ABSTRACT
This article presents a comprehensive review on the use of recycled concrete aggregate (RCA) and recycled aggregate concrete (RAC) in construction, with emphasis on structural applications and identification of challenges and opportunities of RCA/RAC materials in Southeast Asia. For the first time and as a first step towards potential standardization of RCA/RAC in Southeast Asia, the article critically examines the physical and mechanical performance of RCA and RAC in structural applications. Global aggregate demand is projected to surpass 50 billion tons by 2025, with major Asian countries accounting for 62% of consumption. At the same time, the global annual production of construction and demolition waste (C&DW) exceeds 3.57 billion tons, and Asia is responsible for 53% of this total. Recycling C&DW plays a crucial role in addressing environmental issues and promoting sustainable construction practices. Previous research indicates that RAC exhibits certain physical and mechanical deficiencies, with strengths 10% to 20% lower than natural aggregate concrete (NAC). At the structural level, RAC elements show reductions of up to 15% in axial, bonding, shear, and flexural strengths relative to NAC. Measures such as treatment of RCA, recycling process optimization, and optimized mixing techniques are recommended to enhance RAC properties. Prioritizing RCA treatment during construction and exploring novel strengthening techniques could elevate improve RAC and make it suitable for structural applications. The review also found that C&DW recycling efforts vary significantly across countries (particularly in Southeast Asia), with some countries lagging regarding recycling technologies and use of best practices. Various strategies to improve the performance of RAC elements are also proposed and discussed. The main findings and shortcomings of previous investigations are critically discussed, and further research needs are identified.
1. Introduction
Following water, concrete is the most widely used material on a global scale (Chinnu et al. Citation2021). Concrete is widely utilized in construction due to its high strength, low cost, good durability, and adaptability. These properties make it a preferred option for infrastructure construction worldwide. Unfortunately, concrete demands the use of massive amounts of raw aggregate materials, which has led to environmental problems in many nations. Consequently, the construction industry is seeking practical solutions to make concrete more sustainable in the long term.
Aggregates (both fine and coarse) make up about 70% of the total volume of a typical concrete mix used for structural purposes (Almeida and Cunha Citation2017). Most of these are raw aggregates extracted from riverbeds and banks. Consequently, the production of new concrete poses an environmental challenge as natural resources are being depleted. This is particularly true in Asia, a continent that has experienced accelerated urbanization since the early 1980s (Hunt Citation1996; Shatkin Citation2016). Urbanization has accelerated construction in the continent, which in turn has increased the demand for aggregates. shows the proportion of aggregate consumption in major regions of the world over the last years (Makul et al. Citation2021; Tam, Soomro, and Evangelista Citation2018). While annual aggregate demand is approximately 40 billion metric tons worldwide (Slattery Citation2014) with a growth of 5.2% every five years (Wang et al. Citation2021), it is evident that most of the world’s aggregate consumption (about 62%) is concentrated in Asian countries, including China (38%) and India (13%) (Tam, Soomro, and Evangelista Citation2018). Huge demands for aggregates are also expected from Southeast Asian countries, primarily because the region is still developing, and large infrastructure projects are still being built.
Over the past two decades, the increase in population and the need for housing have driven a significant revitalization of existing buildings in major urban areas of Southeast Asia. This has resulted in a continuous cycle of demolition and new construction activities (Al-Bayati, Tighe, and Achebe Citation2018) and in a stream of construction and demolition waste (C&DW) that, if recycled and treated appropriately, can be reused in construction. This could also help address the environmental issues created by more than 3.57 billion metric tons of C&DW generated around the globe (Chen et al. Citation2011), of which Asia generates 53.2% (see ). China uses approximately 200 million tons of recycled materials in construction (Xiao et al. Citation2012), mostly recovered from its 1.13 billion ton of C&DW generated annually. Likewise, India generates 520 million tons from construction and demolition annually (Akhtar and Sarmah Citation2018; Mohanta and Murmu Citation2022), and significant efforts are underway to recycle most of these materials. Despite this progress, C&DW recycling efforts vary significantly across countries (particularly in Southeast Asia) and many lag regarding recycling technologies and use of best practices. Therefore, action and more coordinated efforts are necessary to speed up the adoption of resource-efficient practices in construction.
Past studies show that 50%–80% of C&DW waste consists of mainly concrete and bricks (Ponnada and Kameswari Citation2015; Wu et al. Citation2019). As individual components, approximately 30% of C&DW is brick masonry and 25% is concrete (Akhtar and Sarmah Citation2018; Tam, Soomro, and Evangelista Citation2018). Aggregate produced by crushing and recovering concrete from C&DW is known as recycled concrete aggregate (RCA) (Hansen Citation1986). Numerous research studies have investigated the quality of RCA and its applications in construction. Notable examples include studies on: recycled concrete aggregate properties with amounts of old adhered mortars (Duan and Poon Citation2014), the current status on the use of recycled aggregates in concrete (De Brito and Silva Citation2016), a critical review and assessment of recycled aggregate as a sustainable construction material (Kisku et al. Citation2017), characteristics and mechanical properties of composite cement-based RAC (Tejas and Pasla Citation2023), Physical, deformation, and stiffness properties of recycled concrete aggregate (Gabryś, Soból, and Sas Citation2021), novel treatment methods (Wang et al. Citation2021), alternative sustainable aggregates (Mohanta and Murmu Citation2022), factors influencing the properties of concrete incorporating construction and demolition waste (Ibrahim et al. Citation2023), assessing the relaxation of RAC from free and restrained shrinkage tests (Roziere et al. Citation2023), and strength and elastic modulus of RAC (Kakizaki et al. Citation2023), among others. summarizes relevant review articles on RCA and RAC with brief descriptions on the focus of the studies. It was found that although numerous review articles exist in the literature, only one article (Makul et al. Citation2021) focused on RCA/RAC in Southeast Asia, despite the fact that the region is the third largest consumer of concrete aggregates in the world (see ).
Figure 2. Annual production of C&DWs by continent (Akhtar and Sarmah Citation2018; Tam, Soomro, and Evangelista Citation2018).
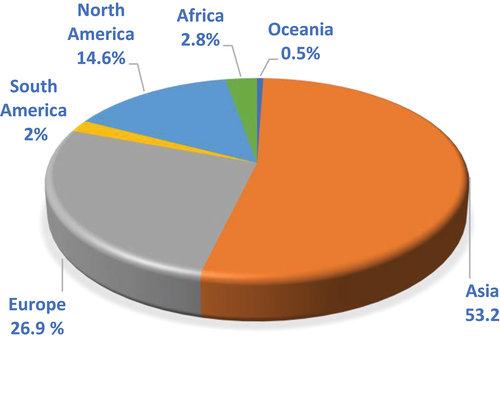
Table 1. Major reviews on RCA and RAC in recent years.
