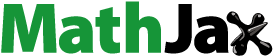
Abstract
Size is one of the important factors for antigen carriers because it influences cellular interaction, pharmacokinetics, immune cell activation and the types of immune responses. We developed pH-sensitive polysaccharide derivative-modified liposomes as antigen carriers for induction of antigen-specific immune responses. For an earlier study, the size of these liposomes was controlled to within 100–200 nm considering the suitable size for endocytosis. However, since most antigen-presenting cells can engulf larger particles, the proper liposome size and its effect on the induction of cellular versus humoral immune responses remains unclear. Here, we examined the effects of polysaccharide derivative-modified liposome size on immune responses. Liposome size was controlled by using an extrusion method by passing liposomes through a polycarbonate membrane of different pore sizes. Small liposomes encapsulating model antigenic protein suppressed antigen-expressing tumour growth effectively and increased the IgG2a/IgG1 ratio early after liposome administration, suggesting that Th1 response was mainly induced. Large liposomes increased antigen-specific IgG1 and IgG2a production for 56 days compared with small liposomes. These results suggest that small liposomes modified with pH-responsive polysaccharide derivatives could be effective for cancer immunotherapy whereas large liposomes could be suitable for induction of antibody production towards vaccination.
Introduction
The outbreak of SARS-CoV-2 virus in 2019 caused a global pandemic of Coronavirus Disease 2019 (COVID-19), prompting development of vaccines of various types against the virus [Citation1, Citation2]. Vaccines against viral infection should activate both humoral immunity and cellular immunity to induce prophylactic effect and to eliminate pathogens [Citation2, Citation3]. Antigen-presenting cells (APCs) present antigens to CD4+ T cells and induce the differentiation of CD4+ T cells into Th1 cells and Th2 cells [Citation4, Citation5]. Th2 cells mainly activate B cells, whereas Th1 cells cause the activation of B cells, macrophages and cytotoxic T cells (CTLs), resulting in the induction of cellular immunity [Citation6]. Induction of cellular immunity is important for immunotherapy because CTLs are associated with favorable prognoses for various cancers and for the alleviation of infection [Citation7–9].
For the activation of humoral and cellular immunity, liposomes are useful as antigen carriers because both hydrophilic and hydrophobic antigens, related molecules can be encapsulated within the aqueous inner space or into the lipid bilayer [Citation10]. In addition, liposomes can be functionalized with modification by various materials such as antibodies, peptides, carbohydrates and proteins onto surfaces either by adsorption or by chemical conjugation [Citation11]. The formulation parameters of the particles themselves (e.g. lipid composition, size and surface charge) affect the regulation of the immune response [Citation12]. Especially, liposome size plays an important role in immune induction because the particle size affects cellular uptake by APCs and the type of induced immune response [Citation13–17]. Therefore, identification of the optimal liposome size is important when using liposome formulation for immunotherapy.
We have developed pH-responsive polysaccharide-modified liposomes of 100–200 nm [Citation18–22]. Polysaccharides were conjugated with 3-methylglutarylated (MGlu) groups as pH-responsive segments [Citation18–22]. Protonation of a carboxy group in an MGlu group at weakly acidic pH gives the MGlu group hydrophobicity. This hydrophobicity induced the destabilization of liposome membranes and/or endosome membranes. MGlu groups in polysaccharide derivatives played a role as functional groups with adjuvant activity. Curdlan, Aquaβ (β-1,3-1,6-glucan derived from Aureobasidium pullulans), and chondroitin sulfate with MGlu groups promoted pro-inflammatory cytokine secretion from APCs more than polysaccharides without MGlu groups did [Citation18–20]. Among them, Aquaβ is useful as a backbone of pH-responsive polysaccharide derivatives for induction of immune response because MGlu-Aquaβ-modified liposomes induced the increase in the percentage of CD8+ T cells effectively and strong antitumour effect without adjuvant compounds more effectively than MGlu-curdlan-modified liposomes [Citation20]. Moreover, small liposomes modified with MGlu-Aquaβ were found to be more useful for their pH-responsive properties than large liposomes were, because small liposomes induced the release of more content from the liposomes than large liposomes in weakly acidic pH [Citation23]. Nevertheless, the effect of polysaccharide derivative-modified liposome size on immune induction remains unclear.
In the present study, we investigated the size effect of MGlu-Aquaβ-modified liposomes on the interaction with immune cells and assessed their immunity induction properties. The liposome size was controlled using extrusion, as we reported earlier [Citation23]. We evaluated the cellular association, cytokine secretion, antitumour effects and antibody production using antigen-loaded MGlu-Aquaβ-modified liposomes with various sizes to identify the proper liposome size for maximizing liposome vaccine effects.
Materials and methods
Materials
Egg yolk phosphatidylcholine (EYPC, Cat#306-16781) was kindly donated by NOF Corp. (Tokyo, Japan). Cholesterol (Chol, Cat#C75209), ovalbumin (OVA, Cat#A5503) and fetal bovine serum (FBS) were purchased from Sigma-Aldrich Corp. (St. Louis, MO). Phenol and phospholipid C test-Wako (Cat#433-36201) were from FUJIFILM Wako Pure Chemical Corp. (Osaka, Japan). Cell strainer was purchased from Falcon Corning (NY). Chloroform, methanol, sulfuric acid, polyoxyethylene sorbitan monolaurate (Tween 20), penicillin G potassium salt, streptomycin sulfate, MEM Nonessential Amino Acids Solution, RPMI-1640 medium and L-glutamine were from NACALAI TESQUE, INC. (Kyoto, Japan). 1,1′-Dioctadecyl-3,3,3′,3′-tetramethylindocarbocyanine perchlorate (DiI), CLI-095 (TAK-242, Cat#tlrl-cli95-4), whole glucan particles (WGP, Cat#tlrl-wgps) soluble, 2-mercaptoethanol, Mouse Uncoated ELISA Kit (IFN-γ (Cat#88-7314-88), IL-4 (Cat#88-7044-88) and TNF-α (Cat#88-7324-88)) and ELISA/ELISPOT Diluent were obtained from Invitrogen (Massachusetts, USA). Coomassie (Bradford) Protein Assay Kit (Cat#23200), Fixable Viability Dye eFluor® 520 (Cat# 65-0867-14) and CD16/CD32 monoclonal antibody (Cat#14-0161085) were purchased from Thermo Fisher Scientific Inc. (MA). Peroxidase assay kit (Cat#ML-1120T) was obtained from Sumitomo Bakelite CO., Ltd. (Tokyo, Japan). cOmplete™, Mini, EDTA-free Protease inhibitor cocktail was obtained from Roche Corp. (Basel, Switzerland). Anti-CD40-PE (3/23, Cat#124609), anti-CD11c-PE/Cyanine7 (N418, Cat#117318), anti-CD86-APC (GL-1, Cat#105012) and anti-F4/80-APC-Cy750 (BM8, Cat#123118) antibodies were purchased from BioLegend, Inc. (San Diego, CA). β-1,3-1,6-glucan (Aquaβ, Mw: 100 kDa, the degree of branching is 0.71 calculated from 1H NMR) was kindly donated by Osaka Soda Co., Ltd. (Osaka, Japan). MGlu-Aquaβ (MGlu unit: 56% and decylamide group-containing anchor unit: 4% per OH units in Aquaβ) was synthesized in the previous report [Citation20].
