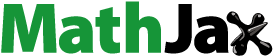
ABSTRACT
Water deficiency is the most prominent constraint for crop production worldwide which is exacerbated by climate change. Developing drought-resistant species is a cost-effective solution to resolve the water lack problems in arid and semi-arid regions. A two-year study was conducted with the aim of developing the cultivation of Lallemantia royleana drought-resistant ecotypes in desert climates. Drought treatments were included control (−0.5 atm), mild (−3.5 atm), moderate (−6.5 atm), and severe (−9.5 atm). Balangu ecotypes were collected from Kalat in Khorasan Razavi province, Zakheh in Kurdistan province, Kondor in Alborz province, and Jupar in Kerman province. The results showed that Kondor and Jupar were drought-resistant ecotypes, and Kalat and Zakheh were drought-sensitive ecotypes. Growth traits, RWC, photosynthetic pigments content, seed yield, seed oil amount, and omega-6 fatty acids contents in all ecotypes decreased in drought conditions. On the contrary, the content of seed mucilage and soluble carbohydrates, the activity of APX, CAT, SOD, and POX, and the amount of phenol and proline increased. However, the drought-tolerant ecotypes produced more dry matter and seed yield under drought conditions. They can use a large amount of photosynthetic energy for biomass and seed production up to −6.5 atm of soil water potential. However, this ability is retained up to −3.5 atm of soil water potential in drought-sensitive ecotypes.
Graphical Abstratct
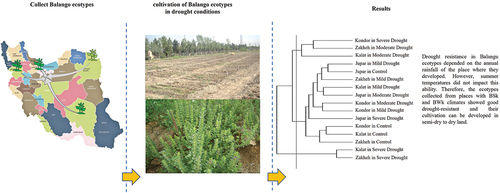
Introduction
Drought is the most prominent environmental constraint for crop production worldwide and is exacerbated by climate change, especially in arid and semi-arid regions (Chai et al., Citation2016). Crop yields are projected to decline by 55 to 70% by the end of the 21st century with drought intensification (Leng & Hall, Citation2019). This will be more critical in arid and semi-arid regions, which cover about 40% of the world’s agricultural land (N Khan et al., Citation2019a). Drought adverse impacts can be mitigated by adopting different approaches such as using drought-tolerant genotypes, changing cultivation patterns to plants that have less water requirement, using some chemicals and biological compounds to improve drought tolerance, etc. (Elgamaal & Maswada, Citation2013). Introducing drought-tolerant genotypes of low water requirement species is the most cost-effective solution to resolve water scarcity problems in arid and semi-arid regions (Ghorbanpour & Varma, Citation2017).
Some medicinal plants are a good candidate for cultivation in semi-arid lands due to their high economic value, drought acclimatization, and low water demand (Ghorbanpour & Varma, Citation2017). Kalaichelvi and Swaminathan (Citation2009) reported that Ashwagandha (Withania somnifera), Kalmegh (Andrographis paniculata), Senna (Cassia angustifolia), Gymnema sylvestre, etc. are appropriate to cultivate in the drylands. Besides, medicinal plant cultivation has recently become widespread due to satisfaction with herbal remedies. It can be an opportunity to address the problem of future drought exacerbation in arid and semi-arid regions. However, the economic development of medicinal plants in those regions requires introducing drought-resistant genotypes with high yields.
Drought stress causes plants a set of morpho-physiological, biochemical, metabolism, and molecular changes (Sharma et al., Citation2020). These changes occur to balance the photosynthetic process and increase the plant defense against drought stress by performing a series of physiological/biochemical adjustments. These events ultimately limit water losses and increase water use efficiency (Kapoor et al., Citation2020). Plants use two different strategies including avoidance and tolerance to cope with drought stress. Plants avoid drought by reducing the vegetative growth stage, increasing water storage, morphological changes (such as leaf rolling, shoot reducing, and boosting root architecture), reducing the growth stage, regulating metabolism, etc. Drought tolerance is obtained through some physiological, biochemical, and molecular mechanisms like stomata closure, boosting antioxidant systems, hormonal balance, plasma membrane protection, gene overexpression, osmolytes accumulation, and aquaporin synthesis stimulation (Cheng et al., Citation2018; S-H. Zhang et al., Citation2018a). However, the strength of drought avoidance and tolerance is varied in different genotypes of a species. These abilities are deeply rooted in evolution which is controlled by ecological, genetic, and epigenetic factors (Anderson et al., Citation2011). Dos Santos et al. (Citation2019) showed that resistant clones of rubber trees (Hevea brasiliensis) possessed better growth and oxidative metabolism under drought. Another study revealed that drought-tolerant thyme (Thymus serpyllum Serpolet) had more growth, photosynthesis pigment amount, proline content, and antioxidant enzyme activity than drought-susceptible thyme (Thymus Vulgaris L.; Mohasseli & Sadeghi, Citation2019). Ahrar et al. (Citation2017) showed that the Bulgarian ecotype (BG) of Arundo donax as drought-adapted was specified by higher photosynthesis and water use efficiency under well-watered conditions. However, the photosynthesis and biochemistry factors limited CO2 diffusion under drought conditions in BG and in Italian ecotype as drought susceptible, respectively. Besides, photosynthesis recovery in the BG ecotype was more rapid following the drought period pass.
Balangu (Lallemantia royleana Benth.) is an annual medicine herb of the Lamiaceae family, which naturally has been distributed in various parts of Iran. However, it is cultivated in other Asian and European countries such as Pakistan, Afghanistan, Turkmenistan, Turkey, and northern India (Koohdar et al., Citation2019). This plant is used in traditional medicine for the treatment of respiratory problems, pneumonia, rheumatism, intestinal troubles, heartburn, fever, arthritis, joint pain, and abscesses inflammations. The seed, leave, and root of this plant have healing properties. The seeds are a good source of fiber, protein, oil, and mucilage (A. Khan et al., Citation2019b). Besides, the presence of phenolic, flavonoid, and tannin compounds has also been reported in Balangu (A. Khan et al., Citation2019b; Razavi & Moghaddam, Citation2011).
Balangu grows in various climatic conditions in Iran, which indicates the high level of developmental and biochemical plasticity in its populations. Hence, identifying resistant and compatible genotypes with harsh climatic conditions helps to develop its cultivation in arid and semi-arid regions. Therefore, phytochemical, morpho-physiological, and biochemical responses to drought were studied on different populations collected of Balangu.
2. Materials and methods
2.1. Filed location and treatments
A two-year experiment was conducted in a split-plot based on a randomized complete block design with three replicates in 2015 and 2016. The weather data for both years of the research farm at Shahed University, Tehran, Iran (35° 34ʹ N and 51° 8ʹ E, 1190 m above sea level) are presented in . Experiment treatments were four levels of drought arranged to the main plot and four Balangu ecotypes ordering to the subplot. Drought treatments included control (field capacity), mild, moderate, and severe. The soil water potential in the drought treatments adjusted to −0.5, −3.5, −6.5, and −9.5 atmospheres, respectively. The soil water potential was determined by Equitensiometer equipment (EQ3 connected to GP2 data logger, Delta-T Devices Company) installed at 30 cm depth of the main plots. One data logger and four sensors were allocated for each drought treatment (main plot). Then the average change of soil water potential was recorded daily and used to adjust irrigation intervals. The drought treatments were imposed from the flowering onset (June 1 to 10). All plots were irrigated at the field capacity before the drought started (every 7 to 10 days). Irrigation operation was performed in furrows form and the soil depth was wetted up to 50 cm every time. Balangu ecotypes were collected from Kalat in Razavi Khorasan province, Zakheh in Kurdistan province, Kondor in Alborz province, and Jupar in Kerman province ().
