Abstract
DEEPER ROOTING 1 (DRO1) of rice controls the gravitropic response of root growth angle. In order to clarify the effects of DRO1 on root growth angle and root length density under different soil resistance to penetration, and to quantify the relationship between root growth angle and root length density, we assessed the root growth of Dro1-NIL (a near-isogenic line homozygous for the Kinandang Patong allele of DRO1 in the IR64 background) under upland Andosol field conditions in Japan in 2013 and 2014. The trial included three levels of soil compaction (none, moderate, and hard). Root length density at a depth of 30 to 60 cm was largest in Kinandang Patong, followed by Dro1-NIL, and was least in IR64 in both years and in all compaction treatments. Root length density at this depth decreased with hard compaction (to 70% of control) and increased with moderate compaction (to 135%). The number of roots with a deep angle (i.e. 45° to 90° from the horizontal) measured by the basket method was similar at maximum tillering and maturity stages, and its value as a proportion of the total number of roots was strongly correlated with the root length density at 30 to 60 cm in both years, which demonstrates the importance of a deep root angle for the development of deep roots. Dro1-NIL had a higher proportion of deep roots than IR64, but the difference was small under hard compaction, with a significant genotype × compaction interaction.
Classification:
Introduction
Deep rooting offers many advantages to plants, including greater mechanical stability and greater acquisition of resources such as nutrients and water during crucial growth stages, including under water and nutrient deficit conditions, thereby helping plants to attain greater biomass production and yield than shallow-rooted plants. Root growth angle, which affects how deeply roots penetrate into the soil, is regulated by multiple genes, as well as by environmental factors and plant growth stages (Abe & Morita, Citation1994). These factors, together with the elongation and branching patterns of the roots, define a framework for the root system (Yamauchi et al., Citation1987). The root growth angle is defined as the angle between the horizontal and the long axis of each root, and can be quantified to provide a synthetic indicator of the proportion of the total number of roots that grow in a primarily vertical direction (Oyanagi, Citation1994). The numbers of roots and their proportion can be quantified using the ‘root basket method’, in which plants are grown within a mesh basket so that root emergence at different levels can be easily observed (Oyanagi et al., Citation1993).
Root growth angle can be simply measured in a hydroponic system using a small basket at the young seedling stage (e.g. around 1 month after sowing); such studies have contributed to the identification of quantitative trait loci (QTLs) for root growth angle (Kitomi et al., Citation2015; Uga et al., Citation2011, Citation2013a, Citation2015). However, the effectiveness of the root basket method with field-grown rice plants at different ages and under different levels of ‘soil strength (i.e. resistance to penetration)’ has not been tested sufficiently. Large differences in the proportion of roots with a deep angle have sometimes been recorded at the young seedling stage (e.g. 12% vs. 93% for two cultivars; Uga et al., Citation2011), but it is unknown whether such large differences are maintained during later growth stages in field-grown plants. Furthermore, it is not clear whether and to what extent a deep root angle contributes to the prevalence of deep roots (i.e. to the root length density in deep soil layers). It is, therefore, necessary to quantify the variation in deep root angle during later growth stages under field conditions, and to clarify the relationship between the root growth angle measured by the root basket method and actual root length density in the field, as well as the effects of the resistance to penetration on this relationship, because it is the root length density in deep soil layers that supports growth through the acquisition of water and other resources from the subsoil, particularly under drought conditions (Lynch, Citation2013; Nguyen et al., Citation1997; Yoshida & Hasegawa, Citation1982).
Soil with a high resistance to root penetration can decrease the proportion of roots that reach the deep soil, since soil strength is usually distributed non-homogeneously throughout the depth profile, but typically has a peak value at some depth (e.g. a hardpan 15 to 20 cm below the surface). When roots approach a layer of strong soil, they may either penetrate the layer or may get a deflection from their original direction (Dexter & Hewitt, Citation1978). Soil compaction is known to decrease root elongation rate and increase cross-sectional area of roots (Lipiec et al., Citation2012; Rich & Watt, Citation2013), but its effects on root growth angle are not clarified.
Recently, DEEPER ROOTING 1 (DRO1) was isolated as a functional allele that controls the gravitropic curvature of rice roots. This gene was identified in the deep-rooting cultivar Kinandang Patong (a traditional tropical japonica upland cultivar from the Philippines) and originated in the genetic background of the shallow rooting parent cultivar IR64, which is a modern lowland indica cultivar that is widely grown in South and South-east Asia (Uga et al., Citation2013a). Its effects on rice growth have been examined under droughted upland conditions and flooded lowland conditions at different fertilizer application rates (Arai-Sanoh et al., Citation2014; Uga et al., Citation2013a). The results showed that DRO1 plays a significant role in the acquisition of resources that permit higher yield. However, the effects of DRO1 under different levels of soil compaction have not been studied.