Preparation of MGlu-Aquaβ-modified liposomes
5–10 mg of EYPC and Chol (EYPC/Chol = 4/1, mol/mol) dissolved in chloroform was added to a 10-mL round-bottom flask. After removal of chloroform using a rotary evaporator, MGlu-Aquaβ (lipids/polysaccharide derivatives = 7/3 or 8/2 (w/w)) dissolved in methanol were added to the flask. Methanol was evaporated, and the remaining organic solvent was thoroughly removed under vacuum. The obtained mixed thin film of EYPC, Chol and MGlu-Aquaβ was dispersed in phosphate-buffered saline (PBS) for phenol–sulfuric acid method or OVA/PBS solution (4 mg/mL) for other experiments by a brief vortex. The liposome suspension was hydrated by freeze-thaw cycles. The suspension was purified with centrifugation (3300 g, 10 min, 4 °C) three times to obtain OVA-loaded liposomes without extrusion (OL-NE). Subsequently, the liposome suspension was extruded through polycarbonate membranes with 1000, 400 or 200 nm pore sizes to control the liposome size (OL-1000, OL-400, OL-200). OL-1000 and OL-400 were further centrifuged (13,000 g, 30 min, 4 °C) twice to remove the smaller fraction of liposomes. OL-200 was concentrated by centrifugation (126,000 g, 1 h, 4 °C).
Characterization of liposomes
Sizes and zeta potentials of liposomes were measured using a Zetasizer Nano ZS (Malvern Instruments Ltd., Worcestershire, UK). The concentrations of lipid and OVA in liposome suspension were measured using phospholipid C test-Wako and Coomassie Protein Assay Reagent, respectively. Data were obtained as an average of at least three measurements of different samples prepared from different batches. Polysaccharide contents per lipid were measured by using a phenol–sulfuric acid method as previously described [Citation23]. In belief, to 200 μL aqueous solution of MGlu-Aquaβ-modified liposomes (lipid concentration: 0.5 mmol/L), 200 µL of 5% phenol aqueous solution and 1 mL of 98% sulfuric acid were added sequentially. For a calibration curve, mixtures of 0–250 µg/mL of MGlu-Aquaβ and lipid suspension (lipid concentration: 0.5 mmol/L) were prepared. Absorption spectra (450–550 nm) for samples were measured and then polysaccharide contents were calculated from the secondary differentiation of absorbance at 497 nm. The release properties of MGlu-Aquaβ-modified liposomes were measured according to the method reported previously [Citation23]. In belief, pyranine-loaded liposomes were prepared as described above except that mixed thin film of lipids and MGlu-Aquaβ was dispersed in aqueous 35 mmol/L pyranine, 50 mmol/L DPX and 25 mmol/L phosphate solution (pH 7.4). Pyranine-loaded liposomes were added to PBS or the solution adjusted to weakly acidic pH buffered with acetate buffer at 37 °C in a quartz cuvette at lipid concentration of 2.0 × 10−2 mmol/L. Fluorescence intensity at 512 nm of the suspension was followed with excitation at 416 nm using a spectrofluorometer (Jasco FP-6500). The release percentage of pyranine from liposomes was calculated by the equation:
where Fi and Ft are the initial and intermediary fluorescence intensities of the suspension, respectively. Ff is the fluorescent intensity of the suspension after the addition of TritonX-100 at a final concentration of 0.1%.
Cellular association of liposomes
For fluorescent labeling of liposomes, 0.1 mol% DiI in chloroform was added into lipid mixture and then the following procedure was the same as that described above for liposome preparation. DC2.4 cells, a murine dendritic cell (DC) line, were a gift from Dr. K. L. Rock (Harvard Medical School, USA). DC2.4 cells were cultured at 7.5 × 104 cells/well in a 24-well plate for 2 days with culture medium (RPMI-1640 medium supplemented with 10% FBS, 2 mM L-glutamine, 0.1 mmol/L MEM Nonessential Amino Acids Solution, 0.55 μmol/L 2-mercaptoethanol and antibiotics). After washing with PBS twice, DiI-labeled liposome suspension (0.25 mL, 0.4 mmol/L lipid concentration) and culture medium (0.25 mL) were added gently to the cells and then the cells were incubated at 37 °C for 4 h. The cells were washed with PBS three times and the fluorescence intensity of cells was determined via a flow cytometric analysis (CytoFLEX, Beckman Coulter, Inc., Tokyo, Japan). The relative fluorescence intensity for each liposome was calculated compared with that of the cells treated with PBS.
In vitro cytokine analysis
DC2.4 cells were cultured at 1.5 × 105 cells/well in a 12-well plate for 2 days. Liposome suspension (0.5 mL, 0.4 mmol/L lipid concentration) and culture medium (0.5 mL) were added gently to the cells and then the cells were incubated at 37 °C for 24 h. TNF-α in the supernatants was measured using Mouse Uncoated ELISA Kit according to the manufacturer’s instruction. For an inhibition assay, TAK-242 (5 μmol/L) as a TLR4 inhibitor and WGP soluble (1 μg/mL) as an inhibitor of Dectin-1 were pretreated with the cells for 1 h before addition of liposomes. After 24 h incubation with liposomes, TNF-α in the supernatants was measured using a Mouse Uncoated ELISA kit (Invitrogen). The data were recorded and calculated using a microplate reader (SH-8000 Lab, CORONA ELECTRIC Co., Ltd., Japan).