Table 1. Specifications of Balangu ecotypes collection areas.
2.2. Field practices, and sampling
The farm was prepared by plowing, disk operations for crushing large lumps, leveling the surface, and adding chemical fertilizer consisting of 150 kg ha−1 urea and 100 kg ha−1 triple superphosphate. The experimental plots were created in 2 × 3 m dimensions in March 2015 and 2016. The plots contained 10 cultivating rows at 30 cm intervals. Balangu seeds were sown on the row with a 20 cm spacing on 15 March 2015. The seeds required for planting in 2016 were obtained from each plot in 2015. Soil’s physical and chemical properties were analyzed before the farm preparation in both years (). Sampling was carried out 12–24 hours before the final irrigation. Seven samples were randomly collected from each plot. However, the sampling coincided with the physiological ripening of at least 70% of the seeds. Some samples were immediately frozen in liquid nitrogen and stored at −80°C for biochemical analysis. The others were dried under the shade to determine morphological characteristics.
Table 2. Soil physicochemical properties of experimental farm at 2015 and 2016.
2.3. Plant growth characteristics
Plant height, leaf number, plant dry weight, and seed yield were measured after transferring samples to the laboratory and drying. Harvest index (HI) was calculated by dividing seed yield by biological yield.
2.4. Relative water content
RWC was measured with the dry immersion method described by Kwasniewski et al. (Citation2016). Fresh leaves were cut into 3 cm segments and recorded as fresh weight (FW). The leaf pieces were immersed in deionized water (10 mL) in a petri dish and were kept in darkness overnight. The swollen leaf pieces were then removed from the water and weighted as turgid weight (TW). The leaf pieces were dried at 70°C until reaching a constant weight and recorded as dry weight (DW). The RWC was calculated as the following equation:
2.5. Seed oil extraction and GC analysis
The oil of ground seeds (50 g) was extracted with 150 mL petroleum ether as a solvent in a Soxhlet apparatus for 4 h. Then, the solvent was removed by a rotary evaporator at 40°C. The oil content was expressed as a percentage of the seed’s weight (Tan & Muhamad, Citation2017). Fatty acid methyl esters were prepared by heating 25 mg oil with 1.5 mL methanolic NaOH 500 mM and 2 mL boron trifluoride-methanol solution 14% in a boiling water bath for 30 min. Fatty acid methyl esters were analyzed on Agilent HP 6890 Series combined with Agilent HP 5973 Mass Selective Detector (GC-MS). Helium as the carrier gas with 1 mL min−1 flow and 20:1 split ratio used in the HP column (60 m length, 0.25 mm ID, 0.2 mm DF). The temperature program included starting from 90◦C to 195◦C with a 25◦C min−1 rate, then increasing to 230◦C and 250◦C with a 15◦C min−1 and 10◦C min−1 rates. The column temperature was 250◦C (Ilyasoğlu, Citation2014). The fatty acids were identified by comparing the mass spectra and retention indices with published literature and the MS computer library. The content of αLA and omega-6 fatty acids was then calculated.
2.6. Mucilage content
To extract the mucilage, 5 g seed was immersed in 100 mL boiling distilled water (pH 5). The mixture was placed in a boiling water bath and shaken for 2 hours. The extract was filtered through Whatman No. 1 and evaporated to 50 mL. To coagulate and sediment the mucilage, 150 mL ethanol (96%) was gradually added to the extract and kept in a refrigerator overnight. The mucilage was separated with filter paper and dried at 40°C for 24 hours. The mucilage content was expressed as a percentage of seed weight (Monrroy et al., Citation2017).
2.7. Phenol content
Phenol content was measured according to the procedure presented by Faurobert et al. (Citation2007). The plant powder was mixed with hot water (20 mL) and placed in a boiling water bath for 1 hour. The extract volume was then increased to 25 mL after passing through filter paper. The extract (1 mL) was reacted with 5 mL Folin- Ciocalteu reagent and 10 mL saturated sodium carbonate (35%) in a 100 mL volumetric flask. The mixture was incubated in the dark at ambient temperature for 45 min. Finally, the mixture absorption was recorded at 750 nm. Phenol content was calculated by standard Gallic acid curve and was expressed as mg g−1 plant dry weight.
2.8. Proline content
Leaf proline was determined by crushing frozen leaf in a pestle and mortar with 10 mL sulfosalicylic acid. The extract was prepared by centrifuging at 13,000 g for 10 min. It was then reacted with equal amounts (2 mL) of Ninhydrin reagent and glacial acetic acid. The reaction was completed by placing the test tube in boiling water for 1 h. Then, toluene (4 mL) was added to the cooled reaction mixture. The absorbance of the red upper layer was read at 520 nm. The proline content was calculated by standard curves and expressed as µM g−1 fresh weight (Bates et al., Citation1973).
2.9. Soluble carbohydrates
The soluble carbohydrates rate was calculated through the method of DuBois et al. (Citation1956). Frozen leaf was homogenized with distilled water in cold conditions. The extract volume increased to 10 mm after passing through filter paper. Then, 1 mL of it was mixed with 5% aqueous phenol and concentrated sulfuric acid in a test tube, respectively. The reaction mixture absorbance was read at 480 nm. The soluble carbohydrate content was calculated by the standard glucose curve and was reported as mg g−1 fresh weight.
2.10. Protein and antioxidant enzyme
2.10.1. Protein
Frozen leaf (0.5 g) was crushed in 5 mL extraction buffer (including 100 mM phosphate buffer, 0.1 mM EDTA, 1 mM ascorbate, and 2% PVP, pH 7). The extract was centrifuged at 13,000 g for 25 min at 4°C. The supernatant was kept in a freezer until measuring protein content and enzymatic activity (Seif Sahandi et al., Citation2019). The reaction mixture to determine soluble protein included the extract, Bradford reagent, and distilled water to 6 mL volume. The mixture absorbance was recorded at 595 nm. Bovine serum albumin was used to draw a standard curve. The soluble protein amount was reported as mg g−1 fresh leaf weight (Bradford, Citation1976).
2.10.2. Ascorbate peroxidase (EC 1.11.1.11)
Ascorbate peroxidase activity was measured by the method of Foyer et al. (Citation1997). The reaction mixture included 50 mM phosphate buffer (pH 7), 1 mM EDTA, 1 mM H2O2, 0.5 mM ascorbate, and extracted enzyme to 3 mL total volume. The reaction began after adding H2O2 at ambient temperature (20°C) and its absorption was read at 290 nm for 3 min. The enzyme activity was expressed based on μM oxidized ascorbate per minute per mg protein (Ɛ = 2.8 mM−1 cm−1).
