Abstract
Melatonin is a secondary messenger in plants that is involved in the regulation of abiotic stress responses, but it is unclear whether it enhances the adaptive capacity of blueberries to cope with salinity stress. The effects of exogenous application of melatonin (100, 200, and 300 μmol·L−1) on the growth, photosynthetic characteristics, leaf anatomy, and physiological and biochemical properties of blueberry plants under mixed salt stress (90 mmol·L−1: 30 mmol·L−1 Na2CO3 + 60 mmol·L−1 NaCl) were investigated. The results showed that blueberries under 90 mmol·L−1 mixed salt stress had significant salt damage symptoms, with reduced blueberry biomass, reduced chlorophyll content, and photosynthesis inhibition. In contrast, melatonin-treated blueberries showed significantly reduced salt damage symptoms compared with stressed blueberry plants, with the optimum effect at 200 μmol·L−1, along with increased chlorophyll content, improved photosynthetic capacity, and increased leaf thickness under stress. Melatonin treatment resulted in reduced malondialdehyde (MDA) content and relative electrolyte leakage (EL), increased K+ content, and decreased Na+ content in blueberry roots, stems and leaves, thereby reducing the toxic effects of mixed salts on blueberries. The results indicate that melatonin can mitigate the effects of mixed salt stress on blueberries and that foliar spraying of melatonin could alleviate mixed salt-induced damage to blueberries, with the optimum effect at 200 μmol·L−1 treatment. This study provided a valuable basis for exploring the mechanism of exogenous melatonin action in blueberries in saline environments.
Introduction
Globally, there are approximately one billion hectares of saline land, which is expanding due to anthropogenic and ecological–climatic factors, and this is one of the factors limiting agricultural development worldwide [Citation1–4]. The ability of plants to grow properly is inextricably linked to their environment. Plants require abundant minerals for growth and development, but saline soils disrupt the dynamic balance of ions in the soil, resulting in an unbalanced ion uptake and ion poisoning [Citation5]. As soil salinity increases, most plants become pale and develop wilted leaves, making recovery difficult [Citation6].
Salinity stress produces large amounts of reactive oxygen species (ROS) in plants, causing damage to the plant cytoplasmic membrane. The lipid membrane undergoes phase changes from a liquid–crystalline state to a gel state after salt stress, resulting in its structure changing from a lipid bilayer to a monolayer; this can consequently disrupt the functioning of the membrane and affect cellular metabolism [Citation7]. ROS scavenging systems in plants, such as superoxide dismutase, catalase, and ascorbate peroxidase, can enhance plants’ resistance to adverse conditions. These are important stress-response defence systems in plants that can effectively protect them from environmental stress and ensure their normal physiological activities [Citation8]. Osmoregulation is an important physiological mechanism for plants to cope with adversity, and plants synthesize organic substances, such as proline, betaine, sugars, and polyols, in response to salinity stress [Citation9–11].
Blueberry (Ericaceae, Vaccinium) is a small berry crop native to North America [Citation12]. Blueberries are rich in phytochemicals, including anthocyanin pigments, which may have the greatest impact on health functions. A regular and moderate intake of blueberries and/or anthocyanins can reduce the risk of cardiovascular diseases, death, and diabetes and improve weight maintenance and neuroprotection [Citation13–16]. Wright et al. studied the effect of different concentrations of NaCl irrigation water on blueberry and found that NaCl above 100 mmol·L−1 caused a large effect on above-ground parts and root dry weight. [Citation17].
The presence of melatonin (N-acetyl-5-methoxytryptamine) in plants has been observed by Hattori et al. [Citation18]. Several studies subsequently demonstrated the presence of melatonin in different plants and plant parts [Citation19,Citation20]. Melatonin has been widely reported to improve plant growth and development [Citation21–23]. For example, its application to the roots reduces the accumulation of toxic substances, such as hydrogen peroxide and malondialdehyde (MDA), in leaves as well as electrolytic leakage [Citation24]. Melatonin, a non-toxic biomolecule, is an important secondary messenger molecule that plays a vital role in coping with various abiotic and biotic stresses [Citation25]. Melatonin has been reported to be involved in the regulation and improvement of abiotic stresses such as saline environments [Citation26], drought [Citation27], high and low temperatures [Citation28]. Cheng et al.’s study showed that melatonin supplementation could effectively improve the inhibition of cotton seed germination by enhancing osmoregulatory substances and adjusting ion homeostasis under salt stress [Citation29]. What is more, under abiotic stress, plants usually increased the synthesis of endogenous melatonin [Citation30]. Melatonin pretreatment decreased ROS production and increased glutathione and ascorbic acid contents and superoxide dismutase, peroxidase, catalase, and ascorbate peroxidase activities in pea leaves under low temperature, salt, and drought stress, suggesting that melatonin protects pea plants from salt stress-induced damage by inhibiting ROS production and regulating antioxidant systems [Citation31]. This has also been demonstrated in other plants [Citation32–34].
Melatonin can also improve the photosynthetic performance of plants in saline environments.
Exogenous melatonin pretreatment increased the photosynthetic rate, maximum photosystem II photochemical efficiency and chlorophyll content of wheat leaves and partially alleviated the growth inhibition of wheat by excessive salt concentrations [Citation35]. The chlorophyll content of melon seeds treated with 50 μmol.L−1 melatonin was also significantly higher than that of untreated seeds [Citation36].
Melatonin is a secondary messenger molecule that plays a crucial role in responses to various abiotic stresses [Citation37,Citation38]. Exogenous melatonin treatment helps protect plants from salt stress by regulating the expression and activity of antioxidant enzymes, polyamine metabolism and nitric oxide signalling [Citation39]. In a previous study, melatonin was found to upregulate the expression of genes suppressed by salt stress, thereby reducing the inhibitory effect of salt stress on gene expression [Citation40,Citation41]. In another study, the transcript levels of the SlCOMT1 gene associated with melatonin biosynthesis were positively correlated with the content of melatonin in tomato leaves under stress, and SlCOMT1 transgenic tomatoes had lower superoxide and hydrogen peroxide levels and higher proline levels than wild-type tomatoes, suggesting that SlCOMT1 is closely associated with melatonin biosynthesis [Citation42].
