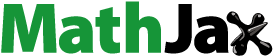
Abstract
Nanoparticles may serve as novel plant growth stimulators to improve plant growth as well as protect plants from abiotic stresses. In this study, biogenic silver nanoparticles (AgNPs) were synthesized using the aqueous extract of Fagonia bruguieri and characterized using UV-visible spectroscopy, Zeta potential, transmission electron microscopy (TEM) and Fourier Transform Infrared Spectroscopy (FTIR). The synthesized AgNPs were employed on Echinops macrochaetus as seeds priming, and plants grown in pots were exposed to salinity stress to assess the ameliorating effects based on various parameters. The growth parameters (shoot length) and biomass (shoot, root and leaves weight) decreased in E. macrochaetus exposed to salinity stress alone (40, 80 and 120 mmol/L NaCl) for 90 days. However, seed priming with a low concentration of AgNPs (40 µmol/L) improved the growth of the plant in terms of biomass, shoot length and chlorophyll content alone as well as ameliorated the toxic effect caused by salinity stress. The mitotic index measured by flowcytometry (FCM) indicated genotoxic effects in plants grown at high concentrations of NaCl (80 and 120 mmol/L) as well as plants obtained with 80 µmol/L AgNPs seed priming and exposed to 120 mmol/L NaCl. Thus, an optimum concentration of AgNPs could be used in E. macrochaetus for production of more biomass by modulating the chlorophyll content, proline and antioxidant enzyme activity.
Introduction
Salinity is one of the environmental factor that disturb the plant growth, physiological parameters and ionic concentrations [Citation1]. Salinity continues to rise in arable land due to cultivation practices and climate change, and it is projected that about 50% of arable land will be vanished by the middle of the 21st first century [Citation2]. Salinity stress also leads to elevated levels of reactive oxygen species (ROS) and, consequently, to oxidative stress in plants, which in turn disturbs the cellular and metabolic processes [Citation3]. Soil salinity and its adverse effects on photosynthetic parameters, nutritional imbalance, seed germination, plant growth, crop yield, carotenoid and chlorophyll pigments, plant/water relationship (hydration of plant cells, and water movement within a plant) and oxidative stress have been studied in plants [Citation4–6]. To deal with high salt stress, plants improve their biochemical and physiological responses involved in oxidative damage control, osmotic homeostasis and detoxification [Citation7,Citation8]. Therefore, there is growing interest in the development of inexpensive strategies that can improve the physiology, morphology and biochemistry of the plants under salinity stress.
The application of nanotechnology has been explored in various plant species, although optimized dose application in agriculture varies in different plant species. Various types of nanoparticles (NPs) have been studied for their potential use in enhancing the defense mechanisms against abiotic and biotic stresses. Some NPs have been effective in alleviating the toxic effects of salt stress in different plants [Citation9]. NPs have distinctive physicochemical properties and potential to improve plant growth and metabolism [Citation10]. Among the various types of nanoparticles, silver nanoparticles (AgNPs) are one of the commonly used nanomaterials in various fields, mainly in the agricultural sector [Citation11]. AgNPs have been proven as plant-growth stimulators in the agriculture segment [Citation12,Citation13]. The effect of AgNPs depends on the age and species of the experimental plants. Moreover, NPs concentration and size are also very important, as they affect the plant growth. The AgNPs has been shown to have a great ability to scavenge free radicals by making phytochemicals, leading to a significant reduction in hydrogen peroxide and levels of malondialdehyde [Citation14–16].
Echinops genus belongs to family Asteraceae [Citation17] and is found in Africa and Asia. Various phytoconstituents are found in the roots of Echinops macrochaetus, including stigmasterol and 5-(but-3-en-1-ynyl)-2,2′-bithiophene and 5-(penta-1,3-diynyl)-2-(3-chloro-4-hydoxy-but-1-ynyl)- thiophene [Citation18,Citation19]. Thiophenes is the important bioactive components of the genus Echinops and is biosynthetically derived from reduced sulfur and fatty acids [Citation20]. Some bioactive compounds such as Macrochaetosides A, Macrochaetosides B and cyclostenol were extracted from E. macrochaetus which showed cytotoxic activity [Citation19]. In our previous study, the leaves, roots and shoots of E. macrochaetus showed the presence of potential secondary metabolites including luteolin 7-rutinoside, quercetin-3-β-D-glucoside and p-coumaric acid [Citation21]. The biogenic zinc nanoparticles improved the growth parameters of E. macrochaetus as well as phytochemicals [Citation21]. Due to presence of various medicinal valued compounds, the plant has great importance for the treatment of various diseases. However, E. macrochaetus is a wild medicinal plant and few populations are found in different geographical regions of Saudi Arabia [Citation21]. This low population number of E. macrochaetus might be due to various abiotic stresses prevailing in natural habitats. Therefore, there is a need to explore the salinity tolerance of this species using various morphological and biochemical parameters. By keeping in mind the great biological potential of biogenic silver nanoparticles and vast range of biological activities, the purpose of this study was to explore the possible effects of various concentrations of AgNPs seed priming on morphological, biochemical and genetic attributes of E. macrochaetus alone as well as under salinity stress.
Materials and methods
Chemicals for AgNPs synthesis
Silver nitrate (AgNO3) was purchased from Merck (Cat: 101512, USA). All aqueous solutions were prepared using double distilled deionized water. The chemicals used in this study were of analytical grade.
Plant extract preparation
Fagonia bruguieri plant was collected from the wild environment (Voucher No: KSU24093), since it grows commonly in Saudi Arabia. The plant was identified using the internal transcribed spacer sequence of nrDNA (GenBank accession number: KJ004309). The leaves were dried in shade and ground into fine powder. Leaf powder (5 g) was added in 100 mL of distilled water and was kept for 30 min in a water bath with intermittent shaking. The sample was allowed to cool at room temperature, and thereafter was filtered through Whatman filter paper No 1. The plant extract was kept in a refrigerator at 4 °C. The extract was used as a stabilizing and reducing agent for the preparation of AgNPs.
Synthesis of silver nanoparticles (AgNPs)
In a simple reaction procedure, following the protocol of Mahakham et al. [Citation22], 60 mL of leaf extract was added to 100 mL of 1 mmol/L AgNO3 aqueous solution, and was incubated in the dark (1 h) with gentle stirring. The solution turned to yellowish brown color upon heating for 5 min at 50 °C. The solution of AgNPs was purified by repeated centrifugation at 7000 rpm (Tomy MX-305, AR300-06) for 15 min followed by washing of the pellet four times with sterile distilled water. The water-suspended nanoparticles were lyophilized for 36 h followed by characterization for their composition and structure as described below.
Characterization of AgNPs
The physicochemical properties of synthesized AgNPs were characterized using several analytical techniques, including UV-visible spectroscopy (Nanodrop 8000 spectrophotometer), Fourier Transform Infrared Spectroscopy (Shimadzu), Transmission electron microscopy (TEM) (JEM-1400) and Zeta potential (Malvern Zetasizer, Malvern Instruments Ltd., Malvern, UK) at the Department of Chemistry, College of Science, King Saud University. The UV spectrum was read from 220 to 270 nm wavelength to check the synthesis and stability of AgNPs. FTIR was used for the confirmation of functional molecules on synthesized AgNPs using infrared light (wavenumber range, 4000–400 cm−1). The particle size was analyzed via TEM, and grids were set by sonicating the AgNPs solution for 4 min and keeping a few drops of AgNPs on the 300-mesh carbon-coated copper grid. Further, water was evaporated under a lamp operated at an accelerating voltage of 120 kV and images were captured by a side-mount camera. The sample of AgNPs was sonicated to measure the Zeta potential and to collect information about the charge on the nanoparticles.