Previous research indicates that the mechanical characteristics of RAC were around 10%-20% lower than those of equivalent natural aggregate concrete (NAC) (Kazmi et al. Citation2019; Kisku et al. Citation2017; Thomas, Thaickavil, and Wilson Citation2018; Verian, Ashraf, and Cao Citation2018). The lower properties of RAC can be attributed to the poor quality of RCA, which is usually contaminated by adhered mortar. Moreover, RCA usually has micro-cracks produced by the recycling/recovery process itself. For instance, the absorption properties of RCA were found to be 10 times higher than natural aggregate (NA), whereas the bulk density of RCA was approximately 22% lower than NA (Abdulla Citation2015; Zaetang et al. Citation2016). Additionally, the compressive, splitting, and flexural properties of RAC reduced by 9.25%, 18.5%, and 17.6%, respectively, compared to equivalent NAC (Chakradhara Rao Citation2018). The performance of RAC structural members also exhibits lower (ranging from 6% to 24%) axial compression, shear resistance, and bond strength (Arezoumandi et al. Citation2015; Prince and Singh Citation2015b; Rahal and Alrefaei Citation2018). However, recent studies (Imjai et al. Citation2023a, Citation2023b; Leelatanon et al. Citation2022; Setkit et al. Citation2021) have identified significant inconsistencies in the use of RAC in structural elements, particularly when using large amounts of RCA (e.g. 100% replacement level of NA). The elimination of contaminating materials and adhered mortar is critical to improve the quality of RCA. Studies suggest that the properties of RCA can be improved by various treatments (Verian, Ashraf, and Cao Citation2018; Wang et al. Citation2021) but with different degrees of success. Simultaneously, as RAC structures demonstrated inferior bond behavior and flexure/shear strengths, the strengthening of RAC elements after construction is considered as a feasible solution that has not been explored sufficiently in the existing literature.
Whilst the construction industry in some Asian countries (e.g., Japan, India, China) have used RAC in real projects for decades, the use of RAC in Southeast Asia is just emerging and many barriers and challenges still remain for the widely adoption of RAC in construction. To bypass these challenges, the authors are working within an AMS-funded project entitled “Capacity and capability building to develop recycled aggregate concrete in Southeast Asia”, which is leveraging best practices and advancing the use of RCA and RAC across partners and stakeholders in the region.
This article presents a comprehensive review on the use of RCA and RAC in construction, with emphasis on structural applications and identification of challenges and opportunities of RCA/RAC materials in Southeast Asia. For the first time and as a first step towards potential standardization of RCA/RAC in Southeast Asia, the article examines the basic properties of RCA, including absorption values, bulk density, specific gravity, adhered mortar, abrasion, crushing, and impact values. Likewise, a thorough summary of the properties of RAC reported in the existing literature is provided, with special focus on compressive strength, tensile strength, and flexural strength. Various strategies to improve the performance of RAC elements are also proposed and discussed. The main findings and shortcomings of previous investigations are critically discussed, and further research needs are identified. This article contributes towards promoting a more efficient use of recycled materials in construction in Southeast Asian countries.
2. Sustainable sourcing of RCA in Asia
The sustainability of RCA lies in its ability to reduce landfill waste, preserve natural resources, and reduce energy consumption. RCA can reduce the environmental impact on approximately 70% of the natural concrete samples compared with recycled concrete (Knoeri, Sanyé-Mengual, and Althaus Citation2013), and also save about 10%-20% of concrete costs by the substitution of NA with RCA (Zheng et al. Citation2017). The primary source of RCA is C&DW. Globally, between 2007 and 2014, aggregate production increased from 21 billion tons to 40 billion tons (Shatkin Citation2016). Currently, the global annual production of C&DW exceeds 3.57 billion tons, with over 53.2% of it originating from Asian countries (Akhtar and Sarmah Citation2018; Tam, Soomro, and Evangelista Citation2018). Pressure exists to use this stream of recycled construction materials due to concerns with landfilling of C&DWs, as well as due to the increasing depletion of natural resources. In recent times, there has been a noticeable shift towards using RCA instead of conventional roadbed gravel and backfill materials in RAC construction (Behera et al. Citation2014). Nonetheless, hindrances exist due to the weak regulations and lack of standardization for the use of RCA and RAC in construction.
2.1. Policy and regulatory framework in Asia
Governments, organizations and standardization committees should play a vital role in advancing and applying RAC technology by establishing comprehensive RAC specifications and standards. However, owing to weak regulatory frameworks and a lack of understanding, recycled and reprocessed recycled materials are not yet considered or utilized in many design codes, particularly in Southeast Asia. In the near future, it is envisaged that the use of RAC will increase and constitute a significant portion of the market and therefore changes in policies and regulatory frameworks are urgently required.
The Japanese Construction Industry Association issued a national standard (BCSJ) for the incorporation of RCA and RAC in 1977 (Takahashi and Abe Citation1995). However, only a limited number of Asian countries (see ) have established their own distinct standards and codes for the specifications and utilization of RCA in construction projects. For example, India permits the mixing of up to 50% RCA with NA, whereas China allows up to 100% (Jagan et al. Citation2020). European countries have also developed and implemented codes, standards, and regulations for RCA/RAC (Xiao et al. Citation2022). For instance, presented by Xia et al (Xiao et al. Citation2022) in his research article such as RILEM TC121-DRG (1994) in the European Union, DIN4226–100 (2002) in Germany, DS2426 (2011) in Denmark, Digest 433 (1998) in the UK, BS 8500–2 (2002) in the UK, EHE-08 (2008) in Spain, Ot 70,085 (2006) in Switzerland, PTV 406 (2003) in Belgium, and CUR (1984) in Netherlands are prime examples of successful steps towards standardization. Brazil with its NBR 15.116 (2005), has also made significant progress.
Table 2. Acceptance criteria of RCA for RAC use in civil engineering works (Hou, Ji, and Su Citation2019; Wardeh, Ghorbel, and Gomart Citation2015; Xiao et al. Citation2022; Yang et al. Citation2020).
As the properties of RCA differ according to location, countrywide standards and guidelines should be developed to utilize RCA for different types of construction works on the basis of local prevailing recycling and construction practices. This can ensure the quality and performance of RAC, making it an environmentally friendly option for construction projects. The standards for RAC acceptance criteria in different Asian countries are presented in .
2.2. C&DW scenarios
C&DW encompasses a wide range of materials such as concrete, bricks, wood, metal, plastics, and glass. The generation of C&DW have become a significant global environmental concern owing to their sheer volume and impact on landfills, resource depletion, and overall sustainability. The volume of C&DW generated is substantial and it varies significantly from one country to another. Highly developed countries with extensive construction activities tend to produce larger quantities of C&DW. Recycling and proper waste management are crucial for mitigating the above negative impacts.
and present data on C&DW generation in various countries and continents. Asian countries, led by China and India, generate a massive amount of C&DW. With China producing 1130 million tons and India generating 530 million tons, the continent contributes significantly to the global waste burden. This may be attributed to rapid urbanization, infrastructure development, and construction projects. Recycled concrete generates approximately 585 million tons per year in major Asian countries. Every year, Asian countries generate over 53% of the total C&DW worldwide. Additionally, European countries, notably France, Germany, and the UK, also play a significant role in C&DW generation, contributing more than 26.87% collectively. This highlights the substantial construction activities in Europe. Similarly, North America generates approximately 14.59% of the global C&DW, whereas Africa and South America account for approximately 2.8% and 1.96%, respectively.
Table 3. Generation of C&DW globally (Akhtar and Sarmah Citation2018; Tam, Soomro, and Evangelista Citation2018).
C&DW waste can be divided mainly into five key categories: metal, concrete and minerals, wood, miscellaneous, and uncategorized waste. The latter consists of a combination of all other categories: concrete, bricks, ceramics, wood, glass, plastics, bituminous and asphalt, metals, stones, insulating materials, gypsum-type materials, and electronic and electrical parts. A summary of the different constituents of C&DW is presented in
According to Monier et al. (Citation2017), the main constituents of C&DW are brick (37%) masonry and concrete (31%). However, regional variations in these figures are to be expected as materials and construction practices vary from country to country.