Ethics statement
All animal experiments were approved by the Institutional Animal Experimentation Committee in Osaka Prefecture University (Approval No. 22-1, approved on April 1, 2022) and were performed in compliance with the institutional guidelines of animal care.
Mice
Seven-week-old female C57BL/6 mice (H-2b) and BALB/c were purchased from Oriental Yeast Co., Ltd (Tokyo, Japan). Mice were housed in groups of 4–5 mice per cage at about 25 °C, with water and food provided ad libitum, and kept in a 12-h light/12-h dark cycle. Four or five mice per treatment were used [Citation14, Citation24].
In vivo cytokine analysis in lymph node
BALB/c mice were subcutaneously injected with 100 μL of PBS or liposome suspension containing 20 μg of OVA at the right flank under anaesthesia with 2% isoflurane. Five mice per treatment were used. Inguinal lymph nodes were harvested 7 days after administration. Lymph nodes were gently mashed and passed through a 70 μm mesh cell strainer with 2% FBS/PBS containing protease inhibitor cocktail to obtain single cell suspensions. The suspensions were centrifuged (400 g, 5 min, 4 °C) and cytokines in the cell supernatants were measured using Mouse Uncoated ELISA Kit according to the manufacturer’s instructions. The data were recorded and calculated using a microplate reader. The total protein concentrations of the supernatants were measured using Coomassie Protein Assay Reagent. The cytokine concentrations were normalized by total protein concentrations.
In vivo APC activation in lymph node
BALB/c mice were subcutaneously injected with 100 μL of PBS or liposome suspension containing 20 μg of OVA at the right flank under anaesthesia with isoflurane. Four or five mice per treatment were used. Inguinal lymph nodes were harvested 7 days after administration. The single cells of lymph nodes were stained with Fixable Viability Dye eFluor® 520 (diluted 1:1000 in PBS) for 30 min on ice. After blocking Fc receptors with 5 μg/mL CD16/CD32 monoclonal antibody, the cells were stained with anti-CD40-PE, anti-CD11c-PE/Cyanine7, anti-CD86-APC and anti-F4/80-APC-Cy750 antibodies for 20 min at 4 °C (each diluted 1:200 in 2%FBS/PBS). The cell populations were analyzed via a flow cytometric analysis.
Induction of antitumour immunity
E.G7-OVA cells (Cat#CRL-2113) were obtained from the American Type Culture Collection (Manassas, VA). C57BL/6 mice were subcutaneously inoculated on the left flank with 5.0 × 105 of E.G7-OVA cells, OVA-expressing T-lymphoma, under anaesthesia with isoflurane. On days 7 and 14 after tumour inoculation, 100 μL of PBS or liposome suspension containing 20 μg of OVA were subcutaneously injected into the right flank of the mice under anaesthesia with isoflurane. Tumour sizes were followed from the day of tumour inoculation. The length and width of tumours were measured by using calipers and tumour sizes were calculated by equation: Tumour volume = (length × width2)/2. Endpoint was set when tumour volumes exceeded 2000 mm3. Mice were sacrificed when tumour volumes exceeded 2000 mm3 by cervical dislocation and the death of mice was confirmed by monitoring respiratory and cardiac arrest. All treated groups contained five mice.
In vitro stimulation of splenocytes
C57BL/6 mice were subcutaneously immunized with PBS or liposome suspension containing 20 μg of OVA at the right flank under anaesthesia with isoflurane on days 7 and 14. Five mice per treatment were used. On day 15, splenocytes were collected. The single splenocytes (2 × 106/2 mL) were then incubated with or without OVA for 4 days. IFN-γ secretion in the supernatant was detected using a Mouse Uncoated ELISA kit (Invitrogen). The data was recorded and calculated using a microplate reader.
Analysis of OVA-specific antibody production
Liposomes suspension (100 μL) containing 20 μg of OVA were subcutaneously injected into the right flank of BALB/c mice under anaesthesia with isoflurane on days 0 and 7. Four or five mice per treatment were used. On days 14, 28, 42 and 56, serum was collected from mice and the OVA-specific antibody titer in serum was measured by ELISA. In brief, 96-well plates were coated with 0.01 mg/mL OVA/PBS at 4 °C overnight. The plates were washed with PBS containing 0.1% Tween 20 (PBS-T) and were blocked with ELISA/ELISPOT Diluent at 37 °C for 2 h. After washing with PBS-T, serial two-fold dilutions of sera were added and incubated at 4 °C overnight. The plates were washed with PBS-T and added with Horseradish peroxidase-labeled goat anti-mouse IgG1 (Invitrogen, Cat# PA1-74421) and IgG2a (Invitrogen, Cat# M32207) antibody (IgG1: 1/2000 dilution, IgG2a: 1/500 dilution) for 2 h at 37 °C. After incubation for 2 h at 37 °C, the detection of antigen-antibody complexes was performed by using a peroxidase assay kit. After washing, substrate solution was added and incubated for 10 min at room temperature. Stop solution (2 mol/L sulfuric acid) was added and the optical density of each well was read at 450 nm on a microplate reader (SH-8000Lab, Corona Electric CO., Ltd., Ibaraki, Japan).
Statistical analysis
Statistically significant differences between experimental groups were determined using Prism software (v10, GraphPad). One-way analysis of variance followed by Tukey’s HSD post hoc test was used; variance between groups was found to be similar by Brown-Forsythe test. The symbols *, **, *** and **** indicate p values less than .05, .01, .001 and .0001, respectively.