Seed priming with AgNPs and determination of percentage germination
The seeds were collected from wild conditions, and stored in the seed bank, Department of Botany and Microbiology, King Saud University (Voucher No- KSUSB34). Petri dishes with filter paper were used for germination of the seeds. The sterilization of seeds followed to the procedure described in a previously published paper on E. macrochaetus [Citation21]. The mature seeds of E. macrochaetus were washed with tap water for 20 min followed by sterile double-distilled water to eliminate microbes and dust particles. The seeds were treated with 50% bleach solution for 5 min for surface sterilization, and thereafter were washed with sterile double-distilled water. Each Petri dish contained seeds of E. macrochaetus which were primed with different concentrations of AgNPs (40, 80, 160, 300 and 400 µmol/L; 100 mL each) and control with double distilled water for 24 h, respectively. The concentration of synthesized nanoparticles was compared to the concentration of silver nitrate that was used for synthesis. There were three biological replicates (three pots) in each treatment. The number of germinated seeds, plumule and radicle lengths were measured on the seventh day after sowing (DAS).
The following formula was used for calculation of germination rate:
Where T is the number of seeds (total) and G7 is the number of germinated seeds on the 7 DAS [Citation23,Citation24].
Determination of optimum salt concentration in germination study
Three concentrations of NaCl (40, 80 and 120 mmol/L) were selected based on a germination study and literature on other plant species. The NaCl concentrations were screened for pot experiments based on percent germination, plumule and radicle length. After sterilization, the seeds were kept on filter paper (size: 90 mm, Hermann, Paulsen) with 5 mL of NaCl of different concentrations. Germination rate (GR) was calculated on seventh DAS as described above.
Pot experiment for determination of physiological and biochemical parameters
The sterilized primed and unprimed seeds were sowed in pots (size: 12 cm × 10 cm) containing 1.2 kg of soil (peat moss and sand-3:1). The experiment was performed in а growth chamber with optimum conditions including 16 h/8 h day/night cycle, light intensity of 250 µmol/(m2 s) and 25 ± 1 °C temperature. For salinity stress treatment, the plants were irrigated (200 mL) at one-month intervals with different concentrations of NaCl (40 mmol/L, 80 mmol/L and 120 mmol/L) and received three treatments over 90 days, starting after 30 days of sowing.
Assessment of growth characteristics in pot experiment
The length of shoot and biomass (fresh and dry weight per plant) were measured by Mattler Toledo analytical balance after 120 days of sowing in all treated plants and compared to control plant groups. The plant materials were dried at 40 °C in an oven and measured.
Determination of biochemical parameters
Chlorophyll content
The amount of chlorophyll was determined using the method of Lichtenthaler [Citation25]. Small pieces of fresh leaves (0.1 g) were used to extract chlorophyll in 100% acetone. The samples were kept for incubation at room temperature overnight, and afterwards centrifuged at 5,000 rpm for 5 min (Tomy Mx-305, AR300-06). The absorbance was recorded at 645 nm and 663 nm using UV-vis spectrophotometer (UV-1800, Shimadzu).
The calculated chlorophyll content was expressed in μg/g FW.
Extraction of protein for determination of enzymate activities
The fresh leaves from each treatment were processed for the extraction of crude protein. The protein was isolated using phosphate buffer (pH 7.4) containing Triton X100, 1% of PVP and β- mercaptoethanol. The crude mixture was centrifuged at 5,000 g for 10 min and the supernatant was transferred in another fresh tube. The concentration of protein was measured at 280 nm using the Nanodrop 8000 spectrophotometer.
Measurement of enzyme activities
Glutathione reductase (EC 1.6.4.2)
The protocol of Rao [Citation26] was employed for the determination of Glutathione reductase (GR) activity in the fresh leaves. The activity of extracted enzyme from treated and untreated plants was measured at a wavelength 340 nm and calculated using the molar absorptivity constant of NADPH (6.2 L/(mol cm)). It was expressed as EU/(mg protein · min).
Ascorbate peroxidase (EC 1.11.1.11)
The Nakano and Asada [Citation27] method was used for Ascorbate peroxidase (APX) activity measurement. The enzyme was extracted from fresh leaves in extraction buffer (50 mmol/L phosphate buffer, pH-7.4). The fresh leaves were ground with liquid nitrogen in extraction buffer (pH-7.2), centrifuged and the supernatant was taken to assess the enzyme activity at 290 nm. The APX activity was calculated using extinction coefficient (ε) 2.8 L/(mol cm)and expressed as EU/(mg protein · min).
Proline determination
The method of Hanson et al. [Citation28] was employed for determination of proline content in fresh leaves of E. macrochetus. Sulfosalicylic acid (3% in water) was used to grind the leaf samples. Then, 2 mL of supernatant was taken in Eppendorf tubes to which 2 mL of acid ninhydrin was added and kept in boiling water for 1 h. The reaction was terminated by putting the samples on ice. Toluene (4 mL) was added in above step to separate chromatophore from the mixture. The absorbance of chromatophore was taken at 520 nm and proline content expressed in µg/g FW.
Nuclei extraction and staining
The genome size (2 C DNA content) was determined using the method of Sadhu et al. [Citation29]. The nuclei were isolated from fresh leaves of treated and untreated E. macrochaetus plants. The leaf samples (20 mg) were chopped with a sharp razor blade into 0.3–0.45 mm size pieces in cold nuclei isolation buffer (600 µL buffer). The extracted nuclei in buffer were passed through double nylon mesh (pore size-20 µm, procured from Macrokun (China)). The final volume of filtered nuclei was made to 800 µL. The nuclei were stained with 50 µg/mL of PI dye (propidium iodide) and incubated for 15 min at 4 °C. The same process was applied for the extraction of nuclei from standard plant Solanum lycopersicum obtained from Dr Jaroslav Dolezel, Czech Republic. Relative 2 C nuclear DNA content (genome size) of each sample was determined by comparing them with a plant DNA standard.
Flow cytometric analysis
The generated histogram from leaves of treated and untreated plants of E. macrochaetus was used for the determination of genome size and mitotic index. The 2 C DNA content (Nuclear DNA content) in all samples was determined according to Doležel et al. [Citation30]. Solanum lycopersicum (2 C = 1.96 pg) was used for calculation of genome size in treated and untreated samples of E. macrochaetus. The 2 C DNA content of samples was calculated according to the given formula:
Data analysis
One-way analysis of variance (ANOVA) was performed using SPSS (version 28) and significant differences among the means were evaluated using Duncan’s multiple test.