Here, our first goal was to clarify the effects of DRO1 on root growth angle and root length density deep in the soil at maximum tillering and maturity stages under field conditions that included three levels of soil compaction. Our second goal was to quantify the relationship between the proportion of roots with a deep growth angle (measured by the basket method) and the actual deep root length density measured in the field by means of core sampling (i.e. to determine whether the basket results can be used to predict performance in the field). We defined two research hypotheses: (1) that DRO1 should increase deep rooting compared with IR64 and produce a greater root length in deep soil layers under upland conditions, but that the difference would decrease with increasing soil compaction owing to the soil’s impedance of root elongation; and (2) that a greater proportion of roots with a deeper growth angle would increase root length density in deeper soil layers only when soil strength did not greatly impede root elongation.
Materials and methods
Experimental site
Two field experiments were conducted under rain-fed upland conditions at Nishitokyo (35°43′N, 39°32′E) from May to October in 2013 and 2014. (Details of the environmental conditions are provided later in the Methods.) We used three rice genotypes: IR64, a shallow-rooting lowland indica cultivar; Kinandang Patong, a deep-rooting upland tropical japonica cultivar; and Dro1-NIL, a near-isogenic line homozygous for the Kinandang Patong allele of DRO1 in the IR64 background. The soil was a typical Japanese volcanic ash soil of the Kanto loam type which consist of a top soil rich in humus (i.e. a humic Andosol of a dark humic silty loam to a depth of ca. 30 cm) and a subsoil of red-brown silty clay loam (below ca. 30 cm) (Yamagishi et al., Citation2003).
Experimental design
In 2013, the three genotypes were grown in upland Andosol fields to provide a basis for their comparison without any soil compaction treatment. The study used a completely randomized design with three replicates. Each plot covered .91 m2, comprising .64 m2 that held 16 plants transplanted into the soil and .27 m2 that held 3 plants that were transplanted in root baskets (as described later in this section).
In 2014, all fields were rototilled to a depth of about 10 cm on 30 May, followed by the compaction treatment. The three genotypes were grown under three levels of soil compaction: no compaction; moderate compaction by passage of a tractor equipped with a roller, and hard compaction created by a 3230-kg excavator that produced an average contact pressure of 32 kPa (303C SR, Caterpillar Japan Ltd., Tokyo, Japan) (Supplementary Figure 1). The soil resistance to penetration created by these treatments is described later in the Methods. Each treatment covered an area of 2 m × 9 m, in which three plots per cultivar, with 9 plants per plot (.81 m2), were completely randomized.
To study the root angles of each genotype, we used a slightly modified version of the root basket method (Oyanagi et al., Citation1993). The hemispherical baskets had a top and bottom diameter of 15 and 8.6 cm, respectively, and a height of 9.5 cm (Figure (a)). To quantify the numbers of roots that penetrated the mesh, we divided the sides of the baskets into four groups of angles from the horizontal, measured from the center of the basket’s top surface: 0° to 25°, 25° to 45°, 45° to 65° and 65° to 90°. The first two categories represented shallow angles and the last two represented deep angles. The baskets had an internal volume of approximately 1109 cm3 (Figure (a)), and were prepared to have a soil density similar to that in the corresponding compaction treatment before they were placed in the field. After implementing the compaction treatments in the field, we extracted soil samples of similar size to the baskets with a shovel and weighed them; they were approximately 1020 g without compaction (bulk density = .845 g cm−3), 1120 g with moderate compaction (.928 g cm−3), and 1320 g with hard compaction (1.094 g cm−3). The baskets were filled with this weight of soil, and were then inserted into the soil of each treatment in the field, with care not to further compact the soil in the baskets.
Figure 1. Diagrams of (a) the size and geometry of the baskets used in the root basket method for counting root numbers from 0° to 45° (shallow angles) and 45° to 90° (deep angles); (b) a seedling raised in a nursery cell tray and transplanted into a basket in the field at one of the three soil compaction levels (no compaction, moderate compaction, and hard compaction); and (c) placement of the basket and the position of the corers used to obtain samples at depths of 0 to 30 cm and 30 to 60 cm.
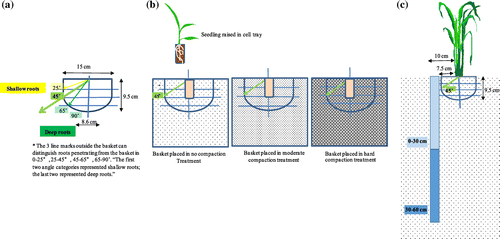
Planting conditions
In 2013, the seeds were sown on 2 May in nursery cell trays, in which each cell had a volume of 13.44 cm3. The young seedlings and surrounding soil were carefully transplanted into the field on 5 July (64 days after sowing; DAS). The long nursery period was chosen to ensure strong establishment of IR64, since it was not clear whether IR64, a semi-dwarf lowland-adapted cultivar, could be transplanted into the upland fields at a younger seedling stage. In 2014, the seeds were sown on 28 April and transplanted on 3 June (36 DAS), when either the 7th leaf (IR64, Dro1-NIL) or the 5th leaf (Kinandang Patong) was expanding, stages at which the 2013 preliminary study demonstrated that seedlings could be safely transplanted into the upland fields. In 2013, the hill space was 20 cm × 20 cm (25 hills m−2) in the main plot, but the baskets were installed at 30 cm × 30 cm (11 hills m−2). In 2014, it was 30 cm × 30 cm, with one seedling per hill. During transplanting, the smallest possible hole was dug to a depth of 4 to 5 cm, just large enough to hold a single cell from the nursery tray, and the soil of the cell, with one seedling, was stabilized within the field soil with gentle manual compression of the soil. For planting of the baskets, a hole with sufficient space for a single seedling was created at the center of the basket (Figure (b)). Sufficient irrigation was supplied just after transplanting to support plant establishment.