In recent years, the activity of melatonin in plants has been comprehensively and intensively explored. In addition, the mechanisms of action associated with melatonin have been gradually revealed [Citation43]. However, it has not yet been tested in blueberries. Salts in the soil do not consist of a single salt, but are often a mixture of several salts. This study aimed to evaluate the effects of different melatonin concentrations on the growth and physiological characteristics of blueberry plants under mixed salt conditions and to determine its optimum concentration to alleviate mixed salt stress-induced damage to blueberry seedlings. This study reveals the physiological mechanism of exogenous melatonin on salt-stressed blueberry, which will lay the theoretical foundation for our work to further explore how melatonin induces the expression of resistance genes at the molecular level, as well as the synergistic or reciprocal regulation mechanism with phytohormones and other signalling molecules such as H2O2, Ca2+, and NO.
Materials and methods
Experimental materials and design
Test plants were selected from 2-year-old semi-highbush blueberry ‘Northland’ cultivars, and plant materials were provided by Jingyu County Branch of Jilin Changchun Pulan High-Tech Co.
Melatonin, modified Hoagland nutrient solution, and salt were purchased from Shanghai Biological Co. (Shanghai, China).
Trials were conducted in a greenhouse of the blueberry industrialization innovation and research practice base of Jilin Agricultural University in a solarium at 24–26 °C and 60% humidity. Grass charcoal (pH 4.5–5.3) was used as the cultivation substrate. Each pot had an outer diameter of 25 cm, an inner diameter of 20.5 cm, a height of 18 cm, a base diameter of 15.5 cm and was supplied with a tray. Approximately, 2.5 L of grass charcoal was used per pot. Two-year-old blueberry seedlings with almost identical growth rates were selected for treatments. Saline treatment was performed by adding NaCl and Na2CO3 to 1/10 modified Hoagland nutrient solution (pH 4.8) as follows: 90 mmol·L−1 mixed saline (30 mmol·L−1 Na2CO3 + 60 mmol·L−1 NaCl) and CK (No salt added, just an equal amount of 1/10 modified Hoagland nutrient solution) mixed with an equal volume of 1/10 modified Hoagland nutrient solution.
Melatonin was applied by foliar spray. Nine pots per treatment were sprayed with 20 mL of the corresponding melatonin concentration (0, 100, 200, and 300 μmol·L−1) per plant at a time (CK was sprayed with an equal volume of water) once every 2 d for a total of three sprays. Spraying was performed every 2 d between 17:00 and 18:00 each day, with the salt stress treatment being started the day after the last spray. Subsequently, 500 mL of saline treatment solution (1/10 modified Hoagland nutrient solution + 90 mmol·L−1 treatment solution) was applied per pot. To avoid salt shock, the 500 mL dose was distributed into five waterings a day, each time using 100 mL of saline. If the solution oozed from the bottom tray, the oozing solution was poured back into the original pot. The treatment time was calculated from the day after the last watering, and growth parameters and photosynthetic and physiological indicators were measured separately 30 d after the treatment.
Determination of growth parameters
After harvesting, three plants from each treatment were randomly selected and taken to the laboratory, where the plants were rinsed with deionized water. Next, the plants were quickly dried using gauze and their aboveground and belowground fresh weight was measured using a Mettler analytical balance (ME204E, Switzerland). Subsequently, the blueberry seedlings were dried to a constant weight using an electric blast dryer (GZX-9246MBE) and weighed separately using the Mettler analytical balance.
Leaf anatomy study
The leaf anatomy was studied using the paraffin section method. The samples were three mature fresh functional leaves randomly selected from each treatment.
Samples (whole fresh leaves) were fixed in fixative for over 24 h and then removed from the fixative and trimmed along the edge of the leaf with a scalpel in a fume hood, preserving the main leaf veins. The trimmed samples and corresponding labels were placed in a dehydration box.
The cassette was placed in a dehydrator (Donatello, DIAPATH, Italy) and dehydrated in a graded alcohol sequence. The protocol used included 75% alcohol for 4 h, 85% alcohol for 2 h, 90% alcohol for 2 h, 95% alcohol for 1 h, anhydrous ethanol I for 30 min, anhydrous ethanol II for 30 min, alcohol benzene for 5–10 min, xylene I for 5–10 min, xylene II for 5–10 min, 65° melted paraffin I for 1 h, 65° melted paraffin II for 1 h, 65° melted paraffin III 1 h.
The wax-soaked samples were embedded in an embedding machine (JB-P5, Wuhan Junjie Electronics Co). The melted wax was poured into the embedding frame and before it solidified, the samples were removed from the dehydration cassette, placed in the embedding frame according to the embedding surface and labelled with the corresponding label. The embedding frame was placed on a freezing platform (JB-L5, Wuhan Junjie Electronics Co., Ltd., China) at −20 °C for cooling. When the wax solidified, each wax block was removed from the embedding frame and was trimmed.
The modified sample chip wax blocks were cut into transverse 4-μm thick sections using a paraffin slicer (RM2016, Shanghai Leica Instruments Co). A slice was considered sufficiently flattened when the slice floated on 40 °C warm water in a spreading machine (KD-P, Cody Equipment Co. Ltd., China). Next, the samples were picked up using glass slides and baked in an oven at 60 °C. After melting the water-baked dried wax, it was removed and the samples were stored at 25 °C.
The sections were rehydrated in two changes of BioDewax and Clear Solution and 100%I% − 100%II − 75% alcohol. Each step took about 5 min. Finally, the samples were rinsed with running water. The sections were dipped into safranin O staining solution for 15–30 s and three cylinders of anhydrous ethanol for rapid dehydration. The sections were placed in a gradient of 50%, 70%, and 80% alcohol for 3–8 s each. The sections were inserted into plant solid green staining solution for 6 ∼ 20 s, followed by anhydrous ethanol three-cylinder dehydration. The sections were placed in clean xylene for 5 min and sealed with neutral resin. Finally, the tissue sections were mounted with neutral balsam.
Leaf thickness, upper epidermal thickness, lower epidermal thickness, palisade parenchyma thickness, and spongy parenchyma thickness were measured using the Motic Images Plus 3.0 (×64) software and observed and photographed using a Motic Panthera i (Fixed Koehler) microscope (MOTIC CHINA GROUP CO., LTD., China; https://www.motic.com/Biological/view_785.html).
Determination of physiological indicators
Chlorophyll content was determined using the method described by Wellburn [Citation44], in which 0.5 g of fresh leaves (veins removed) were subjected to chlorophyll extraction with 10 mL of 80% (v/v) precooled acetone in the dark for 24 h. Chlorophyll a and b contents were measured using the Hanon i9 UV–VIS spectrophotometer at 663 and 646 nm. Total chlorophyll content was determined as follows: Total chlorophyll = chlorophyll a + chlorophyll b.