Results
Characterization of biogenic silver nanoparticles
Different techniques were used for the characterization of the biosynthesized silver nanoparticles as given in . The sharp peak of the UV-spectrum at 430 nm showed the synthesis of AgNPs. The biogenic AgNPs were checked with a UV-Vis spectrophotometer (NanoDrop 8000 spectrophotometer) in the range of 220–750 nm. For Transmission Electron Microscopy (TEM) study, a few drops of AgNPs were used to obtain images using high-resolution transmission electron microscopy with an operating voltage of 120 kV. The TEM analysis of AgNPs showed the size of nanoparticles ranged from 29.728 nm to 49.997 nm, which indicates the synthesis of small size nanoparticles. The synthesized AgNPs showed an average zeta potential of −14 mV with conductivity of 0.0823 mS/cm, which shows the stability of the nanoparticles. The Fourier Transmission Infrared (FTIR) spectrum was acquired in the range of 4000–400 cm−1. The absorption bands detected by FTIR at diverse wavelengths were 412.87 cm−1, 1641.55 cm−1, 2086.89 cm−1 and 3430.45 cm−1. They are associated with various functional groups available in the plant extract of Fagonia bruguieri. The absorption band detected at 3430.45 cm−1 was shown as a broad and intense peak; it resulted from stretching and vibration of O-H functional group. The peak at 1641.55 cm−1 was assigned for compounds having a functional group (C = C stretching) and the peak detected at 2086.89 cm−1 showed N = C=S stretching (isothiocyanate compounds). Thus, the biogenic AgNPs showed the presence of various functional groups in the leaf extract of F. bruguieri, which are responsible for stabilization, and lessening the charge of the synthesized AgNPs.
Figure 1. Characterization of biogenically synthesized AgNPs: (a) transmission electron microscopy (TEM); (b) Fourier transmission infrared (FTIR) spectroscopy (transmittance versus wavenumber); (c) UV-visible spectrum (absorbance versus wavelength; (d) zeta potential (total count versus apparent zeta potential).
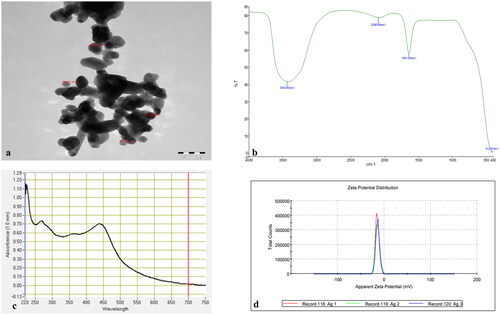
Seed germination study with biogenic AgNPs priming and NaCl treatment
A range of concentrations of biogenic silver nanoparticles (AgNPs) and salt were screened during the germination process of E. macrochaetus and further best concentrations were selected based on percent germination, plumule and radicle length for pot experiments. The percent seed germination was scored on the seventh day after sowing in Petri dishes. The primed seeds with biogenic AgNPs germinated faster than those under salinity stress only. Primed seeds with AgNPs (40 and 80 µmol/L) showed 100% germination like the control and thereafter the percent germination decreased in seeds primed with higher concentrations of AgNPs (160, 300 and 400 µmol/L AgNPs) (). Similarly, unprimed seeds showed fast and 100% germination at 40 mmol/L NaCl. However, the percent germination was decreased at 80 mmol/L NaCl (95%) and 120 mmol/L NaCl (70%) compared to the control, respectively.
Figure 2. Percentage seed germination of E. macrochaetus; C (Control, distilled water); Ag-40 (40 µmol/L AgNPs); Ag-80 (80 µmol/L AgNPs); Ag-160 (160 µmol/L AgNPs); Ag-300 (300 µmol/L AgNPs); Ag-400 (400 µmol/L AgNPs); NaCl-40 (40 mmol/L NaCl); NaCl-80 (80 mmol/L NaCl) and NaCl-120 (120 mmol/L NaCl). The percentage germination was determined on day 7 after sowing.
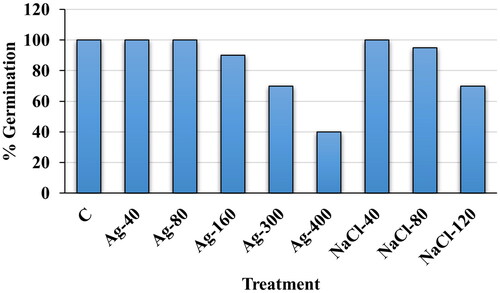
shows the variation in radicle and plumule length under different concentrations of AgNPs and NaCl. The highest variation was recorded in radicle lengths, 13.5 and 10.24 cm at 40 and 80 µmol/L of AgNPs priming than among all treatments as well as than the control (3.06 cm), respectively (). However, non-significant variation was observed in radicle length between treatment with AgNPs at 160 µmol/L and Ag-300 µmol/L, respectively. The plumule and radicle lengths decreased significantly under salinity stress (40, 80 and 120 mmol/L NaCl) compared to the control, and this occurred in a concentration-dependent manner. The radicle length was more affected than the plumule length under salinity stress ( and ). At a low concentration of NaCl (40 mmol/L), the thickness of plumule and radicle length was higher than in the control group. Moreover, faster germination was observed at 40 mmol/L NaCl than at the 80 and 120 mmol/L NaCl, respectively. Thus, a promoting effect of a low concentration of salt (40 mmol/L NaCl) was observed on plumule and radicle length compared to the control group. After screening of biogenic AgNPs and NaCl concentrations in the seed germination study, the seeds of E. macrochaetus primed with AgNPs (40 and 80 µmol/L) were selected and sowed in pots to observe the growth and development of the plants under conditions of salt treatment (40, 80 and 120 mmol/L NaCl) and without salt treatment.
Figure 3. Plumule and radicle lengths in germinated seeds of E. macrochaetus; (a) Control; (b) 40 µmol/L AgNPs; (c) 80 µmol/L AgNPs; (d) 160 µmol/L AgNPs; (e) 300 µmol/L AgNPs; (f) 400 µmol/L AgNPs; (g) 40 mmol/L NaCl; (h) 80 mmol/L NaCl; (i) 120 mmol/L NaCl. Note: Seed priming with AgNPs (24 h) and unprimed seeds germinated under salt solution (seven days). Pictures were taken on the seventh day after sowing.
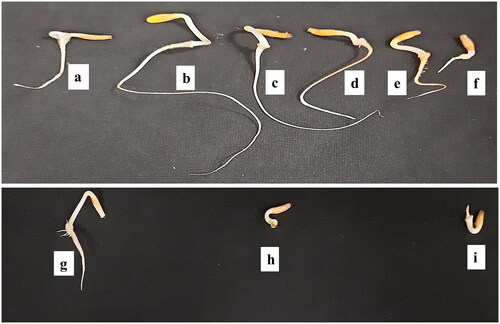
Figure 4. Effect of biogenic AgNPs and NaCl on plumule and radicle lengths in seed germination study. C (Control, distilled water); Ag-40 (40 µmol/L AgNPs); Ag-80 (80 µmol/L AgNPs); Ag-160 (160 µmol/L AgNPs); Ag-300 (300 µmol/L AgNPs); Ag-400 (400 µmol/L AgNPs); NaCl-40 (40 mmol/L NaCl); NaCl-80 (80 mmol/L NaCl) and NaCl-120 (120 mmol/L NaCl); data are means of three replicates (mean ± SD). Different letters on bar graph indicate significant differences (p < .05). Note: Measurements were made on the seventh day after sowing.