Growth conditions
In 2013, all fertilizers were applied basally on 7 May (5 DAS) (6 g m−2 of N, 9 g m−2 of P2O5 equivalent, 8 g m−2 of K2O equivalent, and 100 g m−2 of Ca2SiO4). In 2014, the fertilizers were applied on 29 May (32 DAS), at slightly higher rates (8.2 g m−2 of N, 12.2 g m−2 of P2O5 equivalent, and 10.9 g m−2 of K2O equivalent), without Ca2SiO4.
Minimum and maximum daily air temperatures, total daily solar radiation, and daily rainfall (Figure ) were measured by a weather station (WatchDog 2900ET, Spectrum Technologies Inc., Aurora, IL, USA) installed at the side of the field. The daily minimum and maximum air temperatures were 21.3 ± 3.2 °C (mean ± standard deviation) and 30.5 ± 4.2 °C, respectively, in 2013, and 20.1 ± 3.1 °C and 28.4 ± 4.2 °C, respectively, in 2014. Daily solar radiation totaled 16.3 ± 6.4 MJ m−2 in 2013 and 15.1 ± 7.5 MJ m−2 in 2014. Total seasonal rainfall equaled 636 mm in 2013 and 945 mm in 2014. Average daily evapotranspiration calculated from Penman–Monteith method were 3.36 and 3.49 mm d−1 in 2013 and 2014, respectively, and net water balance were 1.53 and 4.26 mm d−1 in 2013 and 2014, respectively, with larger surplus of water in 2014.
Measurements
In 2014, the soil resistance to penetration was measured with a cone penetrometer (DIK-5,521, Daiki Rika Kogyo Co., Ltd., Saitama, Japan) on four occasions in each of the three compaction treatments during the crop growth period. Soil strength on 30 May (33 DAS) at a depth of 5 to 10 cm was 1 MPa with hard compaction, .5 MPa with moderate compaction, and 0 MPa with no compaction (just after rototilling) (Figure (a)). The soil strength remained highest in the hard compaction treatment, followed by moderate compaction, and the difference increased over time, although the difference between the moderate and no compaction treatments was small, particularly at the end of the growing season (Figure (b) and (c)). The highest level of soil strength was 1.8 MPa at a depth of around 10 cm in the hard compaction treatment on 21 August (116 DAS) and 7 October (163 DAS).
Figure 3. Profile of soil strength (resistance to penetration) in the three soil compaction treatments on (a) 30 May (33 DAS), (b) 21 August (116 DAS), and (c) 7 October (163 DAS) 2014. Bars indicate standard error.
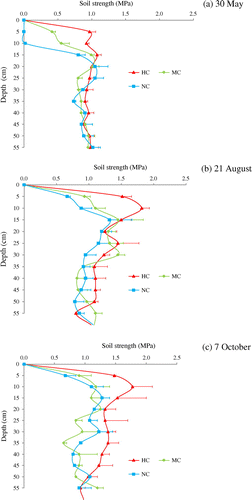
Figure 4. Time course of soil-water potential in no compaction (NC), moderate compaction (MC) and hard compaction (HC) treatments from 26 June to 6 October 2014.
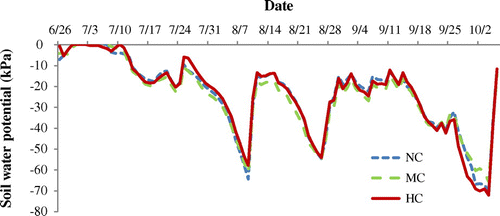
Soil volumetric water contents in the 2014 experiment were measured by a time-domain reflectometer (TDR, FieldScout TDR 300 moisture meter, Spectrum technologies, Inc., Aurora, Illinois) on 9 August (104 DAS), 21 August (116 DAS), and 17 September (163 DAS) for all the three compaction treatments and averaged for two depths, 17% ± 1.4 (mean ± standard deviation), 16% ± 2.0, 19% ± 1.5% by 12 cm rod, and 23% ± 2.5, 22% ± 3.1, 25% ± 2.4 by 20 cm rod, for no compaction, moderate compaction and hard compaction treatments, respectively. Soil water potential at 5-cm depth was monitored every 1 h by tensiometer (WaterMark, Spectrum Technologies Inc., Aurora, IL, USA) connected to the weather station from 7 June to 30 September 2013, and for each compaction treatment from 26 June to 6 October 2014. The average values and standard deviation were −41.7 ± 25.6 kPa in 2013, and −23.9 ± 17.4, -25.1 ± 16.6 and −23.9 ± 18.1 kPa in no compaction, moderate compaction, and hard compaction treatments, respectively, in 2014 (Figure ).