Table 4. Constituents of C&DW (Monier et al. Citation2017).
provides valuable insights into the constituents of C&DW. Concrete and masonry are the most prevalent components in C&DW, collectively accounting for 40% to 84% of the total. Concrete, with a range of 12% to 40%, is a major contributor, reflecting its widespread use in construction projects. Masonry, with a range of 8% to 54%, includes materials like bricks and stones and adds to the bulk of waste generated. Asphalt is also significant, ranging from 4% to 26%. Minor components like wood, metal, gypsum, and plastics contribute less, with ranges between 0.1% to 4%. The average composition of C&DW constituents is depicted in . On the other hand, Jagan et al. (Jagan et al. Citation2020) conducted a study to categorize the constituents of C&DW, revealing that soils and gravels accounted for 36% of the waste, followed by brick and masonry at 31%. Concrete constituted 23% of the waste, while metals and bitumen’s contributed 5% and 2%, respectively. The remaining 3% was attributed to other miscellaneous components.
Figure 3. Major constituents in (a) C&DW, and (b) demolished concrete (Monier et al. Citation2017; Noguchi, Park, and Kitagaki Citation2015).
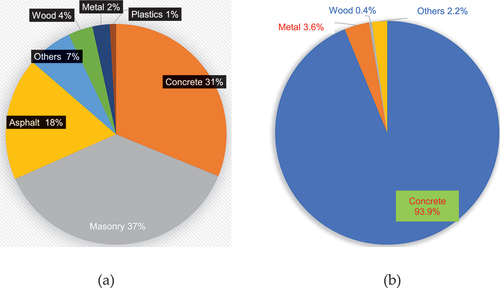
Understanding the composition of C&DW is crucial for an effective screening method and for encouraging the utilization of recycled concrete. Overall, this section underscored the diverse composition of C&DW and the significance of sustainable practices in the construction and demolition sectors, with waste reduction, recycling, and responsible management pivotal for a more environmentally friendly and resource-efficient future.
2.3. Recycling and recovery of C&DW in Asia
Recycling C&DW, with a specific focus on concrete, can significantly contribute to the sustainability of RCA. Prioritizing concrete recycling initiatives would have a considerable impact not only on waste reduction but also on resource conservation.
The recycling process and net concrete and aggregate content in concrete structures after demolition are illustrated in , based on results from Japanese concrete (Noguchi, Park, and Kitagaki Citation2015). lists the component ratios of the mixed concrete waste under different demolition scenarios. The data indicated varying proportions of concrete, metal, wood, and other materials in the waste. In Scenarios 1 and 2, concrete dominated the waste with percentages of 98% and 97.7%, respectively. However, in scenario 3, the proportion of concrete decreased to 92.8% and there was a noticeable increase in the ratios of metals and other materials. Scenarios 4 and scenario 5 continued to show a decrease in concrete contents (90% and 90.9%, respectively) and a corresponding increase in metal and other materials. Similary, delineates the different processes and methods employed for concrete crushing.
Table 5. Component ratio of mixed concrete waste (%) after demolition (Noguchi, Park, and Kitagaki Citation2015).
Table 6. Recycled aggregate production per unit weight of waste concrete.
Concrete remains a significant component of mixed concrete waste even after demolition. The data from different demolition scenarios show that concrete accounts for a high percentage, ranging from 90% to 98%. This information is vital for devising efficient utilization strategies, particularly in terms of recycling and resource recovery, and for making the most of the available resources for RCA. illustrates the typical composite constituents found in waste concrete.
The RA production techniques are categorized into three main groups: heating and rubbing, eccentric-shaft rotors, and mechanical grinding (Noguchi, Park, and Kitagaki Citation2015). The techniques are shown in .
Figure 4. Heating and rubbing technology (a) mechanical grinding technology (b) and electrical shaft rotor technology(c) (Koji Citation2010).
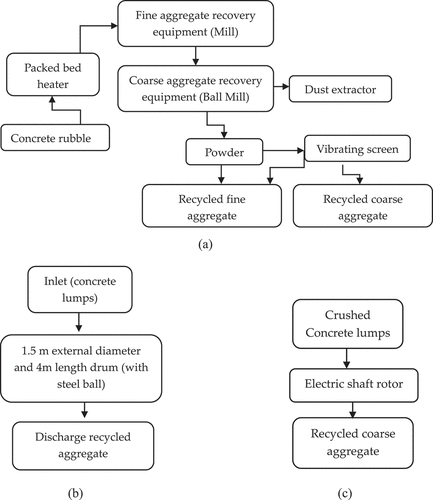
The information reported in this section confirms the importance of characterizing C&DW, which is a pending task in countries across Southeast Asia to realize the potential of RCA and RAC in construction. The authors are currently working with the recycling industry, concrete producers and other relevant stakeholders in several countries to characterize C&DW.
3. Physical and mechanical characteristics of RCA and RAC
According to ACI Committee (ACI Committee 318 Citation2008), the original concrete, contaminants, and processing/recovering technique all affect RCA quality. Recycling old concrete involves an examination of the source concrete, preparation, breaking and screening, removal of impurities (i.e., steel mesh, rebars, dowels), crushing and sizing of the RCA, and sieving (removal of impurities such as finer dust particles) (Effenberger, Kiefer, and Eni Citation1967).
RCA has various physical and mechanical characteristics that require evaluation before incorporation into concrete. Water absorption, bulk density, adhering mortar content, specific gravity, abrasion value, crushing value, and impact value of the aggregates are a few of these. The size of the coarse aggregate has an impact on how much mortar adheres to it, whilst the type of crusher used and the manufacturing process have an impact on the form and texture of aggregate. The physical, mechanical, and chemical attributes of coarse particles have a considerable impact on the strength and durability of concrete. The physical and mechanical characteristics of recycled aggregate and recycled aggregate concrete have thus been the subject of extensive research. This section discusses the key conclusions of earlier research on the characteristics of RCA and RAC.
3.1. Water absorption
A comprehensive review of laboratory test results was performed (), focusing on the absorption characteristics of NA and RCA over a 24-hour period. The analysis revealed that NA exhibited absorption values ranging from 0.05% to 2.5%, whereas RCA exhibited a much broader range of 1.56% to 7%. The date in illustrate the relative absorption values between the NA and RCA groups, highlighting the higher water absorption tendency of RCA compared to that of NA. According to earlier research, the absorption values of RCA appeared to be 1.7–10 times higher than NA. The presence of mortar can lead to higher absorption values, which is detrimental to the workability of RAC mixes and, eventually, to their compressive strength.
Table 7. Absorption percentage of NA and RCA.
A comparison of the absorption values confirms that RCA tends to absorb more water than NA, potentially affecting the concrete mix performance, workability, water demand, and long-term durability. When using RCA as a substitution for NA in concrete, its higher water absorption potential during mix design has to be considered. However, the varying quality of RCA, influenced by factors such as the source and recycling process, emphasizes the need for proper quality control and adoption of standardized recycling practices to ensure consistency and reliability. Designers and concrete technologists must be mindful of the higher water absorption of RCA and adjust the mix designs accordingly for optimal concrete performance.
3.2. Specific gravity
compares the specific gravity values of the NA and RCA from various studies. The specific gravity indicates the density of aggregates, which in turn influences the mix workability and concrete properties. The data in show that the specific gravity of NA generally falls within the range of 2.52 to 2.84, while RCA’s specific gravity varies from 2.21 to 2.66. Accordingly, the specific gravity of RCA can be 2.6% to 18.7% lower than that of NA. Understanding these relationships can aid in optimizing concrete mix designs and promoting sustainable construction practices that leverage the benefits of RCA while maintaining concrete performance. Additionally, optimizing the crushing and recycling processes to minimize the presence of lightweight particles may also contribute to higher specific gravity values of RCA.