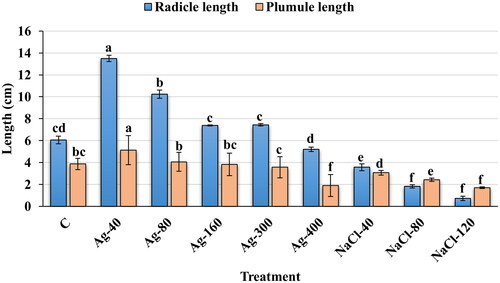
Effect of AgNPs and NaCl on E. macrochaetus phenotypic traits and biomass
The treatments (AgNPs and NaCl) and their interactions significantly and non-significantly influenced the morphological traits and biomass parameters. shows the growth of the E. macrochaetus plants under AgNPs and NaCl treatments. The fresh and dry weight of root, stem and leaves were improved with AgNPs as compared to the control as well as salt treatment alone. A non-significant variation was observed in biomass and shoot length with 40 and 80 µmol/L AgNPs except for the root fresh weight. However, all growth parameters including root, stem and leaves biomass, were decreased under salinity stress as compared to the control. The root fresh weight (5.0 g/plant) and dry weight (0.86 g/plant) were highest at 40 µmol/L AgNPs significantly than the control and salinity stress treatment (80 and 120 mmol/L NaCl). Similarly, the shoot length was recorded highest (8.81 cm) in the plants that received 40 µmol/L AgNPs priming as compared to the control and among all treatments. The biomass of E. macrochaetus was positively improved at 40 µmol/L AgNPs alone as well as with combined treatment (40, 80 and 120 mmol/L NaCl) than the 80 µmol/L AgNPs seed priming. A more positive effect of both concentrations of AgNPs (40 and 80 µmol/L) was observed on the fresh and dry weight of leaves than on the shoot and root (). The positive effect was observed in root fresh weight with 80 µmol/L AgNPs seed priming than the 40 µmol/L AgNPs when plants were exposed to 40 mmol/L NaCl, whereas the fresh leaves weight remained approximately the same at both concentrations of AgNPs under salinity stress (40 and 80 mmol/L NaCl). The large positive effect was not observed on stem fresh and dry weight in treatment T12p than the salinity treatment T6p. A non-significant variation was observed in shoot length with seed priming (80 µmol/L AgNPs) and plant exposed to 80 and 120 mmol/L NaCl stress.
Figure 5. Morphological characteristics of E. macrochaetus with AgNPs and NaCl treatment in pot experiment; (a) T1p (Control, distilled water); (b) T2p (40 µmol/L AgNPs); (c) T3p (80 µmol/L AgNPs); (d) T4p (40 mmol/L NaCl); (e) T5p (80 mmol/L NaCl); (f) T6p (120 mmol/L NaCl); (g) T7p (40 mmol/L NaCl + 40 µmol/L AgNPs); (h) T8p (80 mmol/L NaCl + 40 µmol/L AgNPs); (i) T9p (120 mmol/L NaCl + 40 µmol/L AgNPs); (j) T10p (40 mmol/L NaCl + 80 µmol/L AgNPs); (k) T11p (80 mmol/L NaCl + 80 µmol/L AgNPs); (l) T12p (120 mmol/L NaCl + 80 µmol/L AgNPs). Note: Treatment duration was 90 days and photographs were taken after 120 days of sowing.
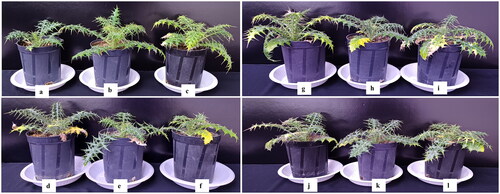
Table 1. Effect of biogenic AgNPs alone and in combined treatment (AgNPs + NaCl) on biomass of different parts of E. macrochaetus.
Assessment of biochemical parameters
Proline content
The osmotic stress induced by high salinity stress causes plants to synthesize compatible organic solutes such as proline in the cytosol. Increased proline content was observed in E. macrochaetus plants with or without treatment (AgNPs and NaCl). A non-significant result was observed in proline accumulation between the treatments (T2p and T3p) obtained on seed priming (with 40 and 80 µmol/L AgNPs) as compared to the control (). The proline content was significantly higher in the treatments under salinity stress than in the control group, and the increase occurred in a concentration dependent manner. However, the proline content remained significantly higher under combined treatment (NaCl + AgNPs) than in the control as well as in the treatments that individually applied salinity stress and AgNPs, respectively. The content of proline decreased in treatment T12p (340 µg/g FW), which was exposed to a high concentration of NaCl (120 mmol/L NaCl + 80 µmol/L AgNPs), as compared to the T10p treatment (402 µg/g FW) and T11p treatment (429 µg/g FW), respectively.
Table 2. Proline content, total chlorophyll and antioxidant enzyme activities (APX and GR) in treated (AgNPs and NaCl) and untreated plants of E. macrochaetus.
Total chlorophyll content
The E. macrochaetus plants obtained from primed seeds (40 µmol/L AgNPs) showed better growth under salinity stress, with enhanced level of chlorophyll comparable to the salinity treatment alone. These treated plants (priming with 40 µmol/L AgNPs) when exposed to salinity stress (40, 80 and 120 mmol/L NaCl) had improved chlorophyll content as compared to the plants under salinity stress alone. At both concentration of AgNPs (40 µmol/L and 80 µmol/L), the chlorophyll content was higher (304 and 214 µg/g FW) than the control. The chlorophyll content decreased in a concentration dependent manner in the plants exposed to salt treatment alone as compared to the control. However, in the plants obtained after seed priming, when exposed to salinity stress (40, 80 and 120 mmol/L), the chlorophyll content improved compared to that in the plants under salinity stress alone. The AgNP-treated plants (40 µmol/L AgNPs), when exposed to 40 mmol/L NaCl treatment, showed a significant enhancement in chlorophyll content (165 µg/g FW) (). Thus, a low concentration of AgNPs applied to seeds ameliorated the adverse effects of NaCl by optimizing the production of photosynthetic pigments in E. macrochaetus.
Antioxidant enzyme activities
The activities of the two antioxidant enzymes, APX and GR, showed non-significant difference in treatment T2p (40 µmol/L AgNPs) as compared to the control plants. However, the enzyme activities increased in the leaves of E. macrochaetus under salinity stress (40, 80 and 120 mmol/L) in a concentration-dependent manner. The activities of APX and GR were highest (1.39 and 0.48 U/mg protein/min) in the T9p treatment (120 mmol/L NaCl + 40 µmol/L AgNPs), respectively (). The activities of GR and APX were found to be higher in plants (T6p, T8p and T9p) compared to those in the plants under salinity stress treatment alone and the control. However, the activities of GR and APX sharply decreased in T12p plants (0.92 and 0.28 U/mg protein/min) compared to the respective salt treatment alone and the control.
Genotoxicity assessment
The fluorescence intensity at the G0/G1 phase was used to measure the genome size in treated and untreated E. macrochaetus plants as previously described [Citation21]. The genome size (2 C DNA content) was measured in triplicate in leaf samples from E. macrochaetus plants subjected to individual treatment and combined treatment (AgNPs + NaCl) along with the control group. The FCM analysis of nuclei extracted from E. macrochaetus showed clear histograms with defined G0/G1 peaks for each treatment as well as control group (). The low number of nuclei were extracted from the treatment T2p, T4p, T5p, T7p and T10p, respectively which might be due to the high content of cytosolic compounds (). The genome size ranged in treatments (AgNPs and NaCl) from 2.0 pg to 2.359 pg compared to the control, which had 2.252 pg (). A minor difference in genome size (2 C DNA content) was observed in treated plants as compared to the control. A reduction in the mitotic index in some treatments, including Tp5, Tp6 and Tp12, showed genotoxicity as compared to the control.