Results
Preparation and characteristics of size-controlled MGlu-Aquaβ-modified liposomes
We previously performed the comparative experiments between liposomes modified with or without MGlu-Aquaβ using 200 nm size liposomes [Citation20]. For cytokine production, modification of MGlu-Aquaβ significantly increased the cytokine secretion from DC2.4 cells (Supplemental Figure S1). Therefore, we focused the size effect of liposomes modified with MGlu-Aquaβ. Size control of MGlu-Aquaβ-modified liposomes was performed using a method reported earlier [Citation23]. The liposome suspensions were passed through polycarbonate membranes with 1000, 400 and 200 nm pore sizes, respectively, to obtain OVA-loaded liposomes with various sizes (OL-1000, OL-400 and OL-200). The sizes of OL-1000, OL-400 and OL-200 were, respectively, 756, 316 and 148 nm ( and Supplemental Figure S2). The liposomes showed almost identical particle sizes to those of pore sizes of membranes used for extrusion. As larger liposomes, we also prepared liposomes without extrusion (OL-NE), with average size of 1425 nm. The amount of MGlu-Aquaβ per lipid in OL-NE prepared at a ratio of lipids/polysaccharide derivatives (7/3, w/w) was 0.208 mg/mg: about twice as high as those in OL-1000, OL-400, and OL-200 reported earlier [Citation23] (Supplemental Figure S3) because they have no extrusion and less purification times than extruded liposomes have. To adjust the polysaccharide derivative contents per lipid, OL-NE samples were prepared with lipids and polysaccharide derivatives at a ratio of 8/2 (w/w). The amount of MGlu-Aquaβ per lipid in OL-NE was 0.071 (mg/mg), which was almost equal to the amounts in OL-1000, OL-400 and OL-200 (Figure S3). The zeta potentials of OL-NE, OL-1000, OL-400 and OL-200 were, respectively, −43.6, −52.0, −38.8 and −54.6 mV (). The OVA-loading amounts in OL-NE, OL-1000, OL-400 and OL-200 were almost equal: 61.5, 59.1, 53.9 and 51.8 g/mol, respectively (). The pH-responsive property of MGlu-Aquaβ-modified liposomes was confirmed by pyranine release from liposomes in weakly acidic conditions (Supplemental Figure S4).
Table 1. Characteristics of EYPC/chol (4/1, mol/mol) liposomes modified with MGlu-aquaβ.
In vitro cytokine analysis and cellular association of liposomes
To evaluate the liposome size effect on adjuvant properties of MGlu-Aquaβ-modified liposomes, DC2.4 cells were incubated with liposomes of various sizes (OL-NE, OL-1000, OL-400, OL-200) for 24 h. OL-NE showed markedly higher TNF-α secretion than either OL-1000, OL-400, OL-200 or PBS (). To investigate why the different liposome sizes showed different adjuvant properties, we evaluated the effects of cellular surface receptors on the adjuvant properties of liposomes. Zhang et al. [Citation25] reported that certain polysaccharides including β-glucan induced TLR4-mediated activation of immune cells. Ikeda et al. [Citation26] reported that complexes of β-1,3-1,6-glucan and fullerene were taken up more effectively by cells expressing higher levels of Dectin-1, indicating that β-1,3-1,6-glucan interacts with cells via Dectin-1. Therefore, the effects of TLR4 and Dectin-1 on cytokine production were investigated. Pre-treatment of TAK-242 (a signaling inhibitor of TLR4) significantly suppressed TNF-α secretion from cells treated with OL-NE and OL-1000 compared with no-treatment of TLR4 inhibition (). TNF-α secretion from cells treated with OL-200 tended to be less pronounced in the presence of TAK-242 (p = .166). This result implies that OL-NE, OL-1000 and OL-200 induced activation of DCs because of recognition via TLR4. TNF-α secretion from cells pre-treated with soluble WGP (a binding inhibitor of Dectin-1) was similar to that from cells without soluble WGP treatment (Supplemental Figure S5). This result suggests that MGlu-Aquaβ-modified liposomes were not recognized by Dectin-1. Conjugation of MGlu unit to Aquaβ might prevent recognition of the Aquaβ backbone by Dectin-1.
Figure 1. TNF-α secretion from DC2.4 cells treated with MGlu-Aquaβ-modified liposomes and TLR4 inhibition assay. DC2.4 cells were pre-incubated with or without TAK-242 (TLR4 signal inhibitor, 5 μmol/L). After 1 h incubation, the cells were treated with MGlu-Aquaβ-modified liposomes for 24 h (lipid concentration: 0.2 mmol/L). TNF-α in cell culture supernatants was measured using ELISA. OL-NE, non-extruded and OVA-loaded liposomes. OL-1000, OL-400, OL-200, OVA-loaded liposomes extruded through polycarbonate membranes of 1000, 400 or 200 nm pore sizes. Results denote the mean of triplicate experiments ± SEM. *p < .05, **p < .01, ***p < .001, ****p < .0001.
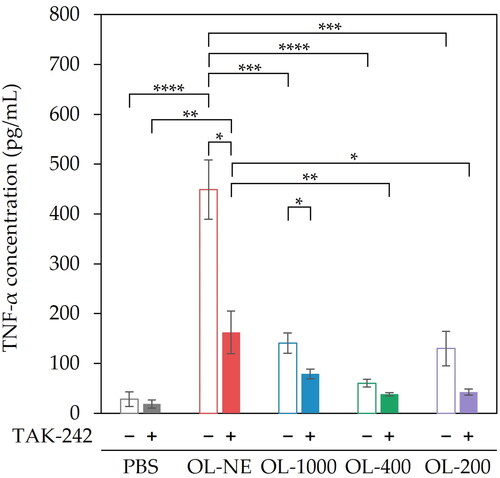
To evaluate the cellular associations of various size-controlled liposomes, the fluorescence intensities of DC2.4 cells were measured 4 h after treatment with DiI-labeled liposomes. No significant difference was found for any size liposome, but association of MGlu-Aquaβ-modified liposomes to cells tended to increase depending on the liposome size from OL-200 to OL-1000 (). OL-NE tended to decrease the cellular association with OL-1000, but there was no significant difference between the cellular association with OL-NE and OL-1000.
Figure 2. Cellular association of MGlu-Aquaβ-modified liposomes. DC2.4 cells were incubated with various size-controlled DiI-labeled liposomes modified with MGlu-Aquaβ (OL-NE, OL-1000, OL-400 or OL-200) for 4 h. The fluorescence intensities of the cells were analyzed using a flow cytometer. OL-NE, non-extruded and OVA-loaded liposomes. OL-1000, OL-400, OL-200, OVA-loaded liposomes extruded through polycarbonate membranes of 1000, 400 or 200 nm pore sizes. Results represent the mean values of triplicate experiments ± SEM.