Figure 5. Profile of root length density (RLD) at a depth from 30 to 60 cm for the three genotypes (IR64, Dro1-NIL, and Kinandang Patong [KP]) on 19 October (126 DAS) 2013. IR64 and Dro1-NIL were significantly different (t-test, n = 3, p < .05); KP could not be compared with the other genotypes because it was not replicated. Values are mean ± standard deviation.
![Figure 5. Profile of root length density (RLD) at a depth from 30 to 60 cm for the three genotypes (IR64, Dro1-NIL, and Kinandang Patong [KP]) on 19 October (126 DAS) 2013. IR64 and Dro1-NIL were significantly different (t-test, n = 3, p < .05); KP could not be compared with the other genotypes because it was not replicated. Values are mean ± standard deviation.](/cms/asset/d2bd0716-5b45-427b-9c7f-bbd9d5fb8648/tpps_a_1288550_f0005_b.gif)
We counted the roots and stems in each replicate on 19 September (140 DAS) in 2013 and on 23 July (86 DAS) (maximum tillering) and 6 October (162 DAS) (maturity) in 2014. We calculated the proportion of the total number of roots that emerged from the basket at shallow and deep angles.
We investigated root profiles to a depth of 60 cm on 19 October (170 DAS) 2013 and 2 September (128 DAS) 2014 using a linear soil core sampler 50 mm in diameter and 30 cm long (DIK-110C, Daiki Rika Kogyo Co., Saitama, Japan). After removing the core obtained from a depth of 0 to 30 cm, we excavated the surrounding soil to that depth, and then obtained an additional core from 30 to 60 cm in depth. The core samples were taken at a position 10 cm from the hills. Three replicates were obtained for each genotype under each compaction treatment in 2014; only one sample was obtained for Kinandang Patong in the 2013 experiment.
In both years, the soil cores from both depths were washed carefully (either immediately after sampling or after storage at −4 °C) in a 1-mm steel mesh sieve to separate roots from the soil. It took four or five cycles of cleaning to remove and separate the roots from the soil and two or three washes to separate them from decayed shoots. The cleaned roots were spread on a plate, and the images were scanned on a root scanner (WinRHIZO, Regent Instruments Inc., Quebec, QC, Canada) at a resolution of 400 dpi and with a pixel threshold value of 150 to 190. We then calculated the total root length in each sample using the manufacturer’s software and classified the lengths into categories of <.1, .1 to .2, .4 to .8, .8 to 1, 1 to 1.5, 1.5 to 2, 2 to 2.5, and >3.0 cm. We calculated the root length density (RLD) in each core as the total root length divided by the volume of each core (calculated as a cylinder).
Statistical analysis
Data were analyzed in Genstat statistical software v. 16.0 (https://www.vsni.co.uk/). Analysis of variance (ANOVA) was conducted to examine the effects of genotype according to a randomized block design in 2013. In 2014, with soil compaction effect as main plot and genotype as sub-plot, ANOVA was conducted according to a split-plot design. ANOVA was conducted separately for IR64 and Dro1-NIL to test for the genotype × compaction interaction, and was tested again for all three genotypes to identify genotypic differences.
Results
Root growth angle
In 2013, Dro1-NIL had significantly more stems and marginally more roots than Kinandang Patong. Although Kinandang Patong had the fewest stems and roots, it had a significantly higher proportion of roots (.53) with a deep angle (Table ). Dro1-NIL had a similar number of roots with a shallow root angle to IR64 (147 and 165, respectively), but had more roots with a deep angle (79 and 24, respectively). In both the 45° to 65° and 65° to 90° categories, Dro1-NIL had more roots than IR64 (data not shown). It also had a significantly higher proportion of roots with a deep angle (.35) than IR64 (.13). Based on the average values for the three genotypes, 66% of the total roots had root angles of <45° from the horizontal and 34% had root angles of ≥45°.
Table 1. Total numbers of roots in the two growth angle categories (0° to 45° and 45° to 90° from the horizontal) and proportions of the total in these categories for IR64, Dro1-NIL, and Kinandang Patong on 19 September (140 DAS) (2013 (mean ± standard error). We calculated the least-significant difference at p < .05 (LSD.05).
In 2014, averaged across all three genotypes, the number of stems increased from 22 to 40 and the total number of roots increased from 171 to 244 from maximum tillering (Table (a)) to maturity stages (Table (b)). At the maximum tillering stage, plants in the no compaction treatment had significantly fewer roots than plants in the hard compaction treatment, which did not differ significantly from the moderate compaction treatment (Table (a)). Plants in the moderate and hard compaction treatments had more roots with a shallow angle than plants in the no compaction treatment, significantly so for plants in the hard compaction treatment. The no compaction and hard compaction treatments had the fewest roots with a deep angle, as well as the fewest roots with an angle of 65° to 90°. The no compaction and moderate compaction treatments had a significantly higher proportion of roots with a deep angle than the hard compaction treatment. There was no significant genotypic variation in the total root number, but the number of roots with a deep angle and its proportion of the total differed significantly among the genotypes: both values were highest in Kinandang Patong (67 and .46, respectively), followed by Dro1-NIL (45 and .25) and IR64 (21 and .12). The genotype × compaction interaction was significant only for the number of roots with a deep angle, which was higher in Kinandang Patong than in IR64 and Dro1-NIL in the hard compaction treatment, but was comparable between Dro1-NIL and Kinandang Patong in the other treatments. In the comparison between IR64 and Dro1-NIL, the genotype × compaction interaction was significant only for the proportions of roots with a shallow or deep angle; Dro1-NIL had larger proportion of deep root values than IR64 in the no compaction and moderate compaction treatments, but similar values in the hard compaction treatment.