2.10.3. Superoxide dismutase (EC 1.15.1.1)
Superoxide dismutase activity was assayed by Giannopolitis and Ries (Citation1977). The reacting mixture consisted of 50 mM phosphate buffer (pH 7.8), 0.1 mM EDTA, 50 mM sodium carbonate (pH 10.2), 63 µM nitro blue tetrazolium (NBT), 1 μM riboflavin, 12 mM L-methionine, and an appropriate volume of enzymatic extract. The reaction began with exposure to light and ended by turning the light off after 15 min at ambient temperature (20°C). The reaction mixture absorption read at 560 nm. The blank was the reaction mixture in the darkness. The SOD activity has been expressed as a unit per mg of protein. A unit of SOD was defined as an enzyme amount to inhibit a 50% reduction in the NBT.
2.10.4. Peroxidase (EC 1.11.1)
Peroxidase enzyme activity was determined based on the Wendel (Citation1981) method. The enzymatic reaction consisted of 100 mM phosphate buffer (pH 6.8), 0.25 mL guaiacol (20 g L−1), and 0.25 mL extracted enzyme. The reaction was initiated by adding 5 mM H2O2 at ambient temperature (20°C). The increase in absorbance was read at 470 nm. The blank contained the reaction mixture without the crud enzyme. The enzyme activity was calculated by the extinction coefficient (Ɛ = 26.6 mM−1 cm−1) and was expressed mM per minute per mg protein.
2.10.5. Catalase (EC 1.11.1.6)
The Cakmak and Horst (Citation1991) method was used for catalase activity measurement. The reaction mixture consisted of 25 mM phosphate buffer (pH 6.8), 10 mM H2O2, and extracted enzyme. The decrease in H2O2 adsorption was recorded at 240 nm in ambient temperature (20°C). The blank included the reaction mixture without H2O2. The catalase activity was presented as μM H2O2 decomposed per minute per mg protein (Ɛ = 43.6 mM−1 cm−1).
2.11. Leaf photosynthetic pigments amount
The chlorophyll and carotenoid amounts were measured by Arnon’s (Citation1949) and MacKinney’s (Citation1941) methods. 1 g frozen leaf was crushed by a mortar and pestle with 10 mL of 80% acetone. The homogenate solution was filtered into a 25 mL volumetric flask and washed to form a colorless residual. Then, the absorbance of the solution was measured at 663, 645, 510, and 480 nm, respectively. The photosynthetic pigments amount was calculated by the following equations and reported as mg g−1 fresh weight.
2.12. Statistical analysis
All data were analyzed using SAS 9.4 software. Traits that did not have homogeneous year variances were analyzed separately after confirming by the Bartlett test. Duncan’s multiple range tests were used to compare the main factors with a 5% confidence interval. The Least Square Means procedure was used to compare the interaction of the main factors. The average method and Euclidean distance were used for the cluster analysis. AICc (Akaike Information Criterion corrected for small samples) was used to select the best-fitted regression models.
3. Results
3.1. Balangu growth features
Growth characteristics rates were different in the various ecotypes, which significantly decreased (p ≤ 0.01) in drought conditions (). Kondor had the highest seed yield in the control (on average 1312.5 kg ha−1) and in drought conditions. On the contrary, Jupar had the lowest seed yield in the control and mild drought (on average 460.4 and 421.3 kg ha−1). Besides, the seed yield of Kalat and Zakheh reduced with the same trend in response to increment drought (). Zakheh had the highest plant height (40.7 cm in 2015 and 35.8 cm in 2016), leaf number (240), and plant dry weight (3.42-ton ha−1) in the control. However, Kondor and Jupar had the least reducing leaf number and dry weight in various drought levels (dry weight decrement 28–61% in Kondor and 30–42% in Jupar; ). Besides, the regression model showed that plant height of Kondor and Jupar reduced with a lower slope by increment drought, especially at a severe level (). The greatest HI was recorded for Kalat in the control (on average 38.25%). However, HI increased in all ecotypes with increment drought intensity (). Kondor and Kalat had the highest RWC in the control (on average 72.7% and 69.5%, respectively). However, the reduction of Jupar RWC followed a quadratic equation with a lower slope than in other ecotypes ().
Figure 2. The drought impact on the dry weight (a), seed yield (b), leaf numbers (c), plant height (d), and HI (e) of various Lallemantia royaleana ecotypes. Values are means (n = 6). Circle = Kalat ecotype, triangle = Kondor ecotype, rectangle = Jupar ecotype, and diamond = Zakheh ecotype.
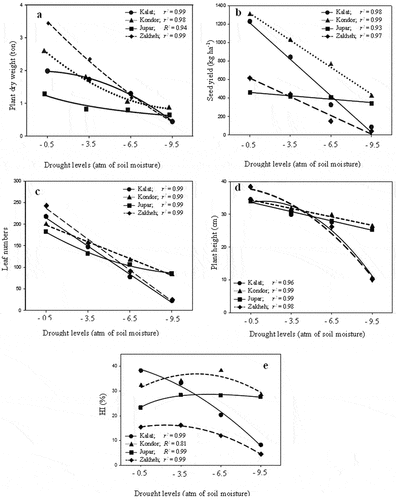
Figure 3. The drought impact on RWC of various Lallemantia royaleana ecotypes. Values are means (n = 3). Means with the same letter have no significant difference at p ≤ 0.05.
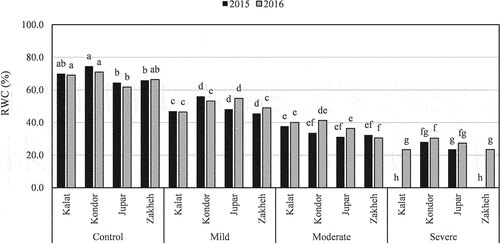
Table 3. Mean comparison of Lallemantia royleana growth features at different drought stress and ecotype.
3.2. Oil, αLA, and omega-6 contents
The content of oil and omega-6 was more in 2016 (18.2% and 13.3%, respectively) than in 2015 (16.8% and 10%, respectively). αLA content had no significant difference between 2015 and 2016. The oil content was reduced under drought conditions in all ecotypes as following a quadratic equation (). Kalat had the highest amount of seed oil in field capacity irrigation. Zakheh had the highest and lowest content of αLA (62.3%) and omega-6 (11.3%), respectively (). There was no significant difference in the amount of omega-6 in Kondor (11.7%) and Jupar (11.69%). The amount of αLA in Jupar (60.5%) and Kalat (59.9%) was also not significantly different. Drought decreased the content of seed oil and omega-6 in different ecotypes. However, αLA content did not significantly change under drought conditions. The seed oil and omega-6 contents slightly decreased in every ecotype under mild drought. However, all ecotypes had higher oil content in mild drought than in severe drought. Also, the content of oil (17%) and omega-6 (11.5%) was identical in moderate and severe drought (16.6% and 11.2% respectively). However, they were significantly lower than the control.
Figure 4. The effect of drought stress on the seed oil content of various ecotypes (a) and omega −6 content (b), and the content change of omega-6 (c) and the α-linolenic acid (c) in different ecotypes in 2015 and 2016. Values are means for seed oil content (n = 6), α-linolenic acid and omega-6 content (n = 3). Different alphabet characters indicate significant differences (p ≤ 0.05), determined by Duncan test.