Table 8. Specific gravity of NA and RCA.
3.3. Unit weight (bulk density)
summarizes the bulk density values of NA and RCA from various studies. It is evident that NA generally exhibits higher bulk density values than RCA. The minimum RCA value is 1270 kg/m3, corresponding to 1435 kg/m3 of NA. The range of the unit weight of RCA was 1270 kg/m3 to 1487 kg/m3, whereas the corresponding values of NA were between 1435 kg/m3 and 1832 kg/m3. The higher bulk density of NA can be attributed to its natural origin and more uniform particle distribution. On the other hand, RCA, being a recycled material, may contain variations in the size and density of particles, leading to relatively lower bulk density values.
Table 9. Unit weight (bulk density) in kg/m3 of NA and RCA.
Overall, the unit weight of RCA was 6.5% to 22.0% lower than that corresponding to NA. Therefore, to improve the quality of RCA, its density should be enhanced. To achieve this, careful sorting and processing of the recycled material can be performed to remove any lightweight or undesirable particles. Additionally, optimizing the crushing and grading process to achieve a more uniform particle distribution in the RCA can contribute to an increased bulk density. Furthermore, considering the appropriate mix design and binder materials when using RCA can enhance the overall density of the concrete mixture.
3.4. Adhered mortar contents
compares the adhered (parent) mortar in RCA determined in various studies. The results indicate that NA generally shows no adhered mortar, whereas RCA exhibits percentages varying from a low 5.0% (Zhou and Chen Citation2017) and up to 50.67% (Rahal Citation2007b). Besides the high variability of results, it is clear that much less research has focused in calculating the amount of adhered mortar, possibly due to the difficulty of the testing procedures. In RCA, excessive adhering mortar may increase water requirements, reduce workability, and alter the mix proportions. This can result in decreased strength, compromised durability, and potential segregation issues, ultimately affecting the overall quality of concrete. Additionally, segregation issues can arise, further impacting the concrete quality. To address these challenges, effective methods for minimizing the adhered mortar during recycling and processing should be explored. Implementing advanced crushing and screening technologies, along with quality control measures, can produce cleaner and higher-quality RCA with a reduced mortar content. Moreover, the relatively low production costs in Southeast Asian countries may offer cost advantages in obtaining RCA with a lower adhered mortar content, thus making it cost-effective in the region.
Table 10. Adhered mortar in RCA.
3.5. Abrasion, crushing and impact values
The comparative results of abrasion, crushing and impact values of RCA from various studies are presented in . The investigation showed that the abrasion values of RCA ranged from 20.7% to 41%, whereas the corresponding abrasion values of NA were between 11.9% and 27.5%. The crushing values of RCA ranged from 25.87% to 36%, whereas NA exhibited values between 18% and 26.7%. The findings indicate that RCA’s abrasion value is 1.5 to 1.7 times higher than NAs, while its crushing values are 1.1 to 1.4 times higher, and the impact values are 1.4 to 1.5 times more significant than those of NA.
Table 11. Abrasion, crushing and impact values of NA and RCA.
The higher abrasion, crushing, and impact values for RCA highlight its reduced resistance to wear, crushing, and impact forces, which can affect the concrete’s overall durability and performance when RCA is used as a substitute for NA. The inferior mechanical properties of RCA are attributed to factors such as the presence of adhered mortar, variations in the composition and strength of the original concrete, and the recycling process. These findings emphasize the importance of carefully selecting and processing RCA to minimize its negative impact on concrete performance.
To improve the mechanical properties of RCA, it is essential to implement improved recycling and processing methods. Efficient methods for removing attached parents’ mortar and controlling the grading of RCA can yield cleaner and higher-quality aggregates. Additionally, using high-strength original concrete can enhance the mechanical properties of RCA. The characterization of RCA using standard tests is necessary for a successful mix design and therefore articles and reports in the area should always report the physical and mechanical properties of RCA used in the mix design.
3.6. Physical properties of RCA from Southern Thailand
Tests were performed to obtain the basic properties of RCA from Southern Thailand, including absorption, specific gravity, unit weight, and abrasion values. Both NA and RCA were tested for a comparative evaluation of coarse aggregate properties in the southern part of Thailand. The NA consisted of crushed natural aggregate obtained from a local quarry, while the RCA was obtained from concrete cylinders crushed with an ad hoc machine (see ). The crushed material was sieved to obtain aggregates that passed through a 20 mm sieve and was retained on a 4.75 mm sieve. Although there is no information confirming the origin of NA and RCA, it is presumed that the quarry is located in the southern region of Thailand. All experiments were conducted in accordance with the relevant ASTM Standards, including water absorption and specific gravity (CitationASTM C127), bulk density (CitationASTM P. C29/C29M-17a), and abrasion tests (ASTM C131). The test results are presented in .
Table 12. Physical and mechanical properties of NA and RCA obtained from tests.
The experimental results demonstrate that the absorption value of the RCA was more than 15 times higher than that of the NA, whereas the RCA’s relative density (specific gravity) is approximately 18% lower than NA’s. Likewise, the bulk density of RCA is about 17% lower than that of NA. The abrasion value of RCA was approximately 37%, whereas the corresponding value of NA was approximately 25%.
3.7. Discussion on RCA properties and its influences in RAC quality
The findings from various studies emphasize the critical role that the physical and mechanical properties of aggregates play in determining the strength and durability of RAC. RCAs are notably deficient compared to NA, particularly in terms of water absorption (), specific gravity (), bulk density (), adhered mortar, abrasion values (), crushing value, and impact value. These results are confirmed by the experimental results obtained from the NA and RCA tested in this study (see ). The trends in the above figures also suggest that while some RCA properties such as specific gravity and unit weight remain within certain limits, other such as absorption and abrasion show a significant variability. RCA exhibit variations in physical properties based on location, recycling methods, and original material quality. The variations in these properties are attributed to factors such as location, recycling methods, and quality of the original materials.
Figure 8. Properties of NA and RCA from Southern Thailand (a) absorption, (b) unit weight, (c) specific gravity, and (d) abrasion value.
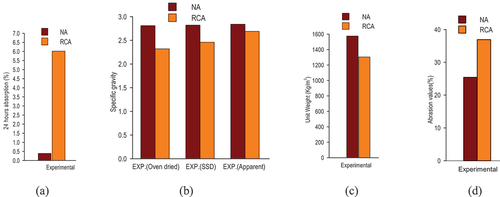
To enhance the performance of concrete with RCA, it is essential to address the deficiencies in its physical properties. Numerous studies (González-Taboada et al. Citation2016; Mohanta and Murmu Citation2022; Verian, Ashraf, and Cao Citation2018; Wang et al. Citation2021) have proposed treatment techniques and methods to enhance the properties of RCA and RAC before and during concrete mixing. The techniques and approaches are summarized below.
3.7.1. Before mixing
Reducing RCA porosity and minimizing adhered mortar layers (can be reduced 12% to 20% of initial mass of RA) can improve the overall quality (e.g. density, absorption, abrasion, bulk density) of RAC.
Coating the RCA surface with pozzolanic powder has demonstrated potential for enhancing the mechanical and physical properties of RAC.
3.7.2. During mixing
The properties of RAC can be improved through the use of various mixing techniques, including a two-stage mixing strategy, mortar mixing approach, and sand-encased mixing approach.