Table 2. Root numbers and proportions of the total for the two growth angle categories (0° to 45° for shallow angles, 45° to 90 for deep angles) for IR64, Dro1-NIL, and Kinandang Patong in the three compaction treatments: no compaction, moderate compaction, and hard compaction (mean ± standard error). We calculated the least-significant difference at p < .05 (LSD.05). (a) 23 July 2014 (around maximum tillering).
At maturity (Table (b)), genotypic variation in root traits generally resembled that at the maximum tillering stage, with larger values of the deep root angle traits in Kinandang Patong, and the lowest values in IR64. Compaction effects were less than those at the maximum tillering stage and were no longer statistically significant, but the genotype × compaction interaction for shallow and deep root angles was significant in IR64 and Dro1-NIL.
Root length density
In 2013, Dro1-NIL had significantly higher RLD than IR64 at a depth of 30 to 60 cm (Figure ). In 2014, Dro1-NIL and Kinandang Patong had higher RLD than IR64 a depth of 30 to 60 cm in all three compaction treatments, significantly so in the no compaction treatment and for Kinandang Patong in the hard compaction treatment (Figure ). In 2013, the proportions of RLD throughout the soil profile (to a depth of 60 cm) accounted for by RLD at a depth of 30 to 60 cm were 3.2% in IR64, 7.9% in Dro1-NIL, and 11.7% in Kinandang Patong. In 2014, the corresponding values (for all compaction treatments combined) were 2.2, 6.4, and 9.7%, respectively. RLD from 30 to 60 cm in the moderate compaction treatment was 1.35 times the value in the no compaction treatment and .7 times the value in the hard compaction treatment. The proportions of RLD throughout the soil profile (to a depth of 60 cm) accounted for by RLD at a depth of 30 to 60 cm were 8.8% in the no compaction treatment and 8.7% in the moderate compaction treatment, but decreased to 5.3% in the hard compaction treatment.
Figure 6. Comparison of the root length density (RLD) at a depth from 30 to 60 cm among the three genotypes (IR64, Dro1-NIL, and Kinandang Patong [KP]) in the three soil compaction treatments: no compaction, moderate compaction, and hard compaction. Data were obtained on 2 September (128 DAS) 2014. Values are mean ± standard deviation (n = 3). For a given compaction treatment, bars labeled with the same letter differ significantly (p < .05, Tukey’s LSD test.
![Figure 6. Comparison of the root length density (RLD) at a depth from 30 to 60 cm among the three genotypes (IR64, Dro1-NIL, and Kinandang Patong [KP]) in the three soil compaction treatments: no compaction, moderate compaction, and hard compaction. Data were obtained on 2 September (128 DAS) 2014. Values are mean ± standard deviation (n = 3). For a given compaction treatment, bars labeled with the same letter differ significantly (p < .05, Tukey’s LSD test.](/cms/asset/98d9c5a6-ca02-4b23-b1bb-5f6557a19c1b/tpps_a_1288550_f0006_b.gif)
Figure 7. (a) Relationships between the number of roots around maximum tillering and at maturity for roots with shallow (s) and deep (d) angles in the no compaction (NC), moderate compaction (MC), and hard compaction (HC) treatments. (b) Relationship between proportion of roots with a deep angle around maximum tillering and root length density (RLD) at a depth of 30 to 60 cm. (c) Relationship between the proportion of roots with a deep angle around maximum tillering and the proportion of total RLD at a depth of 30 to 60 cm. Values are for both 2013 and 2014, for all three rice genotypes (IR64, Dro1-NIL, and Kinandang Patong [KP]) across the three compaction levels in 2014 and in the no compaction treatment in 2013. Only statistically significant linear regression lines are shown. **p < .01; ns, not significant; n = 12.
![Figure 7. (a) Relationships between the number of roots around maximum tillering and at maturity for roots with shallow (s) and deep (d) angles in the no compaction (NC), moderate compaction (MC), and hard compaction (HC) treatments. (b) Relationship between proportion of roots with a deep angle around maximum tillering and root length density (RLD) at a depth of 30 to 60 cm. (c) Relationship between the proportion of roots with a deep angle around maximum tillering and the proportion of total RLD at a depth of 30 to 60 cm. Values are for both 2013 and 2014, for all three rice genotypes (IR64, Dro1-NIL, and Kinandang Patong [KP]) across the three compaction levels in 2014 and in the no compaction treatment in 2013. Only statistically significant linear regression lines are shown. **p < .01; ns, not significant; n = 12.](/cms/asset/2f832517-8758-4da2-b08e-51711e011c65/tpps_a_1288550_f0007_oc.gif)
In the surface layer, to a depth of 30 cm, there were no significant differences in RLD among the three genotypes, with values of 1.58 ± 1.16 in 2013 in the no compaction treatment in 2013, versus 2.45 ± 1.01 (no compaction), 2.65 ± 1.03 (moderate compaction), and 2.47 ± .93 (hard compaction) in 2014. The genotype × compaction interaction was not significant for RLD in this layer. Dro1-NIL had significantly longer RLD at a depth of 30 to 60 cm than IR64 in the no compaction treatment, but its value in the hard compaction treatment did not differ significantly from those of IR64 and Kinandang Patong (e.g. Figure ), with a significant genotype × compaction interaction for RLD at a depth of 30 to 60 cm.