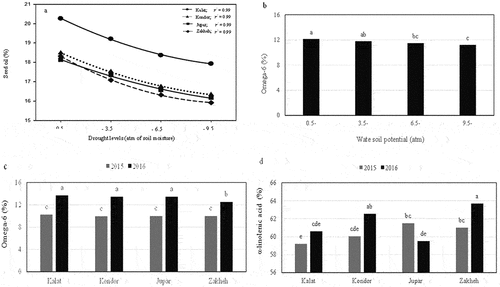
3.3. Protein and mucilage content
The protein and mucilage contents significantly (p ≤ 0.01) changed in the ecotypes under drought conditions. The protein content was altered by a Lorentzian equation along with increasing drought in all ecotypes (). Thus, the protein contents increased with decrement soil water potential up to −4.6 atm in Zakheh, −6 atm in Kalat, −7.2 atm in Jupar, and −9.46 atm in Kondor, and then reduced. Protein synthesis rapidly increased in Zakheh and Kalat by the least water depletion and is delayed in Jupar and Kondor up to severe drought. The mucilage content in Kalat and Zakheh linearly increased as drought intensified (). However, the MC of Kondor increased up to moderate drought (on average 31.18%) and then reduced. Besides, Jupar did not significantly change in MC in drought conditions.
Figure 5. Change of protein (a), phenol (c), and soluble carbohydrate (d) contents in response to drought stresses in the various ecotypes. Values are means (n = 6).
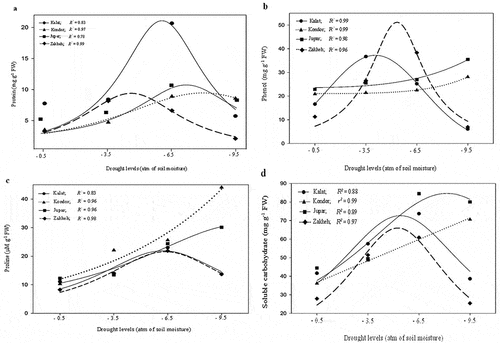
Table 4. Mean comparison of Lallemantia reoyleana biochemical characteristics at different drought stress and ecotype.
3.4. Phenol content
The phenol content increased in the ecotypes with drought intensifying (). The highest phenol content in mild, moderate, and severe drought was recorded for Kalat (36.8 mg g−1 DW), Zakheh (38.4. mg g−1 DW), and Jupar (35.5 mg g−1 DW), respectively. Besides, the lowest phenol content was observed in Zakheh and Kalat in severe drought (6.9 and 6.1 g g−1 DW, respectively). The phenol content of Kalat increased with soil water depletion to mild drought (on average 36.72 mg g−1 DW) and then reduced by 46.6% and 6 times. The maximum phenol content of Zakheh was obtained at −5.35 atm. The phenol content exponentially increased in Jupar and Kandor with increment drought. Thus, its highest amount was observed in severe drought (35.5 and 28.1 mg g−1 DW, respectively).
3.5. Proline and soluble carbohydrate content
The drought significantly (p ≤ 0.01) increased proline and SC contents in the ecotypes. Kondor and Jupar had the highest contents of proline and SC in severe drought (, d). On the contrary, the highest contents of them in Kalat and Zakheh were obtained in moderate drought. The nonlinear regression estimated that Kalat and Zakheh owned the highest proline and SC contents between −5.3 and −5.7 atm of soil water potential. In addition, Kondor and Jupar had the highest proline and SC contents at −8.16 to −9.5 atm of soil water potential.
3.6. Antioxidant enzyme activity
The activity of SOD, CAT, POX, and APX significantly (p ≤ 0.01) increased in every ecotype during the drought. CAT activity in 2016 was higher than in 2015 under drought conditions (). Zakheh and Kalat had higher CAT activity than Jupar and Kondor at any drought level (). Besides, CAT activity in Zakheh increased by a steeper slope than in Kalat. CAT activity in Jupar was lower than other ecotypes in any drought levels and increased by following a Sigmoidal equation. APX activity in Kondor and Zakheh was more than in Kalat and Jupar at every drought level (). However, APX activity in Kondor and Jupar enhanced with more slope than in Zakheh and Kalat in severe drought. SOD activity increment in Kalat and Jupar had an identical trend in response to drought (). Kondor and Zakheh had the highest and the lowest activity of SOD in any drought levels, respectively. POD activity increased in Zakheh, Kalat, and Kondor by drought. In contrast, its activity in Jupar decreased ().
3.7. Photosynthetic pigments
The drought had a significant (p ≤ 0.01) effect on all measured photosynthetic pigments. Water deficit reduced chlorophyll content in all ecotypes and in contrast, increased the Car content (). Kondor and Jupar had the greatest photosynthetic pigments content in filed capacity irrigation. However, this superiority was maintained at all drought levels. Zakheh and Kalat had minimum content of Cha, Chb, and ChT in well-irrigated conditions. The photosynthetic pigments content in Kalat and Zakheh was less than other ecotypes in the moderate and mild drought, respectively. The regression models of ecotypes responses to increasing drought levels showed Cha more dissipated than Chb in Kondor (). However, the loss of Cha and Chb was almost equal in Zakheh. In Kalat, Chb was more resistant to dissipation than Cha in moderate drought. The carotenoid content increase was dependent on drought severity and ecotype (). The carotenoid content in Kalat increased up to mild drought (94.7 mg g−1 FW) and then reduced down to 76.5% in severe drought. The carotenoid amount in Zakheh and Jupar increased up to moderate drought (36% and 3.8 times, respectively) and then decreased (74% and 52%, respectively) at severe drought. Carotenoid content linearly increased in Kondor under drought conditions ().
Figure 7. Photosynthesis pigments content response to drought stress in the various ecotypes. Values are means(n = 6). Circle = Kalat ecotype, triangle = Kondor ecotype, rectangle = Jupar ecotype, and diamond = Zakheh ecotype.
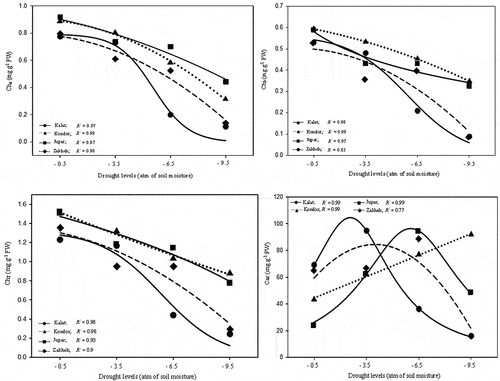
Table 5. Mean comparison of Lallemantia reoyleana pigments at different drought stress and ecotype.
3.8. Principal component analysis and clustering
The first and second components described 66% of the data variance (). Growth traits such as plant height, leaf number, plant dry weight, seed yield, RWC, and the content of photosynthetic pigments, seed oil, and omega-6 had a positive correlation with the first component. In contrast, the second component had a positive correlation with stress-related traits such as antioxidant activity and osmotic adjustment compounds. Zakheh, Kalat, and Kondor were placed in a group when well irrigated. They obtained higher scores for the growth features and lower scores for stress-related traits. Zakheh and Kalat were placed in one group when exposed to severe drought. They had the lowest growth rates and stress-related traits. The treatments were classified into 3 groups by cluster analysis. Besides, traits attributed to growth and seed oil were separated from traits related to drought resistance. Hence, the collected ecotypes were distinguished based on their drought-resistant strength, except Jupar.