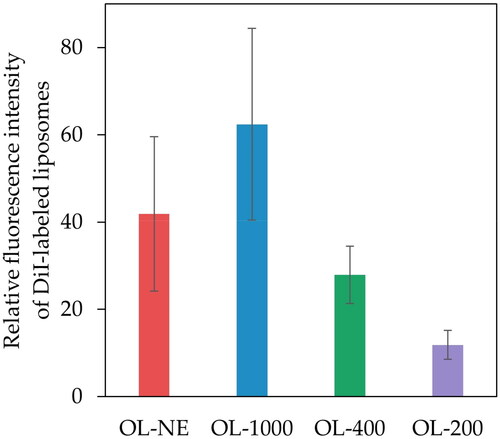
In vivo cytokine analysis in lymph node
To evaluate the adjuvant properties of various size-controlled liposomes in vivo, cytokines in lymph nodes were measured after BALB/c mice were injected subcutaneously with MGlu-Aquaβ-modified liposomes. OL-NE and OL-1000 significantly increase TNF-α secretion from immune cells in lymph nodes compared with OL-400 and PBS, and OL-200 tended to increase TNF-α secretion compared with OL-400 and PBS. Although there was no significant difference between, OL-NE, OL-1000 and OL-200 tended to increase IFN-γ, IL-4 and IL-12 secretion in lymph nodes compared with PBS (). These liposomes might interact with TLR4 expressed on APCs at an injection site or in lymph nodes, activating immune cells and inducing cytokine secretion. In lymph nodes, injection of liposomes tended to slightly increase the percentages of DCs and macrophages expressing CD40 or CD86 molecules compared with PBS (Supplemental Figure S6). These results suggest that MGlu-Aquaβ-modified liposomes activated DCs and macrophages in lymph nodes. OL-400 injection induced significant increase in CD40+ macrophages within lymph nodes (Supplemental Figure S6c). This suggests that OL-400 would have relatively high affinity to lymph node-resident macrophages although the detailed mechanism is unclear.
Figure 3. Cytokine secretion in lymph nodes derived from mice immunized with PBS, OL-NE, OL-1000, OL-400 or OL-200. IFN-γ (a), TNF-α (b), IL-4 (c) and IL-12 (d) in lymph nodes collected 7 days after immunization were measured using ELISA. Each result represents the mean value ± SEM for a group of five mice. OL-NE, non-extruded and OVA-loaded liposomes. OL-1000, OL-400, OL-200, OVA-loaded liposomes extruded through polycarbonate membranes of 1000, 400 or 200 nm pore sizes. *p < .05, **p < .01.
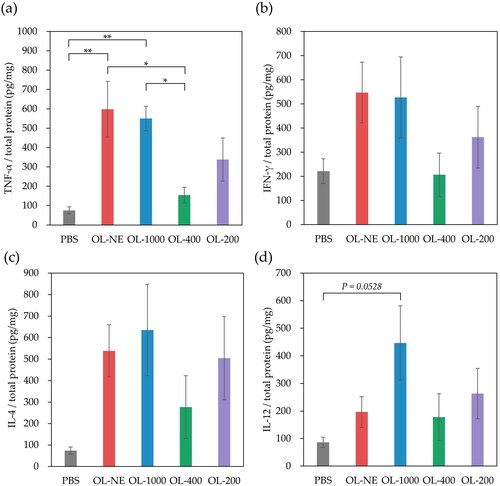
Induction of antitumour immunity
The effects of liposome size on the antitumour activity were evaluated using mice inoculated with OVA-expressing tumour cells (E.G7-OVA cells). OVA-loaded liposomes modified with MGlu-Aquaβ were injected subcutaneously to tumour-bearing mice on days 7 and 14 after tumour inoculation. The tumour volumes of mice treated with PBS increased rapidly and reached endpoints within 30 days ( and S7). In contrast, treatment with all size liposomes suppressed the tumour growth in mice on day 20 significantly compared to treatment with PBS ( and Supplemental Table S1). As shown in , all liposomes tended to improve the survival of mice, although OL-200 did not show significant difference (p = .063) probably because two mice treated with OL-200 that reached an endpoint earlier than those treated with other liposomes might increase the p value. In addition, 20%, 20% and 60% of mice treated respectively with OL-NE, OL-1000 and OL-200 became tumour-free with no tumour recurrence observed (Supplemental Figures S7 and S8). These results suggest that all size liposomes could induce antitumour immunity to attack antigen-presenting tumour cells and OL-200 achieved remarkably strong antitumour effect compared with other size liposomes. To compare the performance of lymph node migration between large and small liposomes, DiI-labeled OL-NE or OL-200 were subcutaneously injected, then DiI fluorescence derived from lymph node and injection site was investigated. At 24 h after injection of DiI-labeled OL-200, significantly high DiI-derived fluorescence was detected in the draining lymph node compared with OL-NE (Supplemental Figure S9a). OL-NE was retained more effectively at the injection site compared with OL-200 (Supplemental Figure S9b) and was detected in lymph nodes even on Day 7 after injection (Supplemental Figure S9a). These results imply that OL-200 might migrate from the injection site to lymph nodes earlier after injection and OL-NE might remain at the injection site for long-term periods compared with OL-200. OL-200 induced IFN-γ production from splenocytes responding to in vitro OVA stimulation (Supplemental Figures S10), indicating that OL-200 induced OVA-specific cellular immune responses. The mice body weight decreased slightly after administration of liposomes (weight loss: about 5% or less), but body weights recovered within 2 days (Supplemental Figure S11). This result indicated that all sizes of MGlu-Aquaβ-modified liposomes had low side effects.
Figure 4. Antitumor effects of OVA-loaded liposomes modified with MGlu-Aquaβ. Changes in the mice tumor volume (a) and survival of mice (b) were monitored after E.G7-OVA cell inoculation (5.0 × 105 cells per mouse). C57BL/6 mice were immunized on days 7 and 14 after tumor inoculation with PBS and liposomes. Arrows indicate the days of sample injection. OL-NE, non-extruded and OVA-loaded liposomes. OL-1000, OL-400, OL-200, OVA-loaded liposomes extruded through polycarbonate membranes of 1000, 400 or 200 nm pore sizes. Each result represents the mean ± SEM for a group of five mice. *p < .05.
