
- Panahi A, Zadeh S. Nickel adsorption from environmental samples by ion imprinted aniline -formaldehyde polymer. Iran J Chem Chem Eng. 31:35–44. 2012;
- Deng J, He CLan, Peng Y, et al. Magnetic and conductive Fe3O4–polyaniline nanoparticles with core–shell structure. Synth Met. 2003;139:295–301.
- Augustine MGPV, Mohanan G. Aniline formaldehyde cross linked polyaniline magnetic nanocomposite cross shell strucure: synthesis and characterisation. In Proceedings on National Seminar on Sustainable Innovations in Functional Materials: Developments and Applications, Department of Physics, Chemistry and Biotechnology, Al Ameen College in collaboration with Indian Science Congress Association, Cochin Chapter; 20th and 21st July 2022. p. 45–51..
- Bindu VU, Mohanan PV. Thermal deactivation of α-amylase immobilized magnetic chitosan and its modified forms: a kinetic and thermodynamic study. Carbohydr Res. 2020;498:108185.
- Fuwa H. A new method for microdetermination of amylase activity by the use of amylose as the substrate. J Biochem. 1954;41:583–603.
- Lowry OH, Rosebrough NJ, Farr AL, et al. Protein measurement with the folin-phenol reagent. J Biol Chem. 1951;193:265–275.
- Ahmed SA, Saleh SAA, Abdel-Hameed SAM, et al. Catalytic, kinetic and thermodynamic properties of free and immobilized caseinase on mica glass-ceramics. Heliyon. 2019;5:e01674.
- Singh KA, Chhatpar HS. Purification and characterization of chitinase from paenibacillus sp. D1. Appl Biochem Biotechnol. 2011;164:77–88.
- Daoud L, Hmani H, Ben Ali M, et al. An original halo-alkaline protease from Bacillus halodurans strain US193: biochemical characterization and potential use as bio-additive in detergents. J Polym Environ. 2018;26:23–32.
- Ahmed SA, Mostafa FA, Ouis MA. Enhancement stability and catalytic activity of immobilized α-amylase using bioactive phospho-silicate glass as a novel inorganic support. Int J Biol Macromol. 2018;112:371–382.
- Abdel-Naby MA, Ahmed SA, Wehaidy HR, et al. Catalytic, kinetic and thermodynamic properties of stabilized Bacillus stearothermophilus alkaline protease. Int J Biol Macromol. 96:265–271. 2017;
- Silva RN, Asquieri ER, Fernandes KF. Immobilization of Aspergillus Niger glucoamylase onto a polyaniline polymer. Process Biochem. 2005;40:1155–1159.
- Bayramoglu G, Altintas B, Yakup Arica M. Immobilization of glucoamylase onto polyaniline-grafted magnetic hydrogel via adsorption and adsorption/cross-linking. Appl Microbiol Biotechnol. 2013;97:1149–1159.
- Dixon M, Webb EC, Thorne CJR, et al. Enzymes. New York: academic Press; 1979. p. 332–354.
- Toscano G, Pirozzi D, Maremonti M, et al. Kinetics of enzyme deactivation: a case study. Catal. Today. 1994;22:489–510.
- Prakash O, Jaiswal N. α, Amylase An ideal representative of thermostable enzymes. Appl Biochem Biotechnol. 2010;160:2401–2414.
- Savitha DP, Vadakethil Unniganapathi B, Geetha G, et al. Improvement in the properties of α-amylase enzyme by immobilization using metal oxide nanocomposites as carriers. Adv. Nanomed. Nanotechnol. Res. 2020;2:77–88.
- Ghosh S, Chaganti SR, Prakasham RS. Polyaniline nanofiber as a novel immobilization matrix for the anti-leukemia enzyme l-asparaginase. J Mol Catal B Enzym. 2012;74:132–137.
- Abdel-Naby MA, Hashem AM, Esawy MA, et al. Immobilization of Bacillus subtilis α-amylase and characterization of its enzymatic properties. Microbiol Res. 1999;153:319–325.
- Karam EA, Abdel Wahab WA, Saleh SAA, et al. Production, immobilization and thermodynamic studies of free and immobilized Aspergillus awamori amylase. Int J Biol Macromol. 2017;102:694–703.
- Ghosh P, Das A, Gayen S, et al. Statistical optimization of α-amylase production from penicillium notatum NCIM 923 and kinetics study of the purified enzyme. Acta Biol Szegediensis. 2015;59:179–188.
- Altinok H, Aksoy S, Tümtürk H, et al. Covalent immobilization of invertase on chemically activated poly (styrene-2-hydroxyethyl methacrylate) microbeads. J Food Biochemistry. 2008;32:299–315.
- Shojaei F, Homaei A, Taherizadeh MR, et al. Characterization of biosynthesized chitosan nanoparticles from Penaeus vannamei for the immobilization of P. vannamei protease: an eco-friendly nanobiocatalyst. Int J Food Prop. 2017;20:1–11.
- Molina-Fernández C, Luis P. Immobilization of carbonic anhydrase for CO2 capture and its industrial implementation: a review. J. CO2 Util. 2021;47:101475.
- Tavares APM, Silva CG, Dražić G, et al. Laccase immobilization over multi-walled carbon nanotubes: kinetic, thermodynamic and stability studies. J Colloid Interface Sci. 2015;454:52–60.
- Ahmed SA, Abdella MAA, El-Sherbiny GM, et al. Catalytic, kinetic and thermal properties of free andimmobilized Bacillus subtilis -MK1 α-amylase on chitosan-magnetic nanoparticles. Biotechnol Rep (Amst)). 2020;26:e00443.
- Marangoni AG. Enzyme kinetics: a modern approach. John Wiley & Sons; 2003. ISBN 0471461415
- Zaboli M, Raissi H, Zaboli M, et al. Stabilization of d-lactate dehydrogenase diagnostic enzyme via immobilization on pristine and carboxyl-functionalized carbon nanotubes, a combined experimental and molecular dynamics simulation study. Arch Biochem Biophys. 2019;661:178–186.
- Gohel SD, Singh SP. Purification strategies, characteristics and thermodynamic analysis of a highly thermostable alkaline protease from a salt-tolerant alkaliphilic actinomycete, Nocardiopsis Alba OK-5. J. Chromatogr. B. 2012;889-890:61–68.
- Pereira EB, De Castro HF, De Moraes FF, et al. Kinetic studies of lipase from Candida rugosa. Twenty-Second Symp Biotechnol Fuels Chem. 2001;91:739–752.
- Wehaidy HR, Abdel-Naby MA, Shousha WG, et al. Improving the catalytic, kinetic and thermodynamic properties of Bacillus subtilis KU710517 milk clotting enzyme via conjugation with polyethylene glycol. Int J Biol Macromol. 2018;111:296–301.
- Fernandez–Lafuente R, Guisan JM, Ali S, et al. Immobilization of functionally unstable catechol-2, 3-dioxygenase greatly improves operational stability. Enzyme Microb. Technol. 2000;26:568–573.