Incorporating supplementary cementitious materials (e.g. fly ash, granulated blast furnace slag, silica fume, or fiber reinforcement) can increase the compressive, splitting, and flexural strength of RAC.
Limiting the mortar contents in RCA and reducing the proportion of RCA in concrete mixes can help achieve better results.
While successful, it is clear that some of the above techniques and approaches will undoubtedly increase the cost of RAC and therefore they should only be used in construction if the additional costs is outweighed by an improvement in the properties of hardened RAC.
3.8. Properties of hardened RAC
compares the compressive, splitting and flexural strengths of RAC and NAC from previous investigations. The test results focused exclusively on untreated RCA sourced from demolished concrete. The chosen concrete samples only comprised RAC made with 100% RCA, allowing for a comparative analysis with the corresponding NAC from each study. All reported tests followed local codes and standards. For instance, Dimitriou et al. (Citation2018) tested the compressive strength in accordance with EN 12,390–3 (2009b), flexural strength according to EN 12,390–5 (2009), and splitting tensile strength following EN 12,390–6 (2009a). Purushothaman et al. (Citation2015) executed the tests following IS 516–1959 (IS 1959), whereas Chakradhara Rao (Citation2018) followed BSI (Citation2009b).
Table 13. Mechanical properties of NAC and RAC (100% RCA replacement).
3.8.1. Compressive strength
According to , the 28-day compressive strength of RAC was found to be between 68% to 99% of the strength of NAC. Overall, the average strength of RAC was 13.5% lower than that of NAC. The data in indicate that RAC exhibits relatively larger scatter of results (most of the corresponding strength variation of was from −0.6% to −26%), and this is likely due to the varying quality of RCA and to the presence of impurities. However, with appropriate measures, RAC is still a valuable and sustainable alternative for certain construction applications. Strategic improvements in recycled aggregate quality through better sorting and screening techniques to remove impurities and weak components, thereby improving the strength of RAC and mix design, can contribute to enhancing RAC’s overall mechanical properties of RAC and make it a more viable option in the construction industry (González-Taboada et al. Citation2016; Mohanta and Murmu Citation2022; Verian, Ashraf, and Cao Citation2018; Wang et al. Citation2021).
3.8.2. Splitting tensile strength
The results in show that the tensile strength of RAC ranged from 77.14% to 104% compared to equivalent NAC results. Overall, RAC’s splitting tensile strength demonstrated a range of approximately + 4% to −27% when compared to NAC. It is evident that the tensile strength is directly influenced by the compressive strength of the concrete, which, in turn, is governed by the quality of its constituents (cement, coarse aggregate, and fine aggregate). To enhance the tensile properties of concrete, it is recommended to improve the physical and mechanical properties of RCA through advanced crushing processes, removal of adhered mortar, proper screening of fine particles, and addition of admixtures during mixing, followed by appropriate curing methods.
3.8.3. Flexural strength
The flexural strength of RCA in was approximately 19% lower compared to equivalent NAC results, with a reduction of up to 40%. From the available data, NAC generally exhibited higher flexural strength values than RAC. To address this difference and enhance the flexural strength of RAC, the incorporation of suitable admixtures and fiber reinforcement is recommended. Implementing these solutions can narrow the gap between the NAC and RAC flexural strength values, making RAC a more competitive and sustainable option.
3.9. Mechanical properties of RCA from Southern Thailand
The mechanical properties of RAC and NAC were examined in via laboratory tests on cylinders (Cy) and cubes (Cu) using a novel custom-made concrete crusher machine (model WU-eco CRM) as shown in Appendix A. The test results are presented in . The target compressive strengths were M15, M21, and M24, and the ingredient proportioning was executed as per ACI 211.1–91 (Citation1991). Likewise, for the test of splitting strength and flexural properties, 3–3 numbers of RCA and NAC cylinders (159 mm diameter and 300 m height), and the same quantities of rectangular beams (size 100 mm × 100 mm × 500 mm) were cast considering a strength of M24. The 28 days compressive strength was determined according to BSI (Citation2009a), the tensile splitting test was carried out according to BSI (Citation2009c), and the flexural strength was determined according to BSI (Citation2009b).
Table 14. Compressive, tensile and flexural strengths of NAC and RAC from Southern Thailand.
The results in indicate that overall, the compressive strength of RAC cubes and cylinders was approximately 17% lower when compared to NA equivalents. Likewise, the splitting and flexural strengths of RAC specimens were 17% and 40% lower than that of NAC counterparts. The experimental results indicate no significant variation in the deficient strength between the RAC and NAC for the concrete grades produced using Southern Thailand’s materials.
3.10. Discussion on the properties and its influences towards the performance of RAC
The comprehensive review and test results presented in the previous section confirm the significant differences in RAC properties compared to NAC. RAC’s compressive strength () is 10–20% lower, splitting tensile strength () is 26% lower, and flexural strength is about 19% lower than NAC. The study emphasized that the quality of recycled aggregates and the presence of impurities in the RAC contributed to the observed variations. Graphical representations of previous studies and experimental results are presented in .
Figure 9. (a) compressive, and (b) splitting tensile strengths, and (c) flexural strengths from past studies of NAC and from past studies.
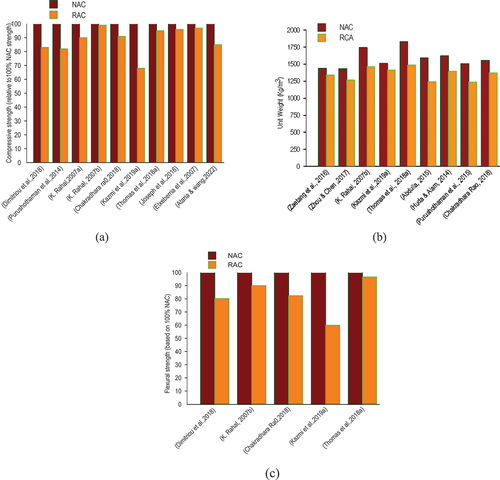
Figure 10. (a) compressive (b) tensile and (c) flexural strengths obtained from tests on Southern Thailand’s NAC and RAC.
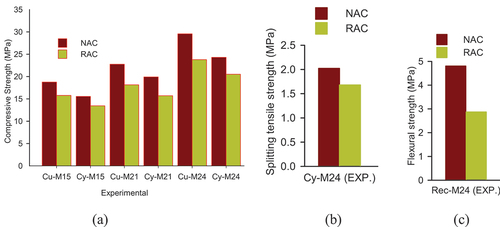
Whilst the above sections focused on the properties of NAC and RAC at the “material” level, the performance of actual structural elements cast with RAC is also discussed in the following sections so as to provide further insight into the potential uses and limitations of RAC in actual construction projects.
4. Performance of RAC structural elements
The performance of structural elements subjected to loads is influenced by the quality of their materials, including concrete and internal reinforcement. This section gives an overview of previous findings about the structural behavior of RAC members.