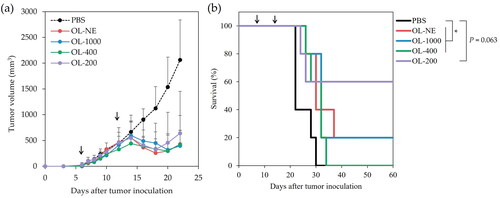
Analysis of OVA-specific antibody
To evaluate OVA-specific antibody production of large and small liposomes, we measured OVA-specific IgG1 and IgG2a in sera of mice treated with OL-NE and OL-200. Treatment of OL-NE induced significantly higher IgG1 production than that of OL-200 (). Treatment of OL-NE also enhanced IgG2a production compared with that of OL-200 (). Comparison of temporal changes of IgG1 and IgG2a production shows that treatment of OL-200 tends to increase IgG1 slightly and that it does not greatly change IgG2a production during days 14–56. However, treatment of OL-NE tended to increase both IgG1 and IgG2a greatly over time. These results imply that OL-NE tends to increase the humoral response more than OL-200 does. In IgG2a/IgG1 ratio, OL-200 tended to show higher values than that of OL-NE until day 42 (Supplemental Figure S12). This tendency implies that treatment with OL-200 shifted the Th1/Th2 balance to Th1 immunity earlier than OL-NE.
Discussion
Liposomes are beneficial as an antigen carrier in evoking immune response. This is because both hydrophilic and hydrophobic antigens can be loaded within liposomes, and liposome can be functionalized with modification by various materials [Citation27, Citation28]. Liposome size affects cellular uptake by immune cells, activation of immune cells or the type of induced immune response [Citation14, Citation29]. Thus, identifying proper liposome size is important for designing functional liposomal vaccines for immunotherapy.
In this study, we investigated the effects of MGlu-Aquaβ-modified liposome size on the immune induction property. Different sized liposomes were prepared using extrusion through polycarbonate membranes of different pore sizes similar to a method reported earlier [Citation23]. In an earlier report [Citation20], we described that unmodified EYPC liposomes showed −7.9 mV of zeta potential. The highly negative zeta potentials of liposomes imply that these liposomes were modified with MGlu-Aquaβ having carboxy groups. The pH-responsive content release property implies that all sizes of MGlu-Aquaβ-modified liposomes destabilize and release contents in response to endosomal pH.
OL-NE induced high TNF-α secretion from DC2.4 cells, implying that OL-NE has higher adjuvant ability than other liposomes. Inhibition of TLR4 signaling with TAK-242 tended to suppress TNF-α secretion from DC2.4 cells treated with OL-NE, OL-1000 and OL-200, indicating the liposomes associate to DCs via TLR4 and activate DCs. Interestingly, when TLR4 signaling was inhibited with TAK-242, OL-NE still induced a markedly higher amount of TNF-α secretion than OL-400, OL-200 and PBS, suggesting that OL-NE interacts with other surface receptors in addition to TLR4. Dectin-1 inhibition experiments with soluble WGP suggested that all size MGlu-Aquaβ-modified liposomes were not recognized by Dectin-1. This might be attributed to the fact that conjugation of MGlu unit to Aquaβ might prevent recognition of the Aquaβ backbone by Dectin-1.
The association of OL-1000, OL-400 and OL-200 tended to increase with increasing liposome size. Chono et al. [Citation30] also reported that uptake of hydrogenated soybean phosphatidylcholine/Chol/ dicetylphosphate (7/2/1, mol/mol/mol) liposomes is dependent on liposome size from 97 nm to 1015 nm; they also reported that uptake of 2059 nm liposome is similar to that of 1015 nm liposome. As the earlier reports describe, the association of liposomes might increase to a certain liposome size, depending on the liposome size. Moreover, the associative capacity of liposomes might be constrained at greater than a certain size. Garrett et al. [Citation31] reported that immature DCs take up antigens more efficiently than mature DCs do. Since OL-NE induced higher TNF-α secretion from DCs than the other liposomes did, OL-NE-induced maturation of the cells might suppress the cellular association of OL-NE compared with OL-1000.
OL-NE significantly increased TNF-α secretion compared with OL-1000, OL-400 and OL-200, but no significant difference was found between OL-NE, OL-1000 and OL-200 in cytokine secretion within lymph nodes. This might be attributed to the fact that TNF-α is secreted from not only DCs but also from various immune cells at injection sites and lymph nodes of mice. Although OL-400 has twice higher cellular association than OL-200, OL-400 exhibited only a half of cytokine secretion as compared with OL-200. These data imply that OL-400 might have relatively low adjuvant property compared with liposomes of other sizes, resulting in relatively low activation performance in lymph nodes. More detailed analyses on liposome interaction with cellular receptors including TLR4, Dectin-1 and scavenger receptors using primary cultured DCs from a specific receptor-knockout mouse are required to reveal the contribution of these receptors on the adjuvant effect of liposomes.
The survival rates associated with OL-NE and OL-1000 were lower than that with OL-200. This might be attributed to the fact that the large liposomes remained at the injection site and migrated to lymph nodes later than OL-200 did. The DiI-fluorescence derived from OL-NE was higher at the injection site than that of OL-200 even on Day 7, which also corresponds to previous literature [Citation32]. Oussoren et al. [Citation32] reported that the remaining amounts of liposomes at the injection site were negatively correlated with liposome size, which indicated that lymphatic uptake increased concomitantly with decreasing liposome size. As we reported earlier [Citation23], MGlu-Aquaβ-modified liposomes of 100–200 nm released more contents under weakly acidic pH than liposomes larger than 200 nm did. Therefore, OL-200 might better release encapsulated antigens under weakly acidic conditions of endosomes than large liposomes to induce cellular immunity.