Figure 6. Histogram display of DNA content from leaves of E. macrochaetus measured by flow cytometry. Figures and their corresponding treatments; (a) T1p (Control, distilled water); (b) T2p (40 µmol/L AgNPs); (c) T3p (80 µmol/L AgNPs); (d) T4p (40 mmol/L NaCl); (e) T5p (80 mmol/L NaCl); (f) T6p (120 mmol/L NaCl); (g) T7p (40 mmol/L NaCl + 40 µmol/L AgNPs); (h) T8p (80 mmol/L NaCl + 40 µmol/L AgNPs); (i) T9p (120 mmol/L NaCl + 40 µmol/L AgNPs); (j) T10p (40 mmol/L NaCl + 80 µmol/L AgNPs); (k) T11p (80 mmol/L NaCl + 80 µmol/L AgNPs); (l) T12p (120 mmol/L NaCl + 80 µmol/L AgNPs).
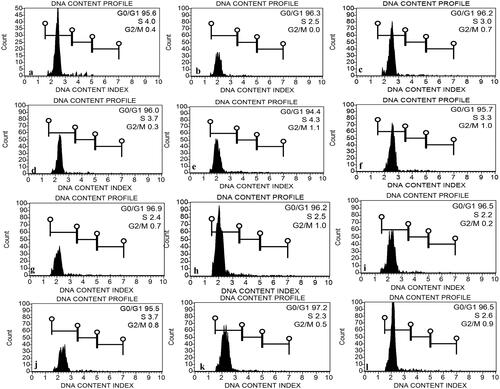
Table 3. Assessment of genotoxicity in E. macrochaetus exposed to NaCl stress and biogenic AgNPs.
Discussion
Abiotic stresses have adverse effects on plant growth and development which modify various physiological parameters. Salinity stress generally produces both osmotic as well as ionic stress in plants, resulting in accumulation or reduction of particular secondary metabolites in plants [Citation31]. To mitigate the adverse effects of abiotic environmental stress on plants, innovative technologies, such as nanotechnology, have been explored. Applying nanotechnology in modern agriculture can also aid for better water retention by the plants, ensure food security, prevent plant diseases, lessen environmental pollution, and augment sustainability. Different types of nanoparticles (NPs) have been applied to plants in several modes for evaluation of their activities. However, various types of NPs produce different effects, and this depends on their shape, size, surface properties and concentration, which specifically controls the cellular mechanisms involved in the alleviation of salt stress. The application of engineered NPs to ameliorate the toxicity caused by salt stress would be a promising strategy. Soil salinity prevents seed germination due to the low osmotic potential around the seeds, which inhibits the water uptake [Citation32,Citation33]. The salt tolerance has been improved using nanoparticles and proposed as a promising substitute in plant defense against salinity stress because of the low-cost NPs synthesis [Citation34,Citation35]. The synthesis of nanoparticles using plant extracts provides an excellent way for fabrication of nanoparticles that are not only good for industries, but can also be manufactured on a large scale.
In the present study, the AgNPs synthesized using the extract of F. bruguieri showed stimulatory effects on the germination of E. macrochaetus. The seeds of E. macrochaetus primed with different concentrations of biogenic AgNPs showed varied levels of percent germination. However, low concentrations of AgNPs (40 and 80 µmol/L) showed a positive effect on seed germination with enhanced plumule and radicle length as compared to the control (, , and ). Earlier findings have shown that the effect of AgNPs on germination of seeds and seedling growth can be either positive or negative, depending on the properties, size, concentration of the nanoparticles, the test plant and the application method [Citation36,Citation37]. In Pennisetum glaucum, there was a positive effect of pre-sowing treatment of seeds with AgNPs [Citation38]. A negative effect on the percent germination, plumule and radicle length was observed in E. macrochaetus under different concentrations of salt. The low concentration of salt (40 mmol/L) showed a less negative effect on E. macrochaetus (seed germination, plumule and radicle length) than the high concentrations of salt (80 and 120 mmol/L), which showed more pronounced negative effect. The different concentrations of salt stress affected the germination and seedling growth potential of Cucurbita maxima, leading to most severe effects at high stress levels for all the traits under study [Citation39]. The plant sensitivity to salinity differs among growth stages [Citation40], while during the germination process, the stress effects are intensified due to the decreased seed water uptake, which limits turgescence and seed imbibition [Citation41].
Nanotechnology is a pioneering and effective means of technology which has the potential to improve crop quality and yield by modifying their physiological and biochemical parameters. Here, the effect of seed priming with biogenic AgNPs on the physiological, biochemical and morphological parameters of E. macrochaetus were studied under salinity stress in a pot experiment. A low concentration of biogenic AgNPs (40 µmol/L) improved the shoot length in E. macrochaetus, in agreement with the report of Soliman et al. [Citation42] on Zea mays, Trigonella foenum-graecum and Alium cepa. The present study confirmed the positive effects of AgNPs priming on the growth parameters of E. macrochaetus, including shoot length, fresh and dry weight of shoot, root and leaves under salinity stress. Moreover, AgNPs considerably augmented the fresh and dry weight in E. macrochaetus, whereas no signs of necrosis and chlorosis were observed at low concentration of AgNPs (40 µmol/L).
AgNPs, can act as a stress alleviator in the plants and decrease the adverse effects of abiotic stress by triggering plant defense mechanisms to scavenge the ROS and reduce the phytotoxicity [Citation43]. The stimulatory effects of biosynthesized AgNPs on seedling growth and their biomass accumulation have been reported in Trigonella foenum-graceum [Citation44], Oriental lilies [Citation45] and Oryza sativa [Citation46]. Likewise, positive effects of low-concentrated solution of AgNP on seedling development were described in Pisum sativum [Citation47]. Application of low concentrations of AgNPs enhanced the biomass and number of roots in tomato cultivars [Citation48].
The amino acid proline plays a significant role in maintaining the metabolic balance and growth of plants under abiotic stress. Different studies indicate a positive correlation between proline augmentation and tolerance of plants to different abiotic stresses [Citation49]. The AgNPs and NaCl interactions had a significant impact on proline accumulation in the leaves of E. macrochaetus. The accumulation of proline in plants subjected to combined treatment (NaCl + AgNPs) in E. macrochaetus was higher than that in the plants subjected to salinity stress alone, which allowed the growth of the plants, suggesting such application could improve plant tolerance to NaCl stress. Since, proline is an inert solute, it does not affect the regular metabolic processes in plant cells. Moreover, proline can act as a metal chelator, which stimulates plant tolerance to toxic metal stress [Citation16,Citation50]. Different concentrations of biogenic AgNPs enhanced the accumulation of proline, chlorophyll content, total protein in Maerua oblongifolia in tissue culture [Citation51].