4.1. Bond behavior
Sufficient bond strength is essential to ensure the structural integrity and effective load transfer between concrete and reinforcing rebars in structures. The bond behavior mechanism of rebars embedded in RAC was found to be somehow similar to that of NAC reported in the literature, although the magnitude of bond strength varies. Prince & Singh (Citation2015a) performed pull-out tests and reported that the average bond strength of RAC was 2.3% higher than that of NAC. However, RAC had 33% lower compressive strength than NAC in normal strength concrete. The compressive strength of RAC for high-strength concrete was 27% lower compared to NAC, and its measured bond strength was 15% lower than NAC. Prince & Singh (Citation2015b) also conducted pullout tests using cylindrical specimens (100 mm in diameter and 200 mm in length) with concentric rebars. It was found the bond strength of RAC of 8 mm diameter rebars was 5.25% greater than that of NAC, but it was 9.75% lower with 10 mm rebars, even though the compressive strength of the NAC remained constant at 51.14 MPa and 35.58 of RAC (30% less than NAC) (refer ). Pandurangan et al. (Citation2016) studied the bond strength of RAC from untreated and treated RCA. The RILEM beam bond test (RILEM Citation1994) with 375 × 180 × 100 mm size concrete specimen was used to evaluate the bond strength of 10 mm diameter rebars. The experiment was carried out in four series: one with untreated RCA, and three with RCA treated in different ways. The bond strength of RAC without treatment of RCA was found to be 7.81 MPa, corresponding to 13.12 MPa of NAC, whereas the compressive strength was 36.96 MPa against the 42.95 MPa of NAC (see ). The bond strengths of treated RCA concrete for three samples were 7%, 13%, and 24% lower respectively, compared to equivalent values of NAC. Alhawat and Ashour (Citation2020) concluded that the bond strength of RAC (with 50% NA) dropped to 6% in the case of normal concrete strength, while compressive strength decreased by 8.5%, compared to NAC. Similarly, RAC with 100% RCA had an 11% lower bond strength, resulting in a 15% decrease in compressive strength when compared to NAC.
Figure 11. Bond strengths of NAC and RAC by (a) Prince & Singh (Citation2015b), and (b) Pandurangan et al. (Citation2016).
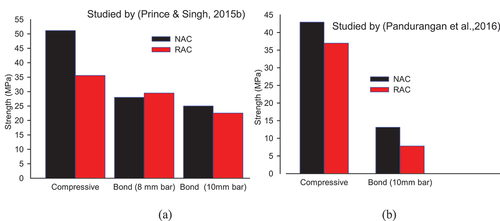
Whilst the experimental evidence to date suggests that the bond strength of bars embedded in RAC is inferior to equivalent NAC samples, the high variability and inconsistency of results indicate that additional experimental research is needed to clarify the complexity of rebar debonding where splitting, pullout and/or combined failures can occur. Moreover, existing studies have studied bond strength using short embedment lengths (5 to 10 bar diameters), which tend to over-predict bond stresses. Results from tests on standard beam-splice RAC specimens with lap splices longer than 15–20 bar diameters (e.g., Garcia et al. Citation2015b; Citation2015a, ; Helal et al. Citation2016a) would enable direct comparisons of results, provide suitable data to develop bond strength models, and aid eventual standardization. Moreover, analytical and numerical studies on the subject are also necessary.
4.2. Shear behavior
The test results by Arezoumandi et al. (Citation2015) demonstrated that 100% RCA concrete beams had, on average, 11% lower shear strength compared to beams built with 50% RCA concrete (see ). This finding was consistent with the analysis conducted using parametric and nonparametric methods, which indicated that RAC beams with 100% RCA exhibited lower shear capacity compared to NAC and 50% RCA concrete beams. Notably, shear capacity did not differ significantly between NA and 50% RCA concrete beams. Additionally, a decrease in the basic mechanical properties, such as the splitting tensile strength, flexural strength, and fracture energy, was observed. Rahal and Alrefaei (Citation2018) also investigated the shear behavior of RAC beams with reinforcements. The experimental results demonstrated that the average shear strength of RC-beams containing 20% RCA and 100% RCA decreased by only 5% and 9%, respectively, when compared to beams of NAC (refer ). Furthermore, the results revealed that the small RCA percentage had no effect on shear cracking patterns, critical shear fractures, longitudinal steel stresses, or mode of failure. The mid-span deflections of the beams were, however, significantly higher (25%) for 100% RCA concrete beams reinforced longitudinally and transversely than for beams reinforced simply longitudinally. On the other hand, Leelatanon et al. (Citation2022) explored the punching shear behavior of RCA slabs. The deflections of slabs made with 100% RCA were 15% and 18% higher that of NAC counterparts when using flexural reinforcement ratios of 1.5% and 0.8%, respectively. The test results also demonstrated that doubling the flexural rebars reduced the deflection of 100% RCA slabs by 68%. Additionally, the normalized punching shear capacity exhibited differences of 6.5% and 9% between the controlled slabs and 100% RCA slabs with flexural rebars of 1.5% and 0.8%, respectively. Sahoo and Singh (Citation2021) examined the punching shear capacity of RAC slab-column connections. The study revealed that for a given concrete compressive strength, replacing NA with 100% RCA had an insignificant effect on punching shear capacity. However, for connections with 100% RCA, there was an increase in the enveloped area (energy) by approximately 18%, 10%, and 16.6% in test specimens with concrete strengths of 28 MPa, 43 MPa, and 60 MPa, respectively. Saribas et al. (Citation2021) studied on the shear-flexure interaction in a RAC column. This study examined the impact of inelastic flexural deformation on the shear strength of columns constructed with a replacement ratio of 50% RCA. The results indicate that both NAC and RAC columns had similar seismic performances in various shear-flexure interaction scenarios. However, reducing the ratio of the transverse reinforcement can decrease the deformation capability of the columns, owing to the heightened influence of shear deformations.
Figure 12. Comparison of shear strengths of NAC and RAC by (a) Arezoumandi et al. (Citation2015), and (b) Rahal and Alrefaei (Citation2018).
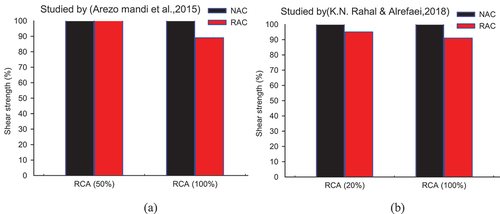
Overall, the results in the literature confirm that the shear strength of RAC elements is lower compared to NAC counterparts. However, some results are inconsistent and even contradictory, which can be attributed to the physical variations of the coarse RCA (Leelatanon et al. Citation2022; Setkit et al. Citation2021). The evidence also suggests that a threshold exists in the percentage of RCA after which the shear strength of RAC is significantly reduced, although that threshold is difficult to determine without tests. As a result, additional research is necessary to investigate how different RCA percentages affect the individual components of concrete shear strengths (e.g., aggregate interlock and dowel action) as well as shear cracking mechanisms. The latter is relevant since research has shown that the formation/development of wide shear cracks can increase the deflection of concrete elements by up to 30% (Imjai et al. Citation2016, Citation2023), which has implications in the service behavior of elements.