The trend in which OL-NE enhanced IgG2a production compared with OL-200 was associated with the OL-NE tendency to increase IFN-γ secretion in lymph nodes more than OL-200 did. IFN-γ induced IgG2a subclass switching in B cells [Citation33]. Th1 cells secrete IFN-γ to induce proliferation of CTLs and activation of APCs [Citation34]. Oussoren et al. [Citation32] reported that, after subcutaneous injection, liposome amounts in the blood and liver increased concomitantly with decreasing liposome size. OL-200 might be metabolized rapidly, whereas OL-NE might remain at the injection site, release OVA, and prime T cells to induce Th2 cells over a longer term. Therefore, OL-NE might increase IgG1 and IgG2a production over time. The Th1/Th2 balance tendency implies that treatment with OL-200 induces Th1 immunity earlier than OL-NE, which is also important to induce cellular immune responses at an earlier timeframe to achieve tumour regression by OL-200.
We selected female mice for animal studies because female mice immunized with antigens induced higher numbers of CD4+ and CD8+ T cells, and higher levels of antigen-specific antibody in response to antigen challenge than male mice did [Citation35, Citation36]. The results obtained in this study (Supplemental Figure S13) are still limited in mouse and OVA model, which is immunogenic against mouse. In a viewpoint of practical application, further experiments using other antigens, other types of tumour models, other mouse strains and humanized models are required [Citation37–39]. In this study, we evaluated the production of IgG2a and IgG1 in mouse model. However, we cannot simply extrapolate these results to human immune systems. More detailed immunological analyses and precise design of liposomal carriers are required towards the application of clinical applications.
Conclusions
Four sizes of pH-responsive polysaccharide derivative-modified liposomes were prepared using extrusion with difference pore sizes of polycarbonate membranes. Liposomes with a size of more than 700 nm tended to induce high TNF-α secretion and to enhance association of liposomes with DCs in vitro. Subcutaneous administration of liposomes increased TNF-α secretion at lymph nodes. Moreover, administration of 200 nm liposomes completely eradicated the tumours in 60% of the treated animals. For antibody production, the ratios of IgG2a to IgG1 in mice treated with 200 nm liposomes increased early after immunization. By contrast, the largest liposomes induced high IgG2a and IgG1 production compared with 200 nm liposomes. These results would be attributed to early lymph node migration of small liposomes and long retention of large liposomes at the injection site. Therefore, selecting a suitable size is important to design effective liposomal vaccines that can induce cellular immunity or humoral immunity and to aid in therapeutic effects in tumour or viral infection and prophylactic effects for viruses and bacteria.
Authors’ contribution
Conceptualization, S.Y. and E.Y.; methodology, S.Y.; validation, E.Y.; formal analysis, S.Y.; investigation, S.Y.; data curation, E.Y. and A.H.; writing—original draft preparation, S.Y. and E.Y.; writing—review and editing, E.Y.; visualization, S.Y.; supervision, E.Y.; project administration, E.Y.; funding acquisition, E.Y. All authors have read and agreed to the published version of the manuscript.
Institutional review board statement
The animal study protocol was approved by the Institutional Review Board of Osaka Prefecture University (protocol code No. 22-1, April 1, 2022).
Supplemental Material
Download PDF (2.3 MB)Acknowledgment
The authors appreciate Maki Ohashi (Sanyo Fine Co., Ltd., Osaka, Japan) for his kind support in providing Aquaβ.
Disclosure statement
The authors declare no conflict of interest.
Data availability statement
All data needed to evaluate the conclusions in the article are present in the article and/or the Supplementary Materials. Additional data are available from the corresponding author [E.Y.] upon reasonable request.
Additional information
Funding
References
- Cheng ZJ, Shan J. 2019 Novel coronavirus: where we are and what we know. Infection. 2020;48(2):1–13. doi: 10.1007/s15010-020-01401-y.
- Li M, Wang H, Tian L, et al. COVID-19 vaccine development: milestones, lessons and prospects. Signal Transduct Target Ther. 2022;7:146.
- Zheng J, Deng Y, Zhao Z, et al. Characterization of SARS-CoV-2-specific humoral immunity and its potential applications and therapeutic prospects. Cell Mol Immunol. 2022;19(2):150–157. doi: 10.1038/s41423-021-00774-w.
- Ruterbusch M, Pruner KB, Shehata L, et al. In vivo CD4+ T cell differentiation and function: revisiting the Th1/Th2 paradigm. Annu Rev Immunol. 2020;38:705–725.
- Ong DSY, Fragkou PC, Schweitzer VA, et al. How to interpret and use COVID-19 serology and immunology tests. Clin Microbiol Infect. 2021;27:981–986.
- Aleebrahim-Dehkordi E, Molavi B, Mokhtari M, et al. T helper type (Th1/Th2) responses to SARS-CoV-2 and influenza A (H1N1) virus: from cytokines produced to immune responses. Transpl Immunol. 2022;70:101495.
- Koh CH, Lee S, Kwak M, et al. CD8 T-cell subsets: heterogeneity, functions, and therapeutic potential. Exp Mol Med. 2023;55(11):2287–2299. doi: 10.1038/s12276-023-01105-x.
- Auladell M, Jia X, Hensen L, et al. Recalling the future: immunological memory toward unpredictable influenza viruses. Front Immunol. 2019;10:1400. doi: 10.3389/fimmu.2019.01400.
- Kaseke C, Tano-Menka R, Senjobe F, et al. The emerging role for CTL epitope specificity in HIV cure efforts. J Infect Dis. 2021;223:32–37.
- Schwendener RA. Liposomes as vaccine delivery systems: a review of the recent advances. Ther Adv Vaccines. 2014;2(6):159–182. doi: 10.1177/2051013614541440.
- Riaz MK, Riaz MA, Zhang X, et al. Surface functionalization and targeting strategies of liposomes in solid tumor therapy: a review. Int J Mol Sci. 2018;19:195.
- Inglut CT, Sorrin AJ, Kuruppu T, et al. Immunological and toxicological considerations for the design of liposomes. Nanomaterials. 2020;10(2):190. doi: 10.3390/nano10020190.
- Brewer JM, Tetley L, Richmond J, et al. Lipid vesicle size determines the Th1 or Th2 response to entrapped antigen. J Immunol. 1998;161(8):4000–4007. doi: 10.4049/jimmunol.161.8.4000.
- Matsuoka Y, Onohara E, Kojima N, et al. Importance of particle size of oligomannose-coated liposomes for induction of Th1 immunity. Int Immunopharmacol. 2021;99:108068.