4. Discussion
The results showed that all ecotypes in 2016 had a higher growth rate and dry matter accumulation than in 2015. Besides, Kalat and Zakheh could not withstand the severe drought in 2015. However, their next generation was able to tolerate the severe drought in 2016. It seems that a half-degree drop in the monthly average temperature between the sowing and harvesting may have reduced high temperature and drought stress detrimental effects in 2016.
The highest growth characteristics and seed yield in all ecotypes were obtained under suitable irrigation conditions. Thus, Kondor, Zakheh, and Kalat had the highest seed yield, plant dry weight, and seed oil content, respectively. Besides, Kondor had high mucilage content in the field capacity irrigation and drought stress. It was also found that Kalat and Zakheh are drought-sensitive ecotypes, while Kondor and Jupar are drought-resistant ecotypes. Kelat and Zakheh grew in almost identical ecological conditions with sufficient rainfall and lower temperatures. Hence, they showed similar growth characteristics at each drought level and clustered within a group with the least distance. Jupar had lower vegetative traits, seed yield, and oil content than other ecotypes under optimal irrigation and drought stress. However, the reduction of these traits showed resistance to the increased drought intensity and decreased with a lower slope.
The omega-6 content was not significantly different between the ecotypes. The αLA content was higher in Zakheh than in other ecotypes. Drought decreased the seed oil and omega-6 contents in all ecotypes but had no significant effect on the amount of αLA. These results were consistent with other findings (Bayati et al., Citation2020; El Sabagh et al., Citation2019). Joshan et al. (Citation2019) reported that the seed oil content decreased in safflower genotypes (Carthamus tinctorius L.) by drought. Besides, oleic acid content increased and linoleic acid content reduced. Tohidi-Moghadam et al. (Citation2011) showed that the canola oil and linoleic acid contents were reduced in drought conditions, on the contrary, α-linolenic content was raised. Hussain et al. (Citation2018) also stated that water deficit along with high air temperature in the late stages of sunflower development reduced the seed oil quality. Seed oil content reduction in drought conditions can be due to decreasing photosynthesis and assimilation remobilization, as well as the oxidation of some polyunsaturated fatty acids (El Sabagh et al., Citation2019). Besides, it is reported that moderate and severe drought stress enhanced the total saturated fatty acids and reduced the unsaturated fatty acids (Dawood & Sadak, Citation2014). The results revealed that leaf area is reduced by decreasing RWC, and subsequently assimilate accumulation and transfer reduced. Thus, seed production and the content of oil and omega-6 fatty acids are reduced. A positive correlation between seed oil, αLA, and omega-6 contents with plant height, leaf number, RWC, PDW, and seed yield () can confirm this claim. On the contrary, reducing the seed oil content of the ecotypes along with increasing proline content and APX activity or a significant negative correlation between catalase and APX activities with the omega-6 amount can indicate oxidation of some polyunsaturated fatty acids.
Kondor and Zakheh accumulated higher dry matter in field capacity irrigation, while Kondor and Kalat produced more seed yields. The results showed a significant positive correlation between plant dry weight with leaf number, plant height, RWC, Cha, Chb, and ChT (, ). These correlations indicate the role of water availability, light absorption, and photosynthetic capacity in dry weight accumulation of Balangu, which was similar to other plants (Amoah et al., Citation2019; Dos Santos et al., Citation2019). Zakheh accumulated more dry matter with lower chlorophyll content and higher leaf number and plant height amount than other ecotypes in the control. In contrast, Jupar accumulated less dry matter due to fewer leaves, although it possessed high chlorophyll content. Kondor accumulated 81% more dry matter only with 10% more leaves number and identical amounts of height and chlorophyll than Jupar. Thus, it is concluded that leaf number and then plant height are effective factors for dry matter accumulation in Balangu in the field capacity. Kumar et al. (Citation2017) supported that increasing leaf area is more important for increasing dry matter accumulation than plant height in cotton genotypes.
Table 6. Pearson correlation coefficients of measured traits under various irrigation regimes.
Despite higher dry matter production, Zakhe had lower HI than Kalat. It may be due to the high temperatures of Shahed University at the seed set stage. Preite et al. (Citation2015) stated that plants adapted to warm climates produced more seeds during a longer flowering period. Hence, it was concluded that Zakheh is adapted to lower temperatures during the seed formation period and is not suitable for cultivation in places with a BWh climate.
Growth traits including plant height, leaf number, dry matter, and seed yield decreased in the ecotypes exposed to drought. In contrast, osmotic regulating substances and oxidative stress-alleviating compounds increased. Gao et al. (Citation2020) showed a decrease in biomass and an increase in antioxidant enzyme activity and osmotic regulation compounds in two Adonis species under drought conditions. Drought alters plant growth and development via impact on physiological and biochemical processes. However, the extent of these changes depends on the intensity and duration of drought, and the plant genotype (Chadha et al., Citation2019). Water deficiency has an adverse effect on light absorption, net photosynthesis rate, the strength of sink and source, etc. Besides, it has been reported that a decrement in cell turgor pressure resulted to reduce cell division and enlargement (Chadha et al., Citation2019). Therefore, genotypes that are able to retain water in drought conditions will have higher leaf area and plant height. In addition, CO2 access and net photosynthesis be improved due to remaining stomatal open and reducing stomatal conductance (Osakabe et al., Citation2017). The obtained results showed the positive correlation between RWC with leaf number (r = 0.97, p ≤ 0.01) and plant height (r = 0.89, p ≤ 0.01). Hence, higher RWC in Kondor helps it to have more leaf numbers and height. Jupar is compatible with a lower RWC because it had the lowest amount of RWC even in the control. Besides, it produced the highest amount of soluble carbohydrates and proline in severe stress and was able to retain more water.
The ability to prevent degradation of chlorophyll and carotenoids under drought stress was another factor for more production of dry matter and seed yield in Kondor and Jupar. Furthermore, a positive correlation was found between chlorophyll content with RWC (r = 0.8, p ≤ 0.01), which indicated water retention helps protect chlorophyll in Kondor and Jupar in severe drought. However, Kondor was more able to maintain Chb and Jupar was more capable of Cha protection. Drought reduced the chlorophyll content by increasing damage to thylakoid membranes and chlorophyllase activity (Fahad et al., Citation2017). T. Zhang et al. (Citation2018b) showed that chlorophyll amount in Ricinus communis reduced under drought conditions and chlorophyll a was more susceptible than chlorophyll b. Moreover, Toscano et al. (Citation2016) was found a negative correlation between chlorophyll b amount and RWC in Eugenia uniflora under drought conditions.
The amount of proline and soluble carbohydrates in all ecotypes increased with increasing drought intensification. Other studies were reported the drought impact on increasing osmose protectant compounds (Cheng et al., Citation2018; T. Zhang et al., Citation2018b). Kondor and Jupar had the highest amount of proline and soluble carbohydrates in severe drought, while Zakheh and Kalat acquired the maximum rate in moderate drought. Also, there was a negative correlation between RWC with the amount of proline (r = −0.77, p ≤ 0.01) and soluble carbohydrate (r = −0.71, p ≤ 0.01) in 2016, which indicated osmolyte adjustment to water depletion. The result is confirmed by Toscano et al. (Citation2016), which found a negative correlation between proline content and RWC. Therefore, it can be described that Kondor is powerful in increasing osmolyte solutes and hence, is able to retain more water content.