Relationship between root growth angle and root length density
Table summarizes the correlations (Pearson’s r) between root number and RLD at 0 to 60, 0 to 30, and 30 to 60 cm, and the proportion of total RLD at 30 to 60 cm. Total root number was not significantly correlated with any of the RLD parameters at maximum tillering or maturity. The number of roots with a shallow angle was significantly correlated only with the proportion of total RLD at 30 to 60 cm at both growth stages. On the other hand, the number of roots with a deep angle was significantly correlated both with RLD at 30 to 60 cm and with its proportion of the total RLD at both maximum tillering and maturity, with a stronger correlation at maximum tillering. The proportion of roots with a deep angle was also strongly and significantly correlated with RLD at 30 to 60 cm and with its proportion of total RLD at both stages. The numbers of roots with a shallow angle at maximum tillering and maturity were not significantly related, but the numbers of roots with a deep angle at these stages were significantly related across the nine genotype × compaction treatment combinations (Figure (a)). RLD (Figure (b)) and the proportion of RLD (Figure (c)) at 30 to 60 cm were both significantly related to the proportion of roots with a deep angle across all genotype × treatment combinations, but the relationship was much stronger for the proportion.
Table 3. Values of Pearson’s correlation coefficient (r) for the relationships between root length density (RLD) measured by core sampling and root number and angle measured in 2014 by the basket method around (a) maximum tillering and (b) maturity.
Discussion
This study conformed the effectiveness of the root basket method to distinguish root growth angle of genotypes known to be different (i.e. IR64 vs Kinandang Patong) under upland fields at maturity stage as was shown at earlier growth stages (cf. Kato et al., Citation2006; Uga et al., Citation2013a) and under different levels of soil strength. Magnitude of the difference in the proportion of roots with a deep angle was maintained at similar size at maturity stage (Table ) compared with tillering stage (Table (a)) between the field-grown deep rooting Kinandang Patong and shallower rooting IR64. We discuss below firstly the effects of DRO1 under different levels of soil compaction, and secondly the extent of contribution of a deep root angle to the prevalence of deep roots (i.e. to the root length density in deep soil layers).
Effects of DRO1 on root growth angle and deep root length under different soil compaction treatments
Our study represents the first assessment of Dro1-NIL under upland field conditions with different levels of soil compaction. We found a significant gene × compaction treatment interaction for DRO1; Dro1-NIL had significantly more roots with a deep growth angle and a significantly higher proportion of the total number of roots with a deep angle than IR64 (Tables and ), but Dro1-NIL decreased proportion of roots with a deep root angle and RLD at a depth of 30 to 60 cm in the hard compaction treatment (with its soil strength of 1.8 MPa at around 10 cm in depth (Figure (b) and (c)) and with relatively favorable soil moisture environment in 2014 (Figure )) more than IR64, with their difference becoming insignificant (Table , Figure ). Deep rooting in cereals is determined by both the root growth angle and root elongation (Araki et al., Citation2002); root growth angle is a constitutive trait, but root elongation is greatly affected by differences in soil strength. In rice, an increase in soil penetration resistance from .1 to 1.5 MPa in the field decreased RLD by 40% (Hasegawa et al., Citation1985). Genetic control of root growth has been shown to interact strongly with soil properties (e.g. Cairns et al., Citation2009). Our results indicate that an accumulation of genes or QTLs other than DRO1 for root elongation or for penetration under strong soil resistance would be essential to let breeders manipulate the rice root system to promote growth into deeper soil layers and improve resource acquisition when hard soil layers would be present in the target fields. Since RLD in the deepest layer and the proportion of roots with a deep angle were consistently larger in Kinandang Patong than in IR64, even in the hard compaction treatment, such genetic manipulation appears to be possible. Genetic variation in root penetration as well as QTLs for penetration resistance have been reported in rice (e.g. Clark et al., Citation2002; Price et al., Citation2000; Ray et al., Citation1996).