The physiological changes occurred in plant under salinity stress exacerbate overproduction of reactive oxygen species (ROS) that affects normal cellular metabolism and consequences in oxidative damage by oxidizing lipids, proteins, other cellular macromolecules and DNA [Citation52,Citation53]. Salinity stress can cause oxidative burst in plants, which might lead to the production of reactive oxygen species (ROS) and hence, reduce the plant growth. However, the improved antioxidant enzymes could be associated with the active involvement of nanoparticles to decrease the toxic effect of stress as observed in our present study on E. macrochaetus. The antioxidant enzyme activities, including APX and GR, increased in the leaves of E. macrochaetus under salt stress. Moreover, the activities of APX and GR were increased in combined treatment (AgNPs + NaCl) in plants primed at both concentrations of AgNPs (40 µmol/L and 80 µmol/L) and exposed to salinity stress (40, 80 and 120 mmol/L). With AgNPs seed priming, plants showed higher chlorophyll content, which was associated with enhanced synthesis of protein, as compared to the control as well as to salinity stressed plants alone. The high accumulation of enzymes APX and GR in treatment T9p (120 mmol/L NaCl +40 µmol/L AgNPs) indicated more pronounced removal of ROS generated under salinity stress. This response might be due to the promoting effect of AgNPs, which induce the level of enzymatic antioxidants that scavenge the free radicals (ROS) which interrupt the cellular functions of the cell [Citation11,Citation16,Citation54] and thus, protect the plants from reactive oxygen species (ROS). The application of low salt concentration on Amaranthus tricolor enhanced the antioxidant activity, protein, β-carotene and ascorbic acid in the leaves [Citation53].
Photosynthetic pigments are recognized as biomarkers for the physiological status of plants. Chlorophyll is a key component of chloroplasts and improves the photosynthesis; however, salinity is associated with a decrease in the chlorophyll amount, which directly correlates to a reduction in the photosynthetic rate [Citation55,Citation56]. Our results in E. macrochaetus confirmed that salinity stress decreased the chlorophyll content, which ultimately negatively affected the various growth parameters. However, seeds primed with AgNPs had an improved chlorophyll content in E. macrochaetus under salinity stress and had higher chlorophyll content as compared to control group as well as salinity treatment alone (). The seed priming with silver NPs enhanced the germination, yield, soluble sugar in Citrullus lanatus [Citation57]. Similarly, seed priming of rice with silver nanoparticles enhanced seed germination, α-amylase activity and soluble sugar for supporting seedlings growth [Citation22]. Foliar application of AgNPs improved the growth of fenugreek plants, as evaluated on the basis of various parameters including number of leaves/plant, shoot length, shoot dry weight, and increased various biochemical parameters such as photosynthetic pigments (carotenoids, chlorophyll a and chlorophyll b) and polyphenolic content [Citation58]. Our results confirmed that high salinity stress (80 and 120 mmol/L NaCl) reduced the chlorophyll content, which eventually decreased the growth parameters of E. macrochaetus including shoot length (). The ameliorating effect of AgNPs has been observed on various plant species including Trigonella foenumgraecum [Citation59], Triticum aestivum [Citation60] and Pennisetum glaucum [Citation61] exposed to salinity stress.
The abiotic and biotic stresses induce oxidative and genotoxic stress, which leads to the formation of various lesions in the DNA double helix [Citation62]. Unrestored damage in the DNA molecule in turn lead to genomic variability, and thus, affect plant growth and yield. The genotoxicity was measured in terms of mitotic index by assessing genome size of E. macrochaetus plants treated with NaCl alone or in combined treatment (AgNPs and NaCl). The calculated mitotic index from the 2 C and 4 C value of the genome size of E. macrochaetus showed some genotoxic effects under high salinity stress (120 mmol/L) as well as in combined treatment. Sihi et al. [Citation63] have observed DNA strand breaks in rice under salinity stress due to the production of reactive oxygen species (ROS). Application of high concentration of AgNPs caused cytotoxicity as well as genotoxicity in Allium cepa [Citation64]. The estimated genome size variation in E. macrochetus might be affected by varied content of secondary metabolites under the treatment of AgNPs and salinity stress, as these hinder the binding of propidium iodide dye (PI) to the DNA, and further affect fluorescent intensity. For example, compounds present in Coffea spp. and anthocyanin in Euphorbia pulcherrim hinder in the PI binding to the DNA [Citation65,Citation66].
Overall, the plants raised by seed priming with AgNPs in pot experiments showed a considerable positive effect than the non-primed plants under salinity stress. Since seed priming enhanced the salinity tolerance of E. macrochetus, such tolerant plants could be used to raise more populations in natural habitats, as low population numbers of this species are found in Saudi Arabia.
Conclusions
Phytosynthesis of nanoparticles (NPs) is a cost-effective and eco-friendly method, and they might act as novel stimulants, if applied in low concentrations. Biogenic AgNPs, positively influenced the percent germination and morphological traits alone as well as in combined treatment (AgNPs + NaCl). Salinity stress negatively affected the morphological traits of E. macrochaetus and biomass in the present study. The seed priming with biogenic AgNPs was associated with improve growth parameters and enhanced antioxidant defense system of the plants, probably by reducing the damage caused by salt-induced oxidative stress. Thus, application of biogenic AgNPs in seed priming techniques might be an alternative approach for improving plant growth parameters under salinity stress by improving the biochemical and physiological responses.
Author contributions
Conceptualization, S.K.; methodology, M.N and. S.K.; software and analysis, M.T. and M.N.; investigation, S.K.; results, M.N and. S.K.; analysis, H.O.S and. A.M.S.; writing—original draft preparation, S.K.; writing—review and editing, S.K. and M.N.; investigation, N. S. A.; Funding acquisition, F.A.-Q., and A.A.H.
Disclosure statement
No potential conflict of interest was reported by the author(s).
Data availability statement
The data supporting the findings of the present study are available in this article and are accessible from corresponding author upon reasonable request.
Additional information
Funding
References
- Kumar S, Li G, Yang J, et al. Effect of salt stress on growth, physiological parameters, and ionic concentration of water dropwort (Oenanthe javanica) cultivars. Front Plant Sci. 2021;12:1. doi: 10.3389/fpls.2021.660409.
- Islam F, Wang J, Farooq MA, et al. Rice responses and tolerance to salt stress: deciphering the physiological and molecular mechanisms of salinity adaptation, Mirza Hasanuzzaman, Masayuki Fujita, Kamrun Nahar, Jiban Krishna Biswas, Woodhead Publishing, U.K. In: Advances in rice research for abiotic stress tolerance. Elsevier; 2019. p. 791–15.
- Ali Q, Daud M, Haider MZ, et al. Seed priming by sodium nitroprusside improves salt tolerance in wheat (Triticum aestivum L.) by enhancing physiological and biochemical parameters. Plant Physiol Biochem. 2017;119:50–58. doi: 10.1016/j.plaphy.2017.08.010.
- Parihar P, Singh S, Singh R, et al. Effect of salinity stress on plants and its tolerance strategies: a review. Environ Sci Pollut Res Int. 2015;22(6):4056–4075. doi: 10.1007/s11356-014-3739-1.
- Li Z, Zhou T, Zhu K, et al. Effects of salt stress on grain yield and quality parameters in rice cultivars with differing salt tolerance. Plants. 2023;12:3243. doi: 10.3390/plants12183243.
- Angon PB, Tahjib-Ul-Arif M, Samin SI, et al. How do plants respond to combined drought and salinity stress?—a systematic review. Plants. 2022;11:2884. doi: 10.3390/plants11212884.
- Bhattarai S, Biswas D, Fu Y-B, et al. Morphological, physiological, and genetic responses to salt stress in alfalfa: a review. Agronomy. 2020;10:577. doi: 10.3390/agronomy10040577.
- Kamran M, Parveen A, Ahmar S, et al. An overview of hazardous impacts of soil salinity in crops, tolerance mechanisms, and amelioration through selenium supplementation. Int J Mol Sci. 2019;21(1):148. doi: 10.3390/ijms21010148.