4.3. Flexural and shear behavior towards seismic performance
Liu, Yan, and Zou (Citation2018) examined the seismic performance of RAC columns subjected to freeze-thaw cycles (FTCs). Both RAC and NAC specimens exhibited flexural failure under constant and reverse cyclic load. Notably, specimens with RAC made with 100% RCA displayed poor frost resistance, resulting in significant loss of ductility and peak lateral load capacity during high FTCs. The study concluded that 100% RCA concrete may have deficiencies in seismic performance when exposed to freeze-thaw cycles. Similarly, Liu et al. (Citation2019) tested the seismic performance of RAC columns subjected to low-cyclic lateral loads. The failure process of RAC columns was comparable to that of NAC columns. This suggests that the hysteretic behavior, ductility, and energy dissipation of the RAC columns satisfy the seismic requirements for structural elements. Hu and Kundu (Citation2019) subjected beam-column joints constructed with RAC made with 100% RCA to quasi-static loading. The focus was on evaluating the strength, stiffness, energy dissipation, damping ratio, and column compressive performance. It was found that higher axial loads on RAC joints enhanced seismic performance. Secondly, an increased longitudinal reinforcement ratio improved the strength but decreases the ductility, energy dissipation, and viscous damping, leading to damage accumulation. Additionally, the observed shear strength of the joints was 15% higher compared to the predicted strength based on prevailing codes. Therefore, Hu and Kundu concluded that RAC joints with appropriate design can achieve high ductility. More recently, Zhang et al. (Citation2021) conducted an experimental investigation of the seismic performance of RAC shear walls under horizontal cyclic loads. The walls showed a satisfactory performance with desirable energy dissipation, stable bearing capacity, and deformation capacity. Failure in RAC walls reinforced with high-strength steel (HSS) primarily occurred because of bending. However, at a 100% replacement of RCA, the RAC wall had minimal impact on bearing capacity and energy dissipation, resulting in a slight reduction in ductility. Conversely, increasing the strength of RAC using ultra-high-strength steel (UHSS) enhanced the peak bearing capacity by 68% compared to walls with HSS reinforcement.
The experimental evidence to date suggests that the use of RAC in structural elements is feasible. However, protective measures should be implemented so that RAC structures meet long-term durability requirements. This is relevant in Southeast Asia, where the hot and humid weather quickly corrodes the internal steel reinforcement of structures. A potential solution could be the use of fiber reinforced polymer (FRP) reinforcement, but additional research is needed to develop guidelines for FRP-reinforced RAC structures. Further research is also needed to investigate the behavior of RAC structures exposed to aggressive environments (e.g., near coastal areas or wet – dry cycles).
5. Needs and approaches for improving the quality of RAC members
From a comprehensive analysis of previous studies and test results, the need to enhance the properties of RAC arises from the low engineering properties of RCA. These deficiencies hinder the overall performance and durability of RAC in structural applications, thus necessitating improvements to bridge the gap between the properties of RAC and those of NAC. Numerous studies (González-Taboada et al. Citation2016; Mohanta and Murmu Citation2022; Verian, Ashraf, and Cao Citation2018; Wang et al. Citation2021) have proposed different methods and techniques to enhance the properties of recycled aggregate concrete prior to and during mixing, as discussed in Section 3.8.
The findings confirm that most improvements on RAC properties have been proposed as pre-construction treatments, whereas post-construction solutions are scarce. However, RAC (as a relatively low-strength LS concrete) could be externally strengthened to increase its capacity. Various techniques have been proposed to improve the capacity and ductility of LS RC (reinforced concrete) columns built using NAC. These techniques include external confinement methods, such as FRP jackets (Geng et al. Citation1998; Ilki et al. Citation2008; Raffoul et al. Citation2019), post-tensioned metal strap (PTMS) confinement (Imjai et al. Citation2018). These techniques and materials have been widely used to strengthen weak RC members made with NAC because they enhance the load-carrying capacity, ductility, and structural integrity. FRP composite applications (Cao, Wu, and Jiang Citation2018; Ghobarah and El-Amoury Citation2005; Parvin et al. Citation2010; Sezen Citation2012; Zhou et al. Citation2015) have proven effective in increasing the capacity and ductility of LS RC columns. For instance, Ilki et al. (Citation2008) have shown that, compared to unconfined columns, FRP-confined LS RC columns with circular, square, and rectangular sections have higher capacities of up to 3, 1.9, and 1.4 times, respectively. Previous studies have also shown the effectiveness of PTMS confinement in improving the behavior of deficient normal-strength concrete elements (Garcia et al. Citation2017; Helal et al. Citation2016b; Ma et al. Citation2019; Moghaddam et al. Citation2010). Likewise, this technique has proven effective at enhancing the capacity and ductility of normal and high-strength concrete columns (Awang et al. Citation2012; Chau-Khun et al. Citation2015, Hoong-Pin et al., Citation2016; Ma et al. Citation2016). The effective implementation of these methods as post-construction treatments can notably enhance the axial and shear capacities of reinforced concrete members.
The review revealed that limited research has investigated the use of strengthening techniques on RAC elements. In particular, the effect of external confinement on RAC columns was investigated in only two studies that applied passive confinement (Han et al. Citation2020; Ma et al. Citation2022). Other cost-effective strengthening techniques (such as PTMS) able to apply active confinement to RAC elements have not been explored. The use of PTMS in Southeast Asia is expected to lead to more efficient and cost-effective solutions compared to other strengthening methods such as FRP jackets, and thus additional research is recommended in this area. Moreover, practical models (e.g., Huang et al. Citation2019) are also necessary for the accurate prediction of creep and fatigue performance of RAC elements. Due to the high seismic risk in some Southeast Asian countries (e.g., Indonesia, the Philippines) further research could also investigate the use of RAC components as structural control devices or energy dissipation dampers (e.g., Wang, Zhou, and Shi Citation2023; Wang et al. Citation2023; Zhang, Wang, and Shi Citation2023).
It should be noted that past research has also investigated the reuse of other C&DW as recycled aggregates (RA) in concrete, including RA such as steel slags (Chen et al. Citation2020; Gencel et al. Citation2021; Lai et al. Citation2021; Papachristoforou, Anastasiou, and Papayianni Citation2020), ceramic waste (Gonzalez-Corominas and Etxeberria Citation2014; Nepomuceno, Isidoro, and Catarino Citation2018; Ray et al. Citation2021), refectory brick aggregates (Cachim Citation2009; Hou et al. Citation2021; Islam and Shahjalal Citation2021; Zhao et al. Citation2018), glass waste (Harrison, Berenjian, and Seifan Citation2020; Pauzi et al. Citation2021) and clay aggregates (Junaid et al. Citation2022; Lotfy, Hossain, and Lachemi Citation2016; Nahhab and Ketab Citation2020). However, this sort of RA is outside the scope of this article and therefore future research should investigate the use of these alternatives in construction.
6. Conclusions and further recommendations
6.1. Conclusions
This article presents a comprehensive review on the use of recycled concrete aggregate (RCA) and recycled aggregate concrete (RAC) in construction, with emphasis on structural applications and identification of challenges and opportunities of RCA/RAC materials in Southeast Asia. For the first time and as a first step towards potential standardization of RCA/RAC in Southeast Asia, the article examines the basic properties of RCA, including absorption values, bulk density, specific gravity, adhered mortar, abrasion, crushing, and impact values. Likewise, a thorough summary of the properties of RAC reported in the existing literature is provided, with special focus on compressive strength, tensile strength, and flexural strength. Various strategies to improve the performance of RAC elements are also proposed and discussed. The main findings and shortcomings of previous investigations are critically discussed, and further research needs are identified. Based on the review and laboratory tests presented in this article, following conclusions are drawn:
Southeast Asia is the third largest consumer of aggregates in the world, with a huge potential to recycle and recover RCA from construction and demolition waste (C&DW). Nonetheless, hindrances exist due to weak regulatory frameworks and lack of standardization for the use of RCA and RAC in construction. Good practices and experience from other countries (Japan, India, China) could be adapted and adopted to encourage and extend the used of RCA/RAC in the region.
The physical and mechanical properties of RCA can differ significantly from those of natural aggregates (NA). Compared to NA, RCA has higher absorption levels, adhered mortar, abrasion, crushing, and impact values, whereas it has lower specific gravity bulk density. Better recycling/recovering methods can be used to enhance such properties with different degrees of success, Likewise, characterizing C&DW and RCA through standard tests is necessary to realize the potential of RCA and RAC in construction.