Soluble protein (SP) content was determined using the Bradford method [Citation45]. The SP content was determined spectrophotometrically at 595 nm using bovine serum albumin as a standard and Coomassie Brilliant Blue G-250 dye.
The content of proline was tested according to Bates et al. [Citation46]. Briefly, 0.3 g of fresh leaves (veins removed) were weighed and ground into a homogenate in 10 mL of 3% aqueous salicylic acid solution and centrifuged with a high-speed refrigerated centrifuge at 4 °C for 4000 r min −1 for 15 min, after which 2 mL of the supernatant was reacted with 2 mL of acid ninhydrin and 2 mL of glacial acetic acid at 100 °C for 1 h. The reaction was incubated for 15 min on ice. Then, 4 mL of toluene was added and was shaken for 20 s. The absorption of the upper phase was measured with a spectrophotometer (Hanon i6, China) at 520 nm and the proline concentration was determined according to the standard curve of proline.
MDA content was determined using the thiobarbituric acid color development method [Citation47]. Briefly, 0.1 g of fresh leaf (veins removed) was weighed and grounded in 10 mL of 10% trichloroacetic acid. The tissue was then centrifuged at 4000 r.min−1 for 10 min. An aliquot of 2 mL of supernatant was then added to 2 mL of 0.5% thiobarbituric acid and heated at 100 °C for 15 min, followed by rapid cooling in an ice bath. The MDA content was calculated as follows: MDA (μmol.g−1 FW) = [6.45 (A532-A620) −0.56 A450] × Vt/FW, where Vt (mL) is the volume of the extract and FW (g) is the fresh weight of the plant sample.
Relative electrolyte leakage (EL) was determined as described by Restrepo et al. [Citation48]. About 0.1 g of fresh leaves was taken, cut into 5-mm segments, and placed in a test tube with 10 mL of deionized water. After allowing the test tubes to stand for 2 h at 30 °C, electrical conductivity (EC) (EC1) was measured using an EC meter (Remag DDSJ-308A, China). Next, the tubes were boiled in a water bath at 100 °C for 15 min and then EC was measured again (EC2). EL was calculated as follows: EL (%) = EC1/EC2 × 100.
Measurement of photosynthetic indicators
Plant photosynthesis was measured using the CIRAS-3 portable plant photosynthesis meter (PP systems, Amesbury, MA). Using a buffer bottle maintained at the same concentration as atmospheric CO2, with a CO2 concentration of around 400 μmol·mol−1, leaf temperature of 25 °C, and relative humidity of 50–70%, the measurement was performed in natural light after stabilizing the instrument. Three plants were randomly selected from each treatment, and two mature functional leaves from the upper middle part of each branch were selected to determine the net photosynthetic rate (Pn), transpiration rate (Tr), intercellular carbon dioxide concentration (Ci), and stomatal conductance (Gs). The experiment was repeated six times.
Determination of K+ and Na+ content
K+ and Na+ contents of plants were determined using a kit purchased from Suzhou Kemin Biotechnology Co. Ltd. (Suzhou, China). A standard solution of 1 mmol L−1 KCl and a standard solution of 1 mmol.L−1 NaCl mother liquor were prepared separately.
About 1 g of sample was taken, to which 5 mL of deionized water was added. It was homogenized and left for extraction for 24 h. Then, it was centrifuged at 1000 r.min−1 for 10 min at 25 °C, and the K+ and Na+ content in the supernatant was measured by a flame photometer (FP8400, Germany).
Sample determination
See Appendix 1 for details of standard curves. The samples were diluted appropriately if the concentration was above 1000 μmol L−1. The following formula was used for calculation: Potassium ion content (mg g−1 fresh weight) = C × V total × dilution factor ÷ W × 10−3 × 39; C: sample concentration, μmol L−1; V: total volume of extract added, 5 mL =0.005 L; W:sample mass, g.
The calculation formula was: Total sodium content (mg g−1 dry weight) = C × V total × dilution factor ÷ W × 10−3 × 22.99; C: sample concentration, μmol·L−1; V: total volume of extract added, 5 mL = 0.005 L; W: sample mass, g.
Statistical analysis
Data analysis was performed using Office 2016 and SPSS version 22.0 (SPSS Inc., Chicago, IL) [Citation49]. Analysis of variance (ANOVA) was used to determine significant differences. The level of statistical significance was set at p < 0.05. Data are presented as means with standard deviations (±SD); different lowercase letter(s) in bar charts indicate significant differences (α = 0.05, LSD) between treatments.
Results
Effect of exogenous melatonin application on the growth and biomass of blueberry seedlings under mixed salt stress
Compared with control (treatment 1 [T1]) plants, aboveground and belowground fresh and dry weights of blueberry plants were significantly lower 30 d after the last mixed salt stress treatment at 90 mmol·L−1 (). The blueberry seedlings also showed evident signs of salt damage, with leaves turning yellow (), and nearly 1 out of 2 leaves dropping (). All three melatonin concentrations reduced the biomass loss and alleviated the salt damage symptoms, with 200 mmol·L−1 melatonin (T4) being the most effective (, and ).
Figure 1. Effect of exogenous application of melatonin on the growth of blueberry seedlings at day 30 after mixed salt stress.
Note: The figure shows the growth of blueberry at the 30th day of treatments. Control T1: nonstressed plants, watered with 1/10 modified Hoagland nutrient solution; Control T2: watered with saline (90 mmol·L−1 mixed salt + 1/10 modified Hoagland nutrient solution); T3, T4, and T5: watered with sprays of 100, 200, and 300 μmol·L−1 melatonin solution and saline (90 mmol·L−1 mixed salt + 1/10 modified Hoagland nutrient solution), respectively.
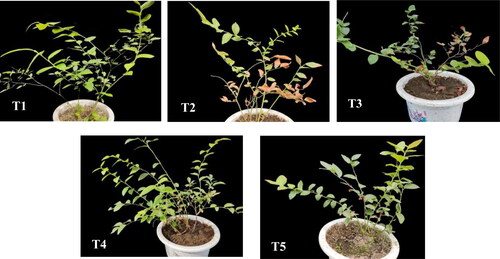
Figure 2. Effect of exogenous application of melatonin on blueberry leaves at day 30 after mixed salt stress.