- Zulfiqar F, Ashraf M. Nanoparticles potentially mediate salt stress tolerance in plants. Plant Physiol Biochem. 2021;160:257–268. doi: 10.1016/j.plaphy.2021.01.028.
- Giraldo JP, Landry MP, Faltermeier SM, et al. Plant nanobionics approach to augment photosynthesis and biochemical sensing. Nat Mater. 2014;13(4):400–408. doi: 10.1038/nmat3890.
- Yan A, Chen Z. Impacts of silver nanoparticles on plants: a focus on the phytotoxicity and underlying mechanism. Int J Mol Sci. 2019;20(5):1003. doi: 10.3390/ijms20051003.
- Steinitz B, Bilavendran AD. Thiosulfate stimulates growth and alleviates silver and copper toxicity in tomato root cultures. Plant Cell Tissue Organ Cult. 2011;107:355–363. doi: 10.1007/s11240-011-9987-6.
- Monica RC, Cremonini R. Nanoparticles and higher plants. Caryologia. 2009;62:161–165. doi: 10.1080/00087114.2004.10589681.
- Azeez L, Adejumo AL, Lateef A, et al. Zero-valent silver nanoparticles attenuate Cd and Pb toxicities on Moringa oleifera via immobilization and induction of phytochemicals. Plant Physiol Biochem. 2019;139:283–292. doi: 10.1016/j.plaphy.2019.03.030.
- Sharma P, Bhatt D, Zaidi M, et al. Silver nanoparticle-mediated enhancement in growth and antioxidant status of Brassica juncea. Appl Biochem Biotechnol. 2012;167(8):2225–2233. doi: 10.1007/s12010-012-9759-8.
- Tejada-Alvarado JJ, Meléndez-Mori JB, Ayala-Tocto RY, et al. Influence of silver nanoparticles on photosynthetic pigment content and mineral uptake in pineapple seedlings grown in vitro under aluminum stress. Agronomy. 2023;13:1186. doi: 10.3390/agronomy13051186.
- Funk VA, Bayer RJ, Keeley S, et al. Everywhere but Antarctica: using a supertree to understand the diversity and distribution of the Compositae. Biol Skr. 2005;55:343–373.
- Abegaz BM. Polyacetylenic thiophenes and terpenoids from the roots of Echinops pappii. Phytochemistry. 1991;30:879–881. doi: 10.1016/0031-9422(91)85271-Z.
- Zamzami TA, Abdallah HM, Shehata IA, et al. Macrochaetosides a and B, new rare sesquiterpene glycosides from Echinops macrochaetus and their cytotoxic activity. Phytochem Lett. 2019;30:88–92. doi: 10.1016/j.phytol.2019.01.025.
- Arroo R, Jacobs J, Van Gestel J, et al. Regulation of thiophene biosynthesis by sulphate in roots of marigolds. New Phytol. 1997;135:175–181. doi: 10.1046/j.1469-8137.1997.00637.x.
- Khan S, Al-Qurainy F, Al-Hashimi A, et al. Effect of green synthesized ZnO-NPs on growth, antioxidant system response and bioactive compound accumulation in Echinops macrochaetus, a potential medicinal plant, and assessment of genome size (2C DNA content). Plants. 2023;12:1669. doi: 10.3390/plants12081669.
- Mahakham W, Sarmah AK, Maensiri S, et al. Nanopriming technology for enhancing germination and starch metabolism of aged rice seeds using phytosynthesized silver nanoparticles. Sci Rep. 2017;7(1):8263. doi: 10.1038/s41598-017-08669-5.
- Alvarado AD, Bradford KJ, Hewitt JD. Osmotic priming of tomato seeds: effects on germination, field emergence, seedling growth, and fruit yield. J Am Soc Hortic Sci. 1987;112:427–432. doi: 10.21273/JASHS.112.3.427.
- Ruan S, Xue Q, Tylkowska K. The influence of priming on germination of rice (Oryza sativa L.) seeds and seedling emergence and performance in flooded soil. Seed Sci Technol. 2002;30:61–67.
- Lichtenthaler HK. [34] chlorophylls and carotenoids: pigments of photosynthetic biomembranes. In: Methods in enzymology. Elsevier; 1987. Vol. 148, p. 350–382.
- Rao M. Cellular detoxifying mechanisms determine the age dependent injury in tropical trees exposed to SO2. J Plant Physiol. 1992;140:733–740. doi: 10.1016/S0176-1617(11)81031-X.
- Nakano Y, Asada K. Hydrogen peroxide is scavenged by ascorbate-specific peroxidase in spinach chloroplasts. Plant Cell Physiol. 1981;22:867–880.
- Hanson A, Nelsen C, Pedersen A, et al. Capacity for proline accumulation during water stress in barley and its implications for breeding for drought resistance 1. Crop Sci. 1979;19:489–493. doi: 10.2135/cropsci1979.0011183X001900040015x.
- Sadhu A, Bhadra S, Bandyopadhyay M. Novel nuclei isolation buffer for flow cytometric genome size estimation of Zingiberaceae: a comparison with common isolation buffers. Ann Bot. 2016;118(6):1057–1070. doi: 10.1093/aob/mcw173.
- Doležel J, Binarová P, Lucretti S. Analysis of nuclear DNA content in plant cells by flow cytometry. Biol Plant. 1989;31:113–120.
- Mahajan S, Tuteja N. Cold, salinity and drought stresses: an overview. Arch Biochem Biophys. 2005;444(2):139–158. doi: 10.1016/j.abb.2005.10.018.
- Taha RS, Seleiman MF, Shami A, et al. Integrated application of selenium and silicon enhances growth and anatomical structure, antioxidant defense system and yield of wheat grown in salt-stressed soil. Plants. 2021;10:1040. doi: 10.3390/plants10061040.
- Tavakkoli E, Rengasamy P, McDonald GK. High concentrations of Na + and Cl–ions in soil solution have simultaneous detrimental effects on growth of faba bean under salinity stress. J Exp Bot. 2010;61(15):4449–4459. doi: 10.1093/jxb/erq251.
- Ross A, Muñoz M, Rotstein BH, et al. A low cost and open access system for rapid synthesis of large volumes of gold and silver nanoparticles. Sci Rep. 2021;11(1):5420. doi: 10.1038/s41598-021-84896-1.
- Kajbafvala A, Li M, Bahmanpour H, et al. Nano/microstructured materials: rapid, low-cost, and eco-friendly synthesis methods. J Nanoparticles. 2013;2013. doi: 10.1155/2013/530170.
- Feregrino-Perez AA, Magaña-López E, Guzmán C, et al. A general overview of the benefits and possible negative effects of the nanotechnology in horticulture. Sci Hortic. 2018;238:126–137. doi: 10.1016/j.scienta.2018.03.060.
- Prażak R, Święciło A, Krzepiłko A, et al. Impact of Ag nanoparticles on seed germination and seedling growth of green beans in normal and chill temperatures. Agriculture. 2020;10:312. doi: 10.3390/agriculture10080312.
- Parveen A, Rao S. Effect of nanosilver on seed germination and seedling growth in Pennisetum glaucum. J Cluster Sci. 2015;26:693–701. doi: 10.1007/s10876-014-0728-y.
- Tarchoun N, Saadaoui W, Mezghani N, et al. The effects of salt stress on germination, seedling growth and biochemical responses of Tunisian squash (Cucurbita maxima Duchesne) germplasm. Plants. 2022;11:800. doi: 10.3390/plants11060800.