Tests at the material level shows that, compared to NAC, RAC has lower compressive, splitting tensile, and flexural strengths (ranging from 10% to 26%), depending on the level of RCA replacement. However, inconsistencies still in experimental results exist, particularly when using large amounts of RCA (e.g., 100% replacement level of NA).
RAC structural members exhibit lower axial compression, shear, and bond behaviours, with reductions ranging from 6% to 24%. However, in some cases RAC elements have similar behaviours to NAC counterparts. The experimental evidence suggests that a threshold exists in the percentage of RCA after which the shear and flexural strengths of RAC are significantly reduced, although such threshold is difficult to determine without tests.
The use of large amounts of RCA (e.g., 100% replacement level of NA) to build RAC structural elements has led to notable inconsistencies in test results. Moreover, protective measures should be implemented so that such RAC structures meet long-term durability requirements. This is relevant in Southeast Asia, where the hot and humid weather quickly corrodes the internal steel reinforcement of structures.
6.2. Further recommendations and research needs
RCA should be subjected to quality control including proper screening and crushing, was well as to removal of impurities and adhered mortar to enhance its quality. The use of admixtures can also improve the properties of RCA. Southeast Asia should take advantage of low production costs to position as producers of high volumes of standardized RCA. However, the additional costs of any treatment should be outweighed by an improvement in the final properties of hardened RAC. Future research should explore the use AI algorithms to optimize the design of RAC mixes.
Whilst the test results suggests that the bond strength of bars embedded in RAC is inferior to equivalent NAC samples, the high variability and inconsistency of results indicate that additional experimental research is needed to clarify the complexity of rebar debonding where splitting, pullout and/or combined failures can occur. Moreover, existing studies have studied bond strength using short embedment lengths (5 to 10 bar diameters), which are known to over-predict bond stresses. Results from tests on standard beam-splice RAC specimens with lap splices longer than 15-20 bar diameters would enable direct comparisons of results, provide suitable data to develop bond strength models, and aid eventual standardization. Moreover, analytical and numerical studies on the subject are also necessary.
Overall, the results in the literature confirm that the shear strength of RAC elements is lower compared to NAC counterparts. However, some results are inconsistent and even contradictory, which can be attributed to the physical variations of the coarse RCA. As a result, additional research is necessary to investigate how different RCA percentages affect the individual components of concrete shear strengths (particularly aggregate interlock and dowel action), as well as shear cracking mechanisms. The latter is relevant since the deflection of concrete elements can increased by up to 30% due to shear cracks, which has implications in the service behavior of RAC elements.
Although the use of RAC in structural elements in Southeast Asia is feasible, durability issues such as corrosion of internal steel reinforcement need to be addressed through additional tests. A potential solution to reduce corrosion could be the use of fiber reinforced polymer (FRP) reinforcement, but additional research is needed to develop guidelines for FRP-reinforced RAC structures. Further research is also needed to investigate the behavior of RAC structures exposed to aggressive environments (e.g., near coastal areas or wet – dry cycles), as well as creep and fatigue loads.
Further research should also examine the use of cost-effective strengthening techniques such as Post Tensioned Metal Straps (PTMS). PTMS can apply active confinement to RAC elements and increase their capacity and ductility. The use of PTMS in Southeast Asia is expected to lead to more efficient and cost-effective solutions compared to other strengthening methods such as FRP jackets.
Implementing the above recommendations can address some of the drawbacks related to RCA and enhance the overall performance and suitability of RAC in structural applications.
Acknowledgements
This research was supported by Thailand Science Research and Innovation Fund Contract No. FRB660041/0227. This research was financially supported by a Walailak University Graduate Research Fund. The authors acknowledge the support provided by the India Science and Research Fellowship (ISRF 2022-2023) between Thailand and India.
Disclosure statement
No potential conflict of interest was reported by the author(s).
Additional information
Funding
Notes on contributors
Ram Prasad Neupane
Ram Prasad Neupane, MSc, Assistant professor, Research fields: Composite materials, Recycled aggregate concrete, Concrete Innovation.
Thanongsak Imjai
Thanongsak Imjai, Ph.D., Associate Professor, Senior Principal Civil Engineer, Research fields: Composite materials, Recycled aggregate concrete, Concrete Innovation.
Natt Makul
Natt Makul Ph.D., Associate Professor, Research fields: Recycled aggregate concrete, Concrete material.
Reyes Garcia
Reyes Garcia, Ph.D., Assistant professor, Research fields: Composite materials, Recycled aggregate concrete, Concrete structures.
Boksun Kim
Boksun Kim, Ph.D., Associate Professor, Research fields: Concrete materials, Recycled aggregate concrete, Concrete structures.
Sandeep Chaudhary
Sandeep Chaudhary, Ph.D., Professor, Research fields: Green construction materials, Recycled aggregate concrete, Concrete structures.
References
- Abdulla, N. A. 2015. “Effect of Recycled Coarse Aggregate Type on Concrete.” Journal of Materials in Civil Engineering 27 (10): 1–9. https://doi.org/10.1061/(asce)mt.1943-5533.0001247.
- ACI Committee 318. 2008. Building Code Requirements for Structural Concrete (ACI 318-08) and Commentary. Farmington Hills, MI, USA: American Concrete Institute.
- Akhtar, A., and A. K. Sarmah. 2018. “Construction and Demolition Waste Generation and Properties of Recycled Aggregate Concrete: A Global Perspective.” Journal of Cleaner Production 186:262–281. https://doi.org/10.1016/j.jclepro.2018.03.085.
- Al-Bayati, H. K. A., S. L. Tighe, and J. Achebe. 2018. “Influence of Recycled Concrete Aggregate on Volumetric Properties of Hot Mix Asphalt.” Resources, Conservation & Recycling 130:200–214. https://doi.org/10.1016/j.resconrec.2017.11.027.
- Alhawat, M., and A. Ashour. 2020. “Bond Strength Between Corroded Steel and Recycled Aggregate Concrete Incorporating Nano Silica.” Construction and Building Materials 237:117441. https://doi.org/10.1016/j.conbuildmat.2019.117441.
- Almeida, A., and J. Cunha. 2017. “The Implementation of an Activity-Based Costing (ABC) System in a Manufacturing Company.” Procedia Manufacturing 13:932–939. https://doi.org/10.1016/j.promfg.2017.09.162.
- American Concrete Institute. 1991. Standard Practice for Selecting Proportions for Normal, Heavyweight, and Mass Concrete (ACI 211.1-91). Farmington Hills: ACI, Location.
- Arezoumandi, M., J. Drury, J. S. Volz, and K. H. Khayat. 2015. “Effect of Recycled Concrete Aggregate Replacement Level on Shear Strength of Reinforced Concrete Beams.” ACI Materials Journal 112 (4): 559–568. https://doi.org/10.14359/51687766.
- ASTM C127:2012 - Test Method for Density, Relative Density (Specific Gravity), and Absorption of Coarse Aggregate. West Conshohocken, Pennsylvania: ASTM International.
- ASTM, P. C29/C29M-17a. 2017. Standard Test Method for Bulk Density (“Unit Weight”) and Voids in Aggregate. West Conshohocken, Pennsylvania: ASTM. www.astm.org.
- Ataria, R. B., and Y. C. Wang. 2023. “Improving the Mechanical Properties of Recycled Aggregate Concrete with Graphene.” European Journal of Environmental and Civil