Note: Control T1: nonstressed plants, watered with 1/10 modified Hoagland nutrient solution; Control T2: watered with saline (90 mmol·L−1 mixed salt + 1/10 modified Hoagland nutrient solution); T3, T4, and T5: watered with sprays of 100, 200, and 300 μmol·L−1 melatonin solution and saline (90 mmol·L−1 mixed salt + 1/10 modified Hoagland nutrient solution), respectively.
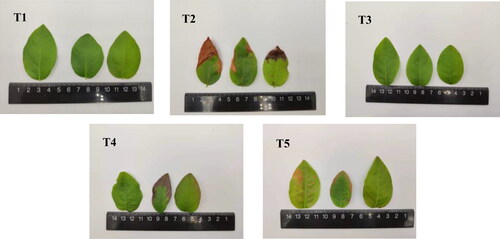
Table 1. Effects of exogenous application of melatonin on blueberry growth indicators under mixed salt stress.
Effect of exogenous application of melatonin on the anatomical structure of blueberry leaves under mixed salt stress
shows the cross-section of a mature functional leaf in the middle of the selected blueberry branches. Under stress (T2), blueberry leaf thickness, palisade parenchyma thickness, and spongy parenchyma thickness were significantly decreased by 19.99%, 25.50%, and 22.50%, respectively, compared with the control (T1; , ). All three melatonin concentrations increased the upper epidermal thickness, lower epidermal thickness, palisade parenchyma thickness, and spongy parenchyma thickness of blueberry leaves under stress (T2) by 34.11%, 92.48%, 35.11%, and 30.74%, respectively, with the highest increase at 200 μmol·L−1 melatonin (T4; , ).
Figure 3. Effects of exogenous application of melatonin on the anatomical structure of blueberry leaves under mixed salt stress.
Note: Blueberry leaf cross-sections at 400× magnification are shown; scale bars =1:50 μm. Control T1: nonstressed plants, watered with 1/10 modified Hoagland nutrient solution. Control T2: watered with saline (90 mmol·L−1 mixed salt + 1/10 modified Hoagland nutrient solution); T3, T4, and T5: watered with sprays of 100, 200, and 300 μmol·L−1 melatonin solution and saline (90 mmol·L−1 mixed salt + 1/10 modified Hoagland nutrient solution), respectively. S0: upper epidermis; S1: palysade parenchyma; S2: spongy parenchyma; S3: lower epidermis.
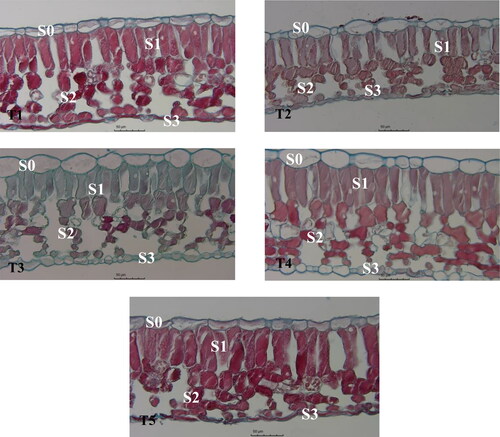
Table 2. Effects of exogenous application of melatonin on the anatomical structure of blueberry leaves under mixed salt stress.
Effect of exogenous application of melatonin on the physiology and biochemistry of blueberry leaves under mixed salt stress
The MDA and Pro content and EL of blueberry leaves under stress increased by 50.91%, 388.26%, and 56.23%, respectively, compared with the control (T1). Treatment with all concentrations of melatonin significantly reduced the EL and MDA content of leaves under stress, with 200 μmol.L−1 melatonin being the most effective of all three concentrations, reducing leaf EL and MDA content by 30.17% and 33.93%, respectively, compared with stress (T2). The SP and Pro contents of blueberry leaves were higher under all three concentrations of exogenous melatonin treatment than those under stress treatment (T2), with the 200 μmol-L−1 melatonin treatment resulting in the highest increase of 12.21% and 19.55%, respectively ().
Figure 4. Effect of exogenous application of melatonin on the physiological characteristics of blueberry under mixed salt stress.
Note: Control T1: nonstressed plants, watered with 1/10 modified Hoagland nutrient solution. Control T2: watered with saline (90 mmol·L − 1 mixed salt + 1/10 modified Hoagland nutrient solution); T3, T4, and T5: watered with sprays of 100, 200, and 300 μmol·L − 1 melatonin solution and saline (90 mmol·L − 1 mixed salt + 1/10 modified Hoagland nutrient solution), respectively. Data represent the mean ± SD of three trials for each treatment. Different letters within each panel indicate significant differences (p < 0.05).
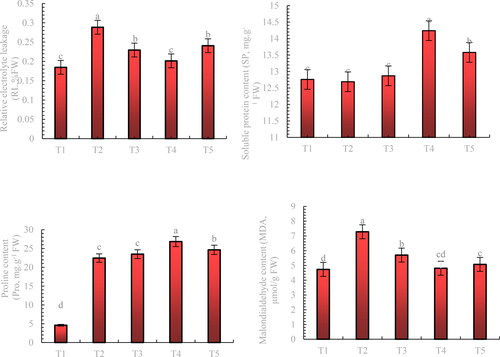
Effect of exogenous application of melatonin on the chlorophyll content and photosynthetic properties of blueberry leaves under mixed salt stress
Pn, Tr, and Gs of blueberry leaves were reduced to varying degrees under stress, while Ci was significantly increased (). Exogenous application of melatonin increased leaf Pn, Tr, and Gs by 77.55%, 131.37%, and 149.22%, respectively, compared with the stress treatment (T2), with 200 μmol·L−1 melatonin treatment (T4) exhibiting the most significant increase. Treatment with 200 μmol·L−1 (T4) and 300 μmol·L−1 (T5) melatonin significantly reduced the leaf Ci of blueberry leaves under stress and maintained it in the control (T1).
Figure 5. Effects of exogenous application of melatonin on the photosynthetic properties of blueberry leaves under mixed salt stress.
Note: Control T1: nonstressed plants, watered with 1/10 modified Hoagland nutrient solution. Control T2: watered with saline (90 mmol·L − 1 mixed salt + 1/10 modified Hoagland nutrient solution); T3, T4, and T5: watered with sprays of 100, 200, and 300 μmol·L − 1 melatonin solution and saline (90 mmol·L − 1 mixed salt + 1/10 modified Hoagland nutrient solution), respectively. Data represent the mean ± SD of three trials for eachv treatment. Different letters within each panel indicate significant differences (p < 0.05).