Relationship between roots with a deep growth angle measured using the basket method and actual deep root length density measured in the field by the core sampling method
Our results show that the proportion of roots with a deep angle around maximum tillering and at maturity measured by the basket method were strongly positively correlated with the root length below 30 cm measured at maturity by means of core sampling. This was the case for our three genotypes across 2 years under upland field conditions with three levels of soil resistance to penetration (Figure (b) and (c); Table ). This is somewhat surprising, as we initially thought that roots passing through the basket at a deep angle (45° to 90°) might be unable to penetrate below 30 cm under hard soil compaction because of impedance of root elongation by the soil’s physical pressure. The difference from the expected results was probably caused by the measurement technique used in the basket method, which counts the numbers of roots that penetrated the basket at ca. 9.5 cm from the base of the plant (Figure ); these numbers reflect both inherent vertical root growth and the effects of reduced elongation due to the compaction. The strong positive correlation based on 2 years of data means that whether researchers use the root basket method or the root core sampling method, they should be able to identify rice phenotypes with deep root development equally well, at least at a soil strength of up to 1.8 MPa. The number of roots with deep angles measured around maximum tillering and at maturity showed a similar tendency. As the measurement of root growth angles by the basket method requires much less labor than the core sampling method, our study demonstrates the usefulness of the basket method for identifying deep root development in rice, at least for the IR64 and Kinandang Patong genetic material.
The baskets used in our experiment were slightly less than 10 cm deep and were 15 cm in diameter, but despite this small size, the technique successfully predicted the differences in RLD at a depth of 30 to 60 cm among the three rice accessions. Considering the labor required for excavating soil and obtaining core samples to a depth of 60 cm, as well as the tedious task of washing the roots, the root basket method can provide an efficient and useful alternative. Even smaller root baskets can be used; for example, the diameter and depth of the baskets used by Uga et al. (Citation2013a) were nearly half of those in our study. Using smaller baskets for seedlings at younger growth stages (i.e. 5 to 7 weeks after sowing) would be an efficient way to phenotype large numbers of genetic materials, such as the lines in a mapping population; Table summarizes recent studies of root growth angles that used the basket approach. However, it will be necessary to confirm the relationship between root angle and deep root development under a range of field rhizosphere environments to confirm the overall applicability of the basket technique.
Table 4. Summary of previous studies of rice root growth angles.
Table also indicates that recent work to identify genes and QTLs for root growth angle (e.g. DRO1, DRO2, DRO3, DRO4, DRO5) focused mostly on populations derived from the donor parent Kinandang Patong (Kitomi et al., Citation2015; Uga et al., Citation2011, Citation2012, 2013b, 2015). Genotypic variation in root growth angles has been reported in other rice cultivars (Henry et al., Citation2011; Kato et al., Citation2006), including Dular, IRAT109, and Yumenohatamochi. Such cultivars can be used to develop mapping populations to support searches for other genes and QTLs for deep rooting. Genetic dissection of root growth angle should be conducted using additional populations derived from different genetic materials.
Disclosure statement
The authors declare no potential conflicts of interest.
Supplemental data
Supplemental data for this article can be accessed at http://dx.doi.org/10.1080/1343943X.2017.1288550
Funding
This study was supported partly by a Japan Society for the Promotion of Science KAKEN-HI [grant number 23380011] and by SATREPS project (JICA-JST) in Colombia.
TPPS_1288550_Supplementary_material.pptx
Download MS Power Point (982.8 KB)Acknowledgments
We thank the technical staff of the University of Tokyo’s Institute for Sustainable Agro-environmental Services for their support in preparation of the study field, including the soil compaction treatments.
References
- Abe, J., & Morita, S. (1994). Growth direction of nodal roots in rice: Its variation and contribution to root system formation. Plant Soil, 165, 333–337.10.1007/BF00008078
- Arai-Sanoh, Y., Takai, T., Yoshinaga, S., Nakano, H., Kojima, M., Sakakibara, H., … Uga, Y. (2014). Deep rooting conferred by DEEPER ROOTING 1 enhances rice yield in paddy fields. Scientific Reports, 4, 5563. doi:10.1038/srep05563
- Araki, H., Morita, S., Tatsumi, J., & Iijima, M. (2002). Physio-morphological analysis on axile root growth in upland rice. Plant Production Science, 5, 286–293.10.1626/pps.5.286
- Cairns, J. E., Audebert, A., Mullins, C. E., & Price, A. H. (2009). Mapping quantitative loci associated with root growth in upland rice (Oryza sativa L.) exposed to soil water deficit in fields with contrasting soil properties. Field Crops Research, 114, 108–118.10.1016/j.fcr.2009.07.009
- Clark, L. J., Cope, R. E., Whalley, W. R., Barraclough, P. B., & Wade, L. J. (2002). Root penetration of strong soil in rainfed lowland rice: Comparison of laboratory screens with field performance. Field Crops Research, 76, 189–198.10.1016/S0378-4290(02)00039-4
- Dexter, A. R., & Hewitt, J. S. (1978). The deflection of plant roots. Journal of Agricultural Engineering Research, 23, 17–22.10.1016/0021-8634(78)90075-6
- Hasegawa, S., Thangaraj, M., & O’Toole, J. C. (1985). Root behaviour: Field and laboratory studies. In Soil Physics and Rice (pp. 283–396). Manila: International Rice Research Institute.
- Henry, A., Veeresh, R. P. G., Rolando, O. T., Kenneth, L. M., & Rachid, S. (2011). Variation in root system architecture and drought response in rice (Oryza sativa): Phenotyping of the Oryza SNP panel in rainfed lowland fields. Field Crops Research, 120, 204–214.