- Acosta-Motos JR, Ortuño MF, Bernal-Vicente A, et al. Plant responses to salt stress: adaptive mechanisms. Agronomy. 2017;7:18. doi: 10.3390/agronomy7010018.
- Golbashy M, Ebrahimi M. Effects of drought stress on germination indices of corn hybrids (Zea mays L.). Electron J Plant Breed. 2012;3:664–670.
- Soliman M, Qari SH, Abu-Elsaoud A, et al. Rapid green synthesis of silver nanoparticles from blue gum augment growth and performance of maize, fenugreek, and onion by modulating plants cellular antioxidant machinery and genes expression. Acta Physiol Plant. 2020;42:1–16. doi: 10.1007/s11738-020-03131-y.
- Abasi F, Raja NI, Mashwani ZUR, et al. Biogenic silver nanoparticles as a stress alleviator in plants: a mechanistic overview. Molecules. 2022;27(11):3378. doi: 10.3390/molecules27113378.
- Jasim B, Thomas R, Mathew J, et al. Plant growth and diosgenin enhancement effect of silver nanoparticles in Fenugreek (Trigonella foenum-graecum L.). Saudi Pharm J. 2017;25(3):443–447. doi: 10.1016/j.jsps.2016.09.012.
- Salachna P, Byczyńska A, Zawadzińska A, et al. Stimulatory effect of silver nanoparticles on the growth and flowering of potted oriental lilies. Agronomy. 2019;9:610. doi: 10.3390/agronomy9100610.
- Gupta SD, Agarwal A, Pradhan S. Phytostimulatory effect of silver nanoparticles (AgNPs) on rice seedling growth: an insight from antioxidative enzyme activities and gene expression patterns. Ecotoxicol Environ Saf. 2018;161:624–633. doi: 10.1016/j.ecoenv.2018.06.023.
- Barabanov P, Gerasimov A, Blinov A, et al. Influence of nanosilver on the efficiency of Pisum sativum crops germination. Ecotoxicol Environ Saf. 2018;147:715–719. doi: 10.1016/j.ecoenv.2017.09.024.
- Guzmán-Báez GA, Trejo-Téllez LI, Ramírez-Olvera SM, et al. Silver nanoparticles increase nitrogen, phosphorus, and potassium concentrations in leaves and stimulate root length and number of roots in tomato seedlings in a hormetic manner. Dose Response. 2021;19(4):15593258211044576. doi: 10.1177/15593258211044576.
- Dar MI, Naikoo MI, Rehman F, et al. Proline accumulation in plants: roles in stress tolerance and plant development. Osmolytes and Plants Acclimation to Changing Environment: emerging Omics Technologies. 2016;155–166.
- Meena M, Divyanshu K, Kumar S, et al. Regulation of L-proline biosynthesis, signal transduction, transport, accumulation and its vital role in plants during variable environmental conditions. Heliyon. 2019;5(12):e02952. doi: 10.1016/j.heliyon.2019.e02952.
- Shaikhaldein HO, Al-Qurainy F, Nadeem M, et al. Biosynthesis and characterization of silver nanoparticles using Ochradenus arabicus and their physiological effect on Maerua oblongifolia raised in vitro. Sci Rep. 2020;10(1):17569. doi: 10.1038/s41598-020-74675-9.
- Gill SS, Tuteja N. Reactive oxygen species and antioxidant machinery in abiotic stress tolerance in crop plants. Plant Physiol Biochem. 2010;48(12):909–930. doi: 10.1016/j.plaphy.2010.08.016.
- Sarker U, Islam MT, Oba S. Salinity stress accelerates nutrients, dietary fiber, minerals, phytochemicals and antioxidant activity in Amaranthus tricolor leaves. PLoS One. 2018;13(11):e0206388. doi: 10.1371/journal.pone.0206388.
- Haghighi Pak Z, Karimi N, Abbaspour H. Effects of silver nanoparticle exposure on growth, physiological and biochemical parameters of Dracocephalum moldavica L. Iran J Plant Physiol. 2017;7:2173–2183.
- Munns R, Tester M. Mechanisms of salinity tolerance. Annu Rev Plant Biol. 2008;59:651–681. doi: 10.1146/annurev.arplant.59.032607.092911.
- Khan I, Awan SA, Raza MA, et al. Silver nanoparticles improved the plant growth and reduced the sodium and chlorine accumulation in pearl millet: a life cycle study. Environ Sci Pollut Res Int. 2021;28(11):13712–13724. doi: 10.1007/s11356-020-11612-3.
- Acharya P, Jayaprakasha GK, Crosby KM, et al. Nanoparticle-mediated seed priming improves germination, growth, yield, and quality of watermelons (Citrullus lanatus) at multi-locations in Texas. Sci Rep. 2020;10(1):5037. doi: 10.1038/s41598-020-61696-7.
- Sadak MS. Impact of silver nanoparticles on plant growth, some biochemical aspects, and yield of fenugreek plant (Trigonella foenum-graecum). Bull Natl Res Cent. 2019;43:1–6. doi: 10.1186/s42269-019-0077-y.
- Hojjat SS, Kamyab M. The effect of silver nanoparticle on Fenugreek seed germination under salinity levels. Russ Agric Sci. 2017;43:61–65. doi: 10.3103/S1068367417010189.
- Mohamed AKS, Qayyum MF, Abdel-Hadi AM, et al. Interactive effect of salinity and silver nanoparticles on photosynthetic and biochemical parameters of wheat. Arch Agron Soil Sci. 2017;63:1736–1747. doi: 10.1080/03650340.2017.1300256.
- Khan I, Raza MA, Awan SA, et al. Amelioration of salt induced toxicity in pearl millet by seed priming with silver nanoparticles (AgNPs): the oxidative damage, antioxidant enzymes and ions uptake are major determinants of salt tolerant capacity. Plant Physiol Biochem. 2020;156:221–232. doi: 10.1016/j.plaphy.2020.09.018.
- Dutta S, Mitra M, Agarwal P, et al. Oxidative and genotoxic damages in plants in response to heavy metal stress and maintenance of genome stability. Plant Signal Behav. 2018;13(8):e1460048. doi: 10.1080/15592324.2018.1460048.
- Sihi S, Bakshi S, Maiti S, et al. Analysis of DNA polymerase λ activity and gene expression in response to salt and drought stress in Oryza sativa Indica rice cultivars. J Plant Growth Regul. 2022;41:1499–1515. doi: 10.1007/s00344-021-10390-7.
- Heikal YM, Şuţan NA, Rizwan M, et al. Green synthesized silver nanoparticles induced cytogenotoxic and genotoxic changes in Allium cepa L. varies with nanoparticles doses and duration of exposure. Chemosphere. 2020;243:125430. doi: 10.1016/j.chemosphere.2019.125430.
- Noirot M, Barre P, Duperray C, et al. Effects of caffeine and chlorogenic acid on propidium iodide accessibility to DNA: consequences on genome size evaluation in coffee tree. Ann Bot. 2003;92(2):259–264. doi: 10.1093/aob/mcg139.
- Bennett MD, Price HJ, Johnston JS. Anthocyanin inhibits propidium iodide DNA fluorescence in Euphorbia pulcherrima: implications for genome size variation and flow cytometry. Ann Bot. 2008;101(6):777–790. doi: 10.1093/aob/mcm303.