Drought increased the protein content in all ecotypes up to moderate drought except Zakheh. Protein amount increment under drought stress was reported by some authors (Gao et al., Citation2020; Toscano et al., Citation2016). Protein content in Kalat sharply increased in moderate drought, while its content in other ecotypes strongly increased in mild drought. There was a positive correlation between protein content with the activity of SOD and POX and soluble carbohydrate content. Park et al. (Citation2021) confirmed the role of protein increment in boosting antioxidant potential and the hormone regulation for the degradation of sucrose and starch under drought stress.
The change of mucilage content was dependent on the ecotypes. So, its content increased in Kondor, Kalat, and Zakheh under drought conditions and did not change in Jupar. Shahriari et al. (Citation2018) reported that the mucilage content of Plantago species slightly changed in drought stress. Mucilage is a secondary metabolite that is composed of complex polysaccharides. These compounds are able to absorb vast water amounts and improve seed germination and seedling growth (Teixeira et al., Citation2020). Therefore, it concluded that the metabolic pathway in the seed coat cells has shifted to the production and accumulation of mucilaginous compounds under drought stress. This is a natural response to acclimatization to drought conditions and could increase next-generation survival (Teixeira et al., Citation2020).
Oxidative stress is a consequence of free radicals bursting under drought conditions and leads to lipid peroxidation and the oxidation of proteins and DNA/RNA molecules (Park et al., Citation2021). ROS generation takes place in different cell compartments and first transmits signals to the nucleus and other organelles to reprogram cell functions including gene expression (Laxa et al., Citation2019). Then, plants withstand free radicals burst by elevating the antioxidant systems (Fahad et al., Citation2017). The collected Balangu ecotypes quenched the free radical via phenolic compounds accumulation and increasing antioxidant enzyme activity. The results of other studies conformed to the findings (Amoah et al., Citation2019; Gao et al., Citation2020). It is reported that APX, SOD, and CAT activities increased in resistant plants to alleviate the damage rate induced by drought (Dawood et al., Citation2014). Gao et al. (Citation2020) reported that drought increased phenol content in Adonis species. The correlation between phenol content with POX activity (r = 0.75, p ≤ 0.01) and APX activity (r = 0.54, p ≤ 0.05) in 2015 proposed the phenol role as a substrate for the enzymatic antioxidant reaction (Gao et al., Citation2020). Besides, a positive correlation between the phenol content with Car amount, SOD activity, and SC value indicated the antioxidant role of phenol in scavenging free radicals. The efficiency of the antioxidant defense system in plants, including ROS scavenging enzymes and non-enzymatic components such as phenol, proline, and carotenoids, can determine the drought adaptation potential. Hence, the consequences of drought stress are minimal for plants adapted to arid and semi-arid regions (Amoah et al., Citation2019).
The results showed that the APX enzyme in Kalat and the POX enzyme in Jupar did not participate in drought tolerance. Laxa et al. (Citation2019) reported that APX activity is commonly enhanced in all species of the plant kingdom exposed to drought, and CAT activity plays a crucial role in the plant tolerance to severe drought stress. fCat activity had an important role to cope with the severe drought. However, all measured antioxidant enzymes were effective in scavenging free radicals in Zakheh. In general, improved cellular turgor pressure and the antioxidant defense system are biochemical mechanisms to cope with drought stress in Balangu ecotypes.
5. Conclusions
Drought-resistant ecotypes were Kondor and Jupar, while drought-sensitive ecotypes were Kalat and Zakheh. The drought-resistant ecotypes can produce more dry matter and seed yield than the drought-sensitive ecotypes under drought conditions. Because, they had more RWC, leaf number, plant height, and chlorophyll content. Kondor and Jupar have grown in semi-desert and desert conditions, so they have good tolerance to drought and high temperatures. They had high RWC under optimal irrigation and drought stress conditions, which was related to high levels of carotenoids, proline, soluble carbohydrates, and antioxidant activity. The drought-resistant ecotypes can use a large amount of photosynthetic energy to produce biomass and seed up to −6.5 atm of soil water potential. However, this ability is retained up to −3.5 atm of soil water potential in the drought-sensitive ecotypes. Thus, phenotypic plasticity, phenological changes, increased antioxidant potency, and water retention ability are components of drought resistance mechanisms in Balangu ecotypes grown in harsh climates.
Abbreviations
APX: Ascorbate peroxidase; BSk: cold arid steppe climate; BWh: Hot arid deserts climate; BWk: cold desert arid climate; Car: Carotenoid; CAT: Catalase; Cha: Chlorophyll a; Chb: Chlorophyll b; ChT; Chlorophyll Total; Csb: Temperate, dry warm summer climate; Dsa: dry hot summer climates; FW: Fresh weight; HI: Harvest index; LN: Leaf number; MC: Mucilage content; PCA: Principal component analysis; PDW: Plant dry weight; PH: Plant height; POX: peroxidase; Pro: protein; RWC: Relative water content; SC: Soluble carbohydrate; SOD: Superoxide dismutase; SY: Seed yield; αLA: α-Linolenic acid.
Acknowledgments
The authors are grateful for the software support provided by Iran National Science Foundation (INSF)’s 90003335 work platform. Omidi is greatly thankful for the companionship and support of MPRC for many years. We also thank Editage for their assistance with language editing.
Disclosure statement
No potential conflict of interest was reported by the author(s).