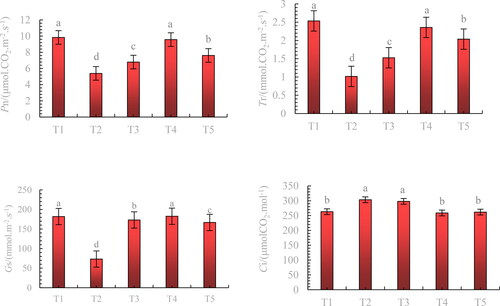
Mixed salt stress reduced the photosynthetic pigment content of blueberry leaves (). Chlorophyll a + b, chlorophyll a, and chlorophyll b contents of blueberry leaves increased to varying degrees after melatonin treatment, with 200 μmol·L−1 melatonin (T4) being the most effective ().
Figure 6. Effect of exogenous application of melatonin on the chlorophyll content of blueberry leaves under mixed salt stress.
Note: Control T1: nonstressed plants, watered with 1/10 modified Hoagland nutrient solution. Control T2: watered with saline (90 mmol·L − 1 mixed salt + 1/10 modified Hoagland nutrient solution); T3, T4, and T5: watered with sprays of 100, 200, and 300 μmol·L − 1 melatonin solution and saline (90 mmol·L − 1 mixed salt + 1/10 modified Hoagland nutrient solution), respectively. Data represent the mean ± SD of three trials for each treatment. Different letters within each panel indicate significant differences (p < 0.05).
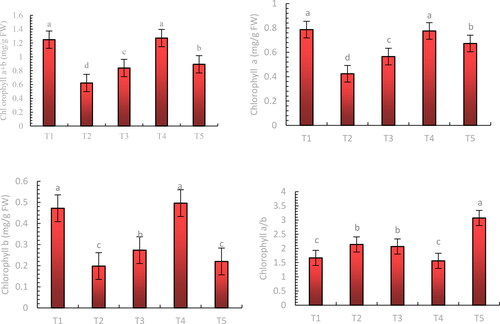
Effect of exogenous application of melatonin on the Na+ and K+ contents of blueberry roots, stems, and leaves under mixed salt stress
In normally growing (CK) blueberries (T1), the Na+ and K+ contents of leaves were higher than those of roots and stems (). Under stress (T2), blueberry root, stem and leaf K+ contents were reduced by 28.66%, 15.82%, and 52.88%, respectively, compared with the control (T1). In contrast, the Na+ content in root, stem and leaf increased by 100.97%, 102.91%, and 786.4%, respectively, compared with the control. The K+ content of the leaves decreased more than that of the root and stem. Na+ accumulation was also higher in the leaves than in the roots and stems. Exogenous melatonin application increased the K+ content of the roots, stems, and leaves under mixed salt stress (T2) to varying degrees, with the greatest increase in K+ content in the roots (29.91%) and leaves (70%) under the 200 μmol·L−1 treatment (T4) compared with the stress treatment (T2). The greatest increase in K+ content in stems was observed under the 300 μmol·L−1 treatment (T5), with an increase of 9.57% compared to that in the stress treatment (T2). Exogenous melatonin application reduced the Na+ content of the roots, stems, and leaves under mixed salt stress to varying degrees, with the most significant reduction in Na+ content under the 200 μmol·L−1 treatment (T4), with 45.41%, 38.28%, and 56.86% compared to the stress treatment (T2), respectively.
Table 3. Effect of exogenous application of melatonin on the Na+ and K+ contents of blueberry roots, stems, and leaves under mixed salt stress.
Discussion
Highly saline soils inhibit plant growth mainly because the high osmotic potential of the soil solution reduces the uptake of nutrients and water by plants, which mainly limits the crop yields in saline areas [Citation50]. In this experiment, blueberries under 90 μmol.L−1 mixed salt stress showed significant salt damage symptoms and reduced biomass because of excessively high salt concentrations, which led to a reduction in soil water potential, resulting in higher soil osmotic pressure than plant cell osmotic pressure. This can lead to osmotic stress from plant water loss and can trigger physiological drought [Citation51]. Melatonin is involved in regulatory physiological processes in plants, such as improving plant germination and maturation processes, photosynthetic activity, biomass production, and root development [Citation52]. In melatonin-treated blueberry plants, melatonin significantly reduced salt damage symptoms and alleviated the damage caused by mixed salt stress, with melatonin at 200 μmol.L−1 significantly reducing the damage caused by mixed salt stress on the growth of blueberry and effectively increasing its biomass. This is consistent with the results of several studies [Citation53–56].
The leaf is the most sensitive and plastic organ of plants in response to environmental changes, and in different ecological environments, it develops different adaptation patterns. The internal anatomy of the leaf can be significantly altered in saline environments [Citation57]. A previous study showed that melatonin-treated tomato leaf flesh tissue was significantly thickened compared with acid rain-stressed tomato leaf flesh tissue [Citation30]. In this study, blueberry leaf thickness, palysade parenchyma thickness, and spongy parenchyma thickness were all significantly reduced under 90 mmol L−1 mixed salt stress. Exogenous melatonin application significantly increased leaf thickness, upper epidermal thickness, lower epidermal thickness, spongy parenchyma thickness, and palysade parenchyma thickness in blueberry compared with mixed salt stress treatment, with 200 μmol.L−1 melatonin treatment yielding the highest values. It could be speculated that the exogenous melatonin treatment also reduced the damage to the cellular structure of leaf mesophyl by mixed salt stress, probably due to the increase in osmoregulatory substances, such as intracellular proline, and the detoxification of excess ROS from chloroplasts by the antioxidant system, thus reducing the degree of damage to the plant cell membrane system and protecting the structural integrity of the leaf mesophyl. Leaf thickness and internal structure are important characteristics that reflect the plants’ tolerance to salt environments, and salt-tolerant plants usually have thicker leaves and are better adapted to saline environments [Citation58], suggesting that melatonin can improve the salt tolerance of blueberries while alleviating mixed salt stress.