- Badiee A, Khamesipour A, Samiei A, et al. The role of liposome size on the type of immune response induced in BALB/c mice against leishmaniasis: rgp63 as a model antigen. Exp Parasitol. 2012;132:403–409.
- Mann JFS, Shakir E, Carter KC, et al. Lipid vesicle size of an oral influenza vaccine delivery vehicle influences the Th1/Th2 bias in the immune response and protection against infection. Vaccine. 2009;27(27):3643–3649. doi: 10.1016/j.vaccine.2009.03.040.
- Yamamoto S, Ishida T, Inoue A, et al. HEPC-based liposomes trigger cytokine release from peripheral blood cells: effects of liposomal size, dose and lipid composition. Int J Pharm. 2002;236(1–2):125–133. doi: 10.1016/s0378-5173(02)00026-1.
- Yuba E, Yamaguchi A, Yoshizaki Y, et al. Bioactive polysaccharide-based pH-sensitive polymers for cytoplasmic delivery of antigen and activation of antigen-specific immunity. Biomaterials. 2017;120:32–45. doi: 10.1016/j.biomaterials.2016.12.021.
- Okubo M, Miyazaki M, Yuba E, et al. Chondroitin sulfate-based pH-Sensitive polymer-modified liposomes for intracellular antigen delivery and induction of cancer immunity. Bioconjug Chem. 2019;30:1518–1529.
- Yanagihara S, Kasho N, Sasaki K, et al. pH-Sensitive branched β-glucan-modified liposomes for activation of antigen presenting cells and induction of antitumor immunity. J Mater Chem B. 2021;9:7713–7724.
- Miyazaki M, Yuba E, Hayashi H, et al. Development of pH-responsive hyaluronic acid-Based antigen carriers for induction of antigen-specific cellular immune responses. ACS Biomater Sci Eng. 2019;5(11):5790–5797. doi: 10.1021/acsbiomaterials.9b01278.
- Yuba E, Tajima N, Yoshizaki Y, et al. Dextran derivative-based pH-sensitive liposomes for cancer immunotherapy. Biomaterials. 2014;35(9):3091–3101. doi: 10.1016/j.biomaterials.2013.12.024.
- Yanagihara S, Kitayama Y, Yuba E, et al. Preparing size-controlled liposomes modified with polysaccharide derivatives for pH-responsive drug delivery applications. Life. 2023;13(11):2158. doi: 10.3390/life13112158.
- Sultan H, Wu J, Fesenkova VI, et al. Poly-IC enhances the effectiveness of cancer immunotherapy by promoting T cell tumor infiltration. J Immunother Cancer. 2020;8(2):e001224. doi: 10.1136/jitc-2020-001224.
- Zhang X, Qi C, Guo Y, et al. Toll-like receptor 4-related immunostimulatory polysaccharides: primary structure, activity relationships, and possible interaction models. Carbohydr Polym. 2016;149:186–206.
- Ikeda A, Akiyama M, Sugikawa K, et al. Formation of β-(1,3-1,6)-d-glucan-complexed [70]fullerene and its photodynamic activity towards macrophages. Org Biomol Chem. 2017;15(45):9734. doi: 10.1039/c7ob90179h.
- Gu Z, Da Silva CG, Van der Maaden K, et al. Liposome-based drug delivery systems in cancer immunotherapy. Pharmaceutics. 2020;12(11):1054. doi: 10.3390/pharmaceutics12111054.
- Thapa Magar K, Boafo GF, Li X, et al. Liposome-based delivery of biological drugs. Chin Chem Lett. 2022;33:587–596.
- Lou G, Anderluzzi G, Woods S, et al. A novel microfluidic-based approach to formulate size-tuneable large unilamellar cationic liposomes: formulation, cellular uptake and biodistribution investigations. Eur J Pharm Biopharm. 2019;143:51–60. doi: 10.1016/j.ejpb.2019.08.013.
- Chono S, Tanino T, Seki T, et al. Uptake characteristics of liposomes by rat alveolar macrophages: influence of particle size and surface mannose modification. J Pharm Pharmacol. 2007;59(1):75–80. doi: 10.1211/jpp.59.1.0010.
- Garrett WS, Chen LM, Kroschewski R, et al. Developmental control of endocytosis in dendritic cells by Cdc42. Cell. 2000;102(3):325–334. doi: 10.1016/s0092-8674(00)00038-6.
- Oussoren C, Zuidema J, Crommelin DJA, et al. Lymphatic uptake and biodistribution of liposomes after subcutaneous injection. II. Influence of liposomal size, lipid composition and lipid dose. Biochim Biophys Acta Biomembr. 1997;1328:261–272.
- Dahlgren MW, Plumb AW, Niss K, et al. Type I interferons promote germinal centers through B cell intrinsic signaling and dendritic cell dependent Th1 and tfh cell lineages. Front Immunol. 2022;13:932388. doi: 10.3389/fimmu.2022.932388.
- Szeponik L, Akeus P, Rodin W, et al. Regulatory T cells specifically suppress conventional CD8αβ T cells in intestinal tumors of APCMin/+ mice. Cancer Immunol Immunother. 2020;69(7):1279–1292. doi: 10.1007/s00262-020-02540-9.
- Ahnstedt H, McCullough LD. The impact of sex and age on T cell immunity and ischemic stroke outcomes. Cell Immunol. 2019;345:103960.
- Schaefer AL, Ceesay M, Leier JA, et al. Factors contributing to sex differences in mice inhaling Aspergillus fumigatus. Int J Environ Res Public Health. 2020;17(23):8851. doi: 10.3390/ijerph17238851.
- Bleul T, Zhuang X, Hildebrand A, et al. Different innate immune responses in BALB/c and C57BL/6 strains following corneal transplantation. J Innate Immun. 2021;13(1):49–59. doi: 10.1159/000509716.
- Van Hoecke L, Van Lint S, Roose K, et al. Treatment with mRNA coding for the necroptosis mediator MLKL induces antitumor immunity directed against neo-epitopes. Nat Commun. 2018;9(1):3417. doi: 10.1038/s41467-018-05979-8.
- Sagiv-Barfi I, Czerwinski DK, Shree T, et al. Intratumoral immunotherapy relies on B and T cell collaboration. Sci Immunol. 2022;7(71):eabn5859. doi: 10.1126/sciimmunol.abn5859.