References
- Ahrar, M., Doneva, D., Tattini, M., Brunetti, C., Gori, A., Rodeghiero, M., Wohlfahrt, G., Biasioli, F., Varotto, C., Loreto, F., & Velikova, V. (2017). Phenotypic differences determine drought stress responses in ecotypes of Arundo donax adapted to different environments. Journal of Experimental Botany, 68(9), 2439–2451. https://doi.org/10.1093/jxb/erx125
- Amoah, J. N., Ko, C. S., Yoon, J. S., & Weon, S. Y. (2019). Effect of drought acclimation on oxidative stress and transcript expression in wheat (Triticum aestivum L.). Journal of Plant Interactions, 14(1), 492–505. https://doi.org/10.1080/17429145.2019.1662098
- Anderson, J. T., Willis, J. H., & Mitchell-Olds, T. (2011). Evolutionary genetics of plant adaptation. Trends in Genetics, 27(7), 258–266. https://doi.org/10.1016/j.tig.2011.04.001
- Arnon, D. I. (1949). COPPER ENZYMES IN ISOLATED CHLOROPLASTS. POLYPHENOLOXIDASE IN BETA VULGARIS. Plant Physiology, 24(1), 1–15. https://doi.org/10.1104/pp.24.1.1
- Bates, L. S., Waldern, R. P., & Teave, I. D. (1973). Rapid determination of free proline for water stress studies. Plant and Soil, 39(1), 107–205. https://doi.org/10.1007/BF00018060
- Bayati, P., Karimmojeni, H., & Razmjoo, J. (2020). Changes in essential oil yield and fatty acid contents in black cumin (Nigella sativa L.) genotypes in response to drought stress. Industrial Crops and Products, 155, 112764. https://doi.org/10.1016/j.indcrop.2020.112764
- Bradford, M. (1976). A rapid and sensitive method for the quantitation of protein utilizing the principle of protein-dye binding. Annual Review of Biochemistry, 72(1–2), 248–254. https://doi.org/10.1016/0003-26977690527-3
- Cakmak, I., & Horst, W. (1991). Effect of aluminum on lipid peroxidation, superoxide dismutase, catalase and peroxidase activities in root tip of soybean (Glysin max). Physiologia Plantarum, 83(3), 463–468. https://doi.org/10.1111/j.1399-3054.1991.tb00121.x
- Chadha, A., Florentine, S. K., Chauhan, B. S., Long, B., Jayasundera, M., & Gonzalez-Andujar, J. L. (2019). Influence of soil moisture regimes on growth, photosynthetic capacity, leaf biochemistry and reproductive capabilities of the invasive agronomic weed; Lactuca serriola. PLoS ONE, 14(6), e0218191. https://doi.org/10.1371/journal.pone.021819
- Chai, Q., Gan, Y., Zhao, C., Xu, H. L., Waskom, R. M., Niu, Y., & Siddique, K. H. M. (2016). Regulated deficit irrigation for crop production under drought stress. A review. Agronomy for Sustainable Development, 36(1), 3. https://doi.org/10.1007/s13593-015-0338-6
- Cheng, L., Han, M., Yang, L. M., Yang, L., Sun, Z., & Zhang, T. (2018). Changes in the physiological characteristics and baicalin biosynthesis metabolism of Scutellaria baicalensis Georgi under drought stress. Industrial Crops and Products, 122, 473–482. https://doi.org/10.1016/j.indcrop.2018.06.030
- Dawood, M. G., & Sadak, M. S. (2014). Physiological role of glycinebetaine in alleviating the deleterious effects of drought stress on canola plants (Brassica napus L). Middle East Journal of Agriculture Research, 3(3), 638–644. https://www.curresweb.com/mejar/mejar/2014/943-954.pdf
- Dawood, M. G., Taie, H. A. A., Nassar, M. R. A., Abdelhamid, M. T., & Schmidhalter, U. (2014). The changes induced in the physiological, biochemical and anatomical characteristics of Vicia faba by the exogenous application of proline under seawater stress. South African Journal of Botany, 93, 54–63.
- Dos Santos, J. O., de Oliveira, L. E. M., De Souza, T., Lopes, G. M., Coelho, V. T., & Gomes, M. P. (2019). Physiological mechanisms responsible for tolerance to, and recuperation from drought conditions in four different rubber clones. Industrial Crops and Products, 141, 111714. https://doi.org/10.1016/j.indcrop.2019.111714
- DuBois, M., Gilles, K., Hamilton, J., Rebers, P., & Smith, F. (1956). Colorimetric method for determination of sugars and related substances. Analytical Chemistry, 28(3), 350–356. https://doi.org/10.1021/ac60111a017
- Elgamaal, A. A., & Maswada, H. F. (2013). Response of three yellow maize hybrids to exogenous salicylic acid under two irrigation intervals. Asian Journal of Crop Science, 5(3), 264–274. https://doi.org/10.3923/ajcs.2013.264.274
- El Sabagh, A., Hossain, A., Barutcular, C., Gormus, O., Ahmad, Z., Hussain, S., Islam, M. S., Alharby, H., Bamagoos, A., Kumar, N., Akdeniz, H., Fahad, S., Meena, R. S., Abdelhamid, M., Wasaya, A., Hasanuzzaman, M., Sorour, S., & Saneoka, H. (2019). Effect of drought stress on the quality of major oilseed crops: Implications and possible mitigation strategies - A review. Applied Ecology and Environmental Research, 17(2), 4019–4043. https://doi.org/10.15666/aeer/1702_40194043
- Fahad, S., Bajwa, A. A., Nazir, U., Anjum, S. A., Farooq, A., Zohaib, A., Sadia, S., Nasim, W., Adkins, S., Saud, S., Ihsan, M. Z., Alharby, H., Wu, C., Wang, D., & Huang, J. (2017). Crop production under drought and heat stress: Plant responses and management options. Frontiers in Plant Science, 8, 1147. https://doi.org/10.3389/fpls.2017.01147
- Faurobert, M., Pelpoir, E., & Chaïb, J. (2007). Phenol extraction of proteins for proteomic studies of recalcitrant plant tissues. Plant Proteomics. Humana Press. https://doi.org/10.1385/1-59745-227-9
- Foyer, C. H., Lopez-Delgado, H., Dat, J. H., & Scott, I. M. (1997). Hydrogen peroxide and glutathione-associated mechanism of acclamatory stress tolerance and signaling. Physiologia Plantarum, 100(2), 241–254. https://doi.org/10.1111/j.1399-3054.1997.tb04780.x
- Gao, S., Wang, Y., Yu, S., Huang, Y., Liu, H., Chen, W., & He, X. (2020). Effects of drought stress on growth, physiology and secondary metabolites of Two Adonis species in Northeast China. Scientia Horticulturae, 259(259), 108795. https://doi.org/10.1016/j.scienta.2019.108795
- Ghorbanpour, M., & Varma, A. (2017). Medicinal plants and environmental challenges. Springer International Publishing. Switzerland. https://doi.org/10.1007/978-3-319-68717-9
- Giannopolitis, C. N., & Ries, S. K. (1977). Superoxide dismutases I. Occurrence in higher plants. Plant Physiology, 59(2), 309–314. https://doi.org/10.1104/pp.59.2.309
- Hussain, M., Farooq, S., Hasan, W., Ul-Allah, S., Tanveer, M., Farooq, M., & Nawaz, A. (2018). Drought stress in sunflower: Physiological effects and its management through breeding and agronomic alternatives. Agricultural Water Management, 201, 152–166. https://doi.org/10.1016/j.agwat.2018.01.028
- Ilyasoğlu, H. (2014). Characterization of Rosehip (Rosa canina L.) Seed and Seed Oil. International Journal of Food Properties, 17(7), 1591–1598. https://doi.org/10.1080/10942912.2013.777075
- Joshan, Y., Sani, B., Jabbari, H., Mozafari, H., & Moaveni, P. (2019). Effect of drought stress on oil content and fatty acids composition of some safflower genotypes. Plant, Soil and Environment, 65(No. 11), 563–567. https://doi.org/10.17221/591/2019-PSE