Osmoregulation is an important physiological mechanism for plants to cope with adversity, and proline, as one of the osmoregulatory substances in plants, plays an important role in maintaining plant growth under various abiotic stresses [Citation9,Citation10]. Salt stress usually disrupts plant cell membrane permeability and integrity, causing membrane lipid peroxidation and thereby increasing membrane permeability [Citation59]. Our results showed that under mixed salt stress, the relative EL from blueberry leaves increased, while large amounts of MDA accumulated in the leaves. Park et al. [Citation60] suggested that increased melatonin concentrations would reduce MDA levels in transgenic rice. Jiang et al. [Citation61] also found that exogenous melatonin application reduced EL and MDA levels by 25% and 22%, respectively. In this study, melatonin treatment reduced the relative EL and MDA content of blueberry leaves and increased the levels of osmoregulatory substances (SP and Pro), and the increase in leaf Pro content resulted in an increase in salt tolerance. The results showed that melatonin treatment can effectively inhibit ROS production in the leaves of blueberry seedlings under mixed salt stress conditions, reduce membrane lipid peroxidation, protect membrane integrity, reduce EL, and mitigate saline environment-induced damage to blueberry seedlings while increasing the levels of osmoregulatory substances to improve the salt tolerance of blueberry seedlings.
Chloroplasts are the main site for photosynthesis in plants, and reduction in chlorophyll content in chloroplasts can inhibit the uptake and use of light energy by plants [Citation62]. Ma et al. [Citation63] found that salt stress reduces chlorophyll content, leading to a decrease in photosynthetic enzyme activity and photosynthetic phenotypic quantum efficiency. In this study, we found that a 90 mmol.L−1 salt mixture caused a reduction in chlorophyll content in blueberry leaves, leading to a reduction in photosynthetic capacity. This is because plants normally contract leaf stomata and reduce Gs under salt stress, limiting the transport of CO2 to chloroplasts and evaporation of leaf water, thereby inhibiting leaf photosynthesis and transpiration. In contrast, the photosynthetic capacity and chlorophyll content of blueberries were significantly improved by exogenous melatonin application, with the best results obtained under the 200 μmol.L−1 treatment. In a similar study, Wang et al. [Citation64] found that 200 mmol.L−1 NaCl damaged 27.7% of chloroplasts in cucumber, and the number of damaged chloroplasts decreased to 20.6% under the 100 μmol.L−1 melatonin treatment, suggesting that melatonin is beneficial in alleviating salt stress-induced damage to the ultrastructure of chloroplasts while decreasing the degradation rate of chlorophyll. This further leads to an increase in the chlorophyll content and improves the photosynthetic rate of plants [Citation65].
Under salt stress, an increased salt uptake by plants inhibits the uptake of other nutrients, leading to nutrient ion deficiency or imbalance. The most common single salt poisoning is Na+ poisoning, where high Na+ concentrations can displace the structural integrity of the cell membrane and disrupt its function, resulting in the leakage of potassium, phosphorus, and organic solutes from cells [Citation6]. There is a competition between K+ and Na+, with high concentrations of Na+ inhibiting the K+ uptake of plants [Citation5]. In contrast, the more resistant apple JM2 accumulated less Na ions in the leaves under 150 mM NaCl conditions and was subjected to less osmotic stress[Citation66]. In this study, the Na+ content of blueberry roots, stems, and leaves increased significantly under the 90 mmol.L−1 mixed salt treatment, whereas K+ content decreased significantly, similar to the results observed in poplar [Citation67] and aloe vera [Citation68]. Li et al. [Citation40] concluded that violets pretreated with melatonin had significantly reduced Na+ and Cl− content and increased K+ content in the leaves under salt stress. In another study, the K+ content and K+/Na+ ratio in salt-affected maize shoots increased significantly by 18% and 52%, respectively, and the Na+ content in leaves decreased significantly after melatonin treatment [Citation61]. In this study, we found that the Na+ content of blueberry roots, stems, and leaves was reduced by external melatonin application, with the highest reduction achieved with 200 μmol.L−1 melatonin treatment, while K+ content in roots and leaves was increased at different melatonin concentrations, with the highest increase achieved with 200 μmol.L−1 melatonin treatment. This indicates that exogenous application of melatonin can reduce the Na+ content of blueberry organs while increasing their K+ content to maintain ionic balance and reduce salt stress-induced damage to blueberry.
We intend to further investigate how exogenous melatonin induces genetic differences in blueberry under salt stress and explore the mechanisms of interaction between melatonin and other signalling molecules, which will help to reveal the facilitation and mechanisms of action of chemical regulators, and screen new plant growth regulators to mitigate salt stress damage, promote plant recovery, and reduce disaster losses. Although melatonin spraying enhances adaptation to salt stress, the effectiveness of single application in production is still limited. Therefore, melatonin should be used as a basis for developing more effective combinations. In the meantime, the dosage effects of melatonin and its formulations in modulating plant responses to salt stress should be further investigated to determine the optimum concentration for its function and to develop precise application techniques in conjunction with its synthetic and metabolic mechanisms.
Conclusions
Our findings revealed that blueberry ‘Northland’ under 90 mmol.L−1 mixed salt stress inhibited seedling growth and reduced their biomass, chlorophyll content, and photosynthetic capacity while increasing MDA content and relative EL from the leaves, which eventually dried out and began to fall off. Melatonin application to the leaf surface significantly improved its growth, biomass, photosynthetic capacity, and ionic balance regulation while decreasing lipid peroxidation-induced damage to the cell membrane. These results suggest that melatonin is effective in mitigating the damage caused by mixed salts to blueberry seedlings. Among the melatonin concentrations examined in this study, the best results were obtained with 200 μmol.L−1 melatonin, which was also consistent with the results of morphological evaluation of blueberries.
Author contributions
Conceptualization, W.J. and X.W.; Methodology, W.J.; Software, Y.W.; Validation, J.M., L.W. and L.L.; Formal Analysis, J.L.; Investigation, J.L.; Resources, L.W.; Data Curation, W.J.; Writing – Original Draft Preparation, W.J.; Writing – Review & Editing, L.W.; Visualization, Y.W.; Supervision, Y.W.; Project Administration, L.W.; Funding Acquisition, Y.W.
Acknowledgements
The authors would like to express their gratitude to the Fruit Quality Analysis Laboratory, Jilin Province Blueberry Research Centre for providing the materials used for the experiments and assistance during this study.
Disclosure statement
The authors declare no conflict of interest.
Data availability statement
All data included in this study are available upon request by contact with the corresponding authors.
Additional information
Funding
References
- Hassani A, Azapagic A, Shokri N. Predicting long-term dynamics of soil salinity and sodicity on a global scale. Proc Natl Acad Sci USA. 2020;117(52):1–14.
- Hwang WM, Kim D, Kang K, et al. Flavobacterium eburneum sp. nov., isolated from reclaimed saline land soil. Int J Syst Evol Microbiol. 2017;67(1):55–59.
- Yan YY, Wang SS, Wei M, et al. Effect of different rootstocks on the salt stress tolerance in watermelon seedlings. Hortic Plant J. 2018;4(6):239–249.
- Rengasamy P. World salinization with emphasis on Australia. J Exp Bot. 2006;57(5):1017–1023.
- Munns R, Tester M. Mechanisms of salinity tolerance. Annu Rev Plant Biol. 2008;59(1):651–681.
- van Zelm E, Zhang Y, Testerink C. Salt tolerance mechanisms of plants. Annu Rev Plant Biol. 2020;71:403–433.
- Chinnusamy V, Jagendorf A, Zhu JK. Understanding and improving salt tolerance in plants. Crop Sci. 2005;45(2):437–448.
- Sun TT, Wang C, Liu R, et al. ThHSFA1 confers salt stress tolerance through modulation of reactive oxygen species scavenging by directly regulating ThWRKY4. Int J Mol Sci. 2021;22(9):5048.
- Rady MM, Hemida KA. Sequenced application of ascorbate-proline-glutathione improves salt tolerance in maize seedlings. Ecotoxicol Environ Saf. 2016;133:252–259.
- Ozturk M, Turkyilmaz Unal B, García-Caparrós P, et al. Osmoregulation and its actions during the drought stress in plants. Physiol Plant. 2021;172(2):1321–1335.
- El Moukhtari A, Cabassa-Hourton C, Farissi M, et al. How does proline treatment promote salt stress tolerance during crop plant development? Front Plant Sci. 2020;11:1127.
- Vander Kloet SP. The genus vaccinium in North america. Canadian Institute of International Affairs. 1988;101(6):2609–2625.
- Miller K, Feucht W, Schmid M. Bioactive compounds of strawberry and blueberry and their potential health effects based on human intervention studies: a brief overview. Nutrients. 2019;11(7):1510.
- Boespflug EL, Eliassen JC, Dudley JA, et al. Enhanced neural activation with blueberry supplementation in mild cognitive impairment. Nutr Neurosci. 2018;21(4):297–305.
- Silva S, Costa EM, Veiga M, et al. Health promoting properties of blueberries: a review. Crit Rev Food Sci Nutr. 2020;60(2):181–200.
- Kalt W, Cassidy A, Howard LR, et al. Recent research on the health benefits of blueberries and their anthocyanins. Adv Nutr. 2020;11(2):224–236.
- Wright GC, Patten KD, Drew MC. Salinity and supplemental calciumInfluence growth of rabbiteye and Southern highbush blueberry. Jashs. 1992;117(5):749–756.
- Hattori A, Migitaka H, Iigo M, et al. Identification of melatonin in plants and its effects on plasma melatonin levels and binding to melatonin receptors in vertebrates. Biochem Mol Biol Int. 1995;35(3):627–634.
- Xia H, Shen Y, Shen T, et al. Melatonin accumulation in sweet cherry and its influence on fruit quality and antioxidant properties. Molecules. 2020;25(3):753.
- Dubbels R, Reiter RJ, Klenke E, et al. Melatonin in edible plants identified by radioimmunoassay and by high performance liquid chromatography-mass spectrometry. J Pineal Res. 1995;18(1):28–31.
- Wang K, Xing Q, Ahammed GJ, et al. Functions and prospects of melatonin in plant growth, yield, and quality. J Exp Bot. 2022;73(17):5928–5946.
- Qiao Y, Yin L, Wang B, et al. Melatonin promotes plant growth by increasing nitrogen uptake and assimilation under nitrogen deficient condition in winter wheat. Plant Physiol Biochem. 2019;139:342–349.
- Tan X, Long W, Zeng L, et al. Melatonin-induced transcriptome variation of rapeseed seedlings under salt stress. Int J Mol Sci. 2019;20(21):5355.
- Vafadar F, Amooaghaie R, Ehsanzadeh P, et al. Crosstalk between melatonin and Ca2+/CaM evokes systemic salt tolerance in dracocephalum kotschyi. J Plant Physiol. 2020;252:153237.
- Sharif R, Xie C, Zhang H, et al. Melatonin and its effects on plant systems. Molecules. 2018;23(9):2352.
- Liu TT, Xing GM, Chen ZF, et al. Effect of exogenous melatonin on salt stress in cucumber: alleviating effect and molecular basis. Biotechnol Biotechnol Equipment. 2022;36(1):818–827.
- Wang P, Sun X, Li C, et al. Long-term exogenous application of melatonin delays drought-induced leaf senescence in apple. J Pineal Res. 2013;54(3):292–302.
- Ayyaz A, Shahzadi AK, Fatima S, et al. Uncovering the role of melatonin in plant stress tolerance. Theor Exp Plant Physiol. 2022;34(3):335–346.
- Chen L, Liu L, Lu B, et al. Exogenous melatonin promotes seed germination and osmotic regulation under salt stress in cotton (gossypium hirsutum L.). PLoS One. 2020;15(1):e0228241.
- Debnath B, Hussain M, Irshad M, et al. Exogenous melatonin mitigates acid rain stress to tomato plants through modulation of leaf ultrastructure, photosynthesis and antioxidant potential. Molecules. 2018;23(2):388–403.
- Butsanets PA, Baik AS, Shugaev AG, et al. Melatonin inhibits peroxide production in plant mitochondria. Dokl Biochem Biophys. 2019;489(1):367–369.
- Arnao MB, Hernández-Ruiz J. Melatonin: plant growth regulator and/or biostimulator during stress? Trends Plant Sci. 2014;19(12):789–797.
- Yang Y, Cao Y, Li Z, et al. Interactive effects of exogenous melatonin and rhizophagus intraradices on saline-alkaline stress tolerance in leymus chinensis. Mycorrhiza. 2020;30(2–3):357–371.
- Zahedi SM, Hosseini MS, Abadía J, et al. Melatonin foliar sprays elicit salinity stress tolerance and enhance fruit yield and quality in strawberry (fragaria × ananassa duch.). Plant Physiol Biochem. 2020;149:313–323.
- Ke Q, Ye J, Wang B, et al. Melatonin mitigates salt stress in wheat seedlings by modulating polyamine metabolism. Front Plant Sci. 2018;9:914.
- Luis Castañares J, Alberto Bouzo C. Effect of exogenous melatonin on seed germination and seedling growth in melon (cucumis melo l.) under salt stress. Hortic Plant J. 2019;5(2):79–87.
- Zhang N, Sun Q, Zhang H, et al. Roles of melatonin in abiotic stress resistance in plants. J Exp Bot. 2015;66(3):647–656.
- Back K. Melatonin metabolism, signaling and possible roles in plants. Plant J. 2021;105(2):376–391.
- Zhan H, Nie X, Zhang T, et al. Melatonin: a small molecule but important for salt stress tolerance in plants. Int J Mol Sci. 2019;20(3):709.
- Li J, Yuan F, Liu Y, et al. Exogenous melatonin enhances salt secretion from salt glands by upregulating the expression of ion transporter and vesicle transport genes in Limonium bicolor. BMC Plant Biol. 2020;20(1):493.
- Wei W, Li QT, Chu YN, et al. Melatonin enhances plant growth and abiotic stress tolerance in soybean plants. J Exp Bot. 2015;66(3):695–707.
- Liu DD, Sun XS, Liu L, et al. Overexpression of the melatonin synthesis-related gene SlCOMT1 improves the resistance of tomato to salt stress. Molecules. 2019;24(8):1514.
- Debnath B, Islam W, Li M, et al. Melatonin mediates enhancement of stress tolerance in plants. IJMS. 2019;20(5):1040.
- Wellburn AR. The spectral determination of chlorophylls a and b, as well as total carotenoids, using various solvents with spectrophotometers of different resolution. J Plant Physiol. 1994;144(3):307–313.
- Bradford MM. A rapid and sensitive method for the quantitation of microgram quantities of protein utilizing the principle of protein-dye binding. Anal Biochem. 1976;72:248–254.
- Bates LS, Waldren RP, Teare ID. Rapid determination of free proline for water-stress studies. Plant Soil. 1973;39(1):205–207.
- Heath RL, Packer L. Photoperoxidation in isolated chloroplasts. I. Kinetics and stoichiometry of fatty acid peroxidation. Arch Biochem Biophys. 1968;125(1):189–198.
- Restrepo H, Gómez MI, Garzón A, et al. Respuesta bioquímica de plántulasde maíz (Zea mays L.) a diferentes condiciones de temperaturas nocturnas. Rev Colomb Cienc Hortic. 2014;7(2):252–262.
- Jiang D, Lu B, Liu L, et al. Exogenous melatonin improves the salt tolerance of cotton by removing active oxygen and protecting photosynthetic organs. BMC Plant Biol. 2021;21(1):331.
- Guo H, Huang Z, Li M, et al. Growth, ionic homeostasis, and physiological responses of cotton under different salt and alkali stresses. Sci Rep. 2020;10(1):21844.
- Hossain, Akbar, editor. Plant stress physiology. London: IntechOpen; 2021.
- Asif M, Pervez A, Irshad U, et al. Melatonin and plant growth-promoting rhizobacteria alleviate the cadmium andarsenic stresses and increase the growth of Spinacia oleracea L. Plant Soil Environ. 2020;66(5):234–241.
- Martinez V, Nieves-Cordones M, Lopez-Delacalle M, et al. Tolerance to stress combination in tomato plants: new insights in the protective role of melatonin. Molecules. 2018;23(3):535.
- Lee HY, Byeon Y, Back K. Melatonin as a signal molecule triggering defense responses against pathogen attack in arabidopsis and tobacco. J Pineal Res. 2014;57(3):262–268.
- Qian Y, Tan DX, Reiter RJ, et al. Comparative metabolomic analysis highlights the involvement of sugars and glycerol in melatonin-mediated innate immunity against bacterial pathogen in arabidopsis. Sci Rep. 2015;5:15815.
- Shi H, Jiang C, Ye T, et al. Comparative physiological, metabolomic, and transcriptomic analyses reveal mechanisms of improved abiotic stress resistance in bermudagrass [cynodon dactylon (L). pers.] by exogenous melatonin. J Exp Bot. 2015;66(3):681–694.
- Bozdag B, Ozdemir A, Hamurcu M, et al. Numerical and statistical evaluation of nitric oxide effect on leaf anatomy of Triticum genotypes under salinity stress. J Microbiol Exp. 2020;8(4):140–146.
- Yuan F, Guo J, Shabala S, et al. Reproductive physiology of halophytes: current standing. Front Plant Sci. 2018;9:9, 1954.
- Fu M, Li C, Ma F. Physiological responses and tolerance to NaCl stress in different biotypes of malus prunifolia. Euphytica. 2013;189(1):101–109.
- Park S, Lee DE, Jang H, et al. Melatonin-rich transgenic rice plants exhibit resistance to herbicide-induced oxidative stress. J Pineal Res. 2013;54(3):258–263.
- Jiang C, Cui Q, Feng K, et al. Melatonin improves antioxidant capacity and ion homeostasis and enhances salt tolerance in maize seedlings. Acta Physiol Plant. 2016;38(4):82.
- Zhang H, Zhong H, Wang J, et al. Adaptive changes in chlorophyll content and photosynthetic features to low light in physocarpus amurensis maxim and physocarpus opulifolius “diabolo. PeerJ. 2016;4:e2125.
- Ma Q, Yue LJ, Zhang JL, et al. Sodium chloride improves photosynthesis and water status in the succulent xerophyte zygophyllum xanthoxylum. Tree Physiol. 2012;32(1):4–13.
- Wang LY, Liu JL, Wang WX, et al. Exogenous melatonin improves growth and photosynthetic capacity of cucumber under salinity-induced stress. Photosynt. 2016;54(1):19–27.
- Shi H, Chen Y, Tan DX, et al. Melatonin induces nitric oxide and the potential mechanisms relate to innate immunity against bacterial pathogen infection in arabidopsis. J Pineal Res. 2015;59(1):102–108.
- Matsumoto K, Kobayashi T. Relative tolerance of Japanese apple (malus spp.) rootstock strains to NaCl stress. Acta Hortic. 2020;1289(1289):9–18.
- Jiang C, Zheng Q, Liu Z, et al. Overexpression of Arabidopsis thaliana Na+/H+ antiporter gene enhanced salt resistance in transgenic poplar (populus × euramericana ‘neva’). Trees. 2012;26(3):685–694.
- Jiang CQ, Quan LT, Shi F, et al. Distribution of mineral nutrients and active ingredients in aloe vera irrigated with diluted seawater. Pedosphere. 2014;24(6):722–730.
Appendix 1
Standard curve plotting(K+)
Standard curve plotting(Na+)