- Kato, Y., Abe, J., Kamoshita, A., & Yamagishi, J. (2006). Genotypic variation in root growth angle in rice (Oryza sativa L.) and its association with deep root development in upland fields with different water regimes. Plant Soil, 287, 117–129.10.1007/s11104-006-9008-4
- Kitomi, Y., Kanno, N., Kawai, S., Mizubayashi, T., Fukuoka, S., & Uga, Y. (2015). QTLs underlying natural variation of root growth angle among rice cultivars with the same functional allele of DEEPER ROOTING 1. Rice, 8, 16. doi:10.1186/s12284-015-0049-2
- Lipiec, J., Horn, R., Pietrusiewicz, J., & Siczek, A. (2012). Effects of soil compaction on root elongation and anatomy of different cereal plant species. Soil and Tillage Research, 121, 74–81.10.1016/j.still.2012.01.013
- Lynch, J. (2013). Steep, cheap and deep: An ideotype to optimize water and N acquisition by maize root systems. Annals of Botany, 112, 347–357.10.1093/aob/mcs293
- Nguyen, H. T., Babu, R. C., & Blum, A. (1997). Breeding for drought resistance in rice: Physiology and molecular genetics considerations. Crop Science, 37, 1426–1434.10.2135/cropsci1997.0011183X003700050002x
- Norton, G. J., & Price, A. H. (2009). Mapping of quantitative trait loci for seminal root morphology and gravitropic response in rice. Euphytica, 166, 229–237.10.1007/s10681-008-9833-z
- Oyanagi, A. (1994). Gravitropic response growth angle and vertical distribution of roots of wheat (Triticum aestivum L.). Plant Soil, 165, 323–326.10.1007/BF00008076
- Oyanagi, A., Nakamoto, T., & Wada, M. (1993). Relationship between root growth angle of seedlings and vertical distribution of roots in the field in wheat cultivars. Japanese Journal of Crop Science, 62, 565–570.10.1626/jcs.62.565
- Price, A. H., Steele, K. A., Moore, B. J., Barraclough, P. B., & Clark, L. J. (2000). A combined RFLP and AFLP linkage map of upland rice (Oryza sativa L.) used to identify QTLs for root-penetration ability. Theoretical and Applied Genetics, 100, 49–56.10.1007/s001220050007
- Ray, J. D., Yu, L., McCouch, S. R., Champoux, M. C., Wang, G., & Nguyen, H. T. (1996). Mapping quantitative trait loci associated with root penetration ability in rice (Oryza sativa L.). Theoretical Applied Genetics, 92, 627–636.10.1007/BF00226082
- Rich, S. M., & Watt, M. (2013). Soil conditions and cereal root system architecture: Review and considerations for linking Darwin and Weaver. Journal of Experimental Botany, 64, 1193–1208.10.1093/jxb/ert043
- Uga, Y., Ebana, K., Abe, J., Morita, S., Okuno, K., & Yano, M. (2009). Variation in root morphology and anatomy among accessions of cultivated rice (Oryza sativa L.) with different genetic backgrounds. Breeding Science, 59, 87–93.10.1270/jsbbs.59.87
- Uga, Y., Okuno, K., & Yano, M. (2011). Dro1, a major QTL involved in deep rooting of rice under upland field conditions. Journal of Experimental Botany, 62, 2485–2494.10.1093/jxb/erq429
- Uga, Y., Hanzawa, E., Nagai, S., Sasaki, K., Yano, M., & Sato, T. (2012). Identification of qSOR1, a major rice QTL involved in soil-surface rooting in paddy fields. Theoretical Applied Genetics, 124, 75–86.10.1007/s00122-011-1688-3
- Uga, Y., Sugimoto, K., Ogawa, S., Rane, J., Ishitani, M., Hara, N., … Yano, M. (2013a). Control of root system architecture by DEEPER ROOTING 1 increases rice yield under drought conditions. Nature Genetics, 45, 1097–1102.10.1038/ng.2725
- Uga, Y., Yamamoto, E., Kanno, N., Kawai, S., Mizubayashi, T., & Fukuoka, S. (2013b). A major QTL controlling deep rooting on rice chromosome 4. Scientific Reports, 3, 3040. doi:10.1038/srep030401
- Uga, Y., Kitomi, Y., Yamamoto, E., Kanno, N., Kawai, S., Mizubayashi, T., & Fukuoka, S. (2015). A QTL for root growth angle on rice chromosome 7 is involved in the genetic pathway of DEEPER ROOTING 1. Rice, 8, 8.10.1186/s12284-015-0044-7
- Yamagishi, J., Nakamoto, T., & Richner, W. (2003). Stability of spatial variability of wheat and maize biomass in a small field managed under two contrasting tillage systems over 3 years. Field Crops Research, 81, 95–108.10.1016/S0378-4290(02)00213-7
- Yamauchi, A., Kono, Y., & Tatsumi, J. (1987). Quantitative analysis on root system structures of upland rice and maize. Japanese journal of crop science, 56, 608–617.10.1626/jcs.56.608
- Yoshida, S., & Hasegawa, S. (1982). The rice root system: Its development and function. In: Drought resistance in crops with emphasis on rice (pp. 97–114). Los Baños: International Rice Research Institute.