- Kalaichelvi, K., & Swaminathan, A. A. (2009). Alternate land use through cultivation of medicinal and aromatic plants- A review. Agricultural Reviews, 30(3), 176–183. https://arccarticles.s3.amazonaws.com/webArticle/articles/ar303003.pdf
- Kapoor, D., Bhardwaj, S., Landi, M., Sharma, A., Ramakrishnan, M., & Sharma, A. (2020). The impact of drought in plant metabolism: How to exploit tolerance mechanisms to increase crop production. Applied Sciences, 10(16), 5692. https://doi.org/10.3390/app10165692
- Khan, N., Bano, A., Babar, M. A., & Subudhi, P. K. (2019a). Metabolic and physiological changes induced by plant growth regulators and plant growth promoting rhizobacteria and their impact on drought tolerance in Cicer arietinum L. PLoS ONE, 14(3), e0213040. pone.0213040. https://doi.org/10.1371/journal
- Khan, A., Siddiqui, A., Jamal, A., & Fatima, G. (2019b). Lallemantia royleana Benth. (Balangu): A compendious review on phytochemistry, pharmacology and ethnomedicinal uses. Journal of Drug Delivery and Therapeutics, 9(5–s), 175–177. https://doi.org/10.22270/jddt.v9i5-s.3279
- Koohdar, F., Sheidai, M., & Talebi, S. M. (2019). Population genetic structure and genetic diversity study in Lallemantia royleana (Benth.) Benth: Identification of potential gene pools in Iran. Hacquetia, 18(1), 97–104. https://doi.org/10.2478/hacq-2018-0009
- Kumar, A., Karunakar, A. P., Nath, A., & Ram Meena, B. (2017). The morphological and phenological performance of different cotton genotypes under different plant density. Journal of Applied and Natural Science, 9(4), 2242–2248. https://doi.org/10.31018/jans.v9i4.1518
- Kwasniewski, M., Daszkowska-Golec, A., Janiak, A., Chwialkowska, K., Nowakowska, U., Sablok, G., & Szarejko, I. (2016). Transcriptome analysis reveals the role of the root hairs as environmental sensors to maintain plant functions under water-deficiency conditions. Journal of Experimental Botany, 67(4), 1079–1094. https://doi.org/10.1093/jxb/erv498
- Laxa, M., Liebthal, M., Telman, W., Chibani, K., & Dietz, K. J. (2019). The role of the plant antioxidant system in drought tolerance. Antioxidants (Basel), 8(4), 94. https://doi.org/10.3390/antiox8040094
- Leng, G., & Hall, J. (2019). Crop yield sensitivity of global major agricultural countries to droughts and the projected changes in the future. Science of the Total Environment, 654, 811–821. https://doi.org/10.1016/j.scitotenv.2018.10.434
- MacKinney, G. (1941). Absorption of light by chlorophyll solutions. Journal of Biological Chemistry, 140(2), 315–322. https://doi.org/10.1016/S0021-9258(18)51320-X
- Mohasseli, V., & Sadeghi, S. (2019). Exogenously applied sodium nitroprusside improves physiological attributes and essential oil yield of two drought susceptible and resistant specie of Thymus under reduced irrigation. Industrial Crops and Products, 130, 130–136. https://doi.org/10.1016/j.indcrop.2018.12.058
- Monrroy, M., García, E., Ríos, K., & García, J. R. (2017). Extraction and physicochemical characterization of mucilage from Opuntia cochenillifera (L.) Miller. Journal of Chemistry, 2017, 1–9. https://doi.org/10.1155/2017/4301901
- Osakabe, Y., Osakabe, K., Shinozaki, K., & Tran, L. S. P. (2017). Response of plants to water stress. Frontiers in Plant Science, 5, 86. https://doi.org/10.3389/fpls.2014.00086
- Park, S. H., Lee, B. R., La, V. H., Mamun, M. A., Bae, D. W., & Kim, T. H. (2021). Drought intensity-responsive salicylic acid and abscisic acid crosstalk with the sugar signaling and metabolic pathway in Brassica napus. Plants, 10(3), 610. https://doi.org/10.3390/plants10030610
- Preite, V., Stöcklin, J., Armbruster, G. F. J., & Scheepens, J. F. (2015). Adaptation of flowering phenology and fitness-related traits across environmental gradients in the widespread Campanula rotundifolia. Ecology and Evolution, 29(2), 249–267. https://doi.org/10.1007/s10682-015-9754-y
- Razavi, S. M. A., & Moghaddam, T. M. (2011). Influence of different substitution levels of Lallemantia royleana seed gum on textural characteristics of selected hydrocolloids. Electronic Journal of Environmental, Agricultural and Food Chemistry, 10, 2826–2837. http://minerva.uvigo.es/./83
- Seif Sahandi, M., Mehrafarina, A., Naghdi Badia, H., Khalighi-Sigaroodia, F., & Sharifi, M. (2019). Improving growth, phytochemical, and antioxidant characteristics of peppermint by phosphate-solubilizing bacteria along with reducing phosphorus fertilizer use. Industrial Crops and Products, 141, 111777. https://doi.org/10.1016/j.indcrop.2019.111777
- Shahriari, Z., Heidari, B., Dadkhodaie, A., & Chen, Z.-H. (2018). Dissection of genotype × environment interactions for mucilage and seed yield in Plantago species: Application of AMMI and GGE biplot analyses. PLoS ONE, 13(5), e0196095. https://doi.org/10.1371/journal.pone.0196095
- Sharma, A., Wang, J., Xu, D., Tao, S., Chong, S., Yan, D., Li, Z., Yuan, H., & Zheng, B. (2020). Melatonin regulates the functional components of photosynthesis, antioxidant system, gene expression, and metabolic pathways to induce drought resistance in grafted Carya cathayensis plants. Science of the Total Environment, 713, 136675. https://doi.org/10.1016/j.scitotenv.2020.136675
- Tan, N. A. H., & Muhamad, I. I. (2017). Optimization of omega 3 rich oil extraction from elateriospermum tapos seed by microwave assisted aqueous enzymatic extraction. Chemical Engineering Transactions, 56, 1783–1788. https://doi.org/10.3303/CET1756298
- Teixeira, A., Iannetta, P., Binnie, K., Valentine, T. A., & Toorop, P. (2020). Myxospermous seed-mucilage quantity correlates with environmental gradients indicative of water-deficit stress: Plantago species as a model. Plant and Soil, 446(1–2), 343–356. https://doi.org/10.1007/s11104-019-04335-z
- Tohidi-Moghadam, H. R., Zahedi, H., & Ghooshchi, F. (2011). Oil quality of canola cultivars in response to water stress and super absorbent polymer application. Pesquisa Agropecuaria Tropical, 41, 579–586. https://doi.org/10.5216/pat.v41i4.13366
- Toscano, S., Farieri, E., Ferrante, A., & Romano, D. (2016). Physiological and biochemical responses in two ornamental shrubs to drought stress. Frontiers in Plant Science, 7, 645. https://doi.org/10.3389/fpls.2016.00645
- Wendel, A. (1981). Glutathione peroxidase In Methods in Enzymology (Vol. 77 pp. 325–333). Academic Press. https://doi.org/10.1016/S0076-6879(81)77046-0
- Zhang, T., Hu, Y., Zhang, K., Tian, C., & Guo, J. (2018b). Arbuscular mycorrhizal fungi improve plant growth of Ricinus communis by altering photosynthetic properties and increasing pigments under drought and salt stress. Industrial Crops and Products, 117, 13–19. https://doi.org/10.1016/j.indcrop.2018.02.087
- Zhang, S.-H., Xu, X.-F., Sun, Y.-M., Zhang, J.-L., & Li, C.-Z. (2018a). Influence of drought hardening on the resistance physiology of potato seedlings under drought stress. Journal of Integrative Agriculture, 17(2), 336–347. https://doi.org/10.1016/S2095-3119(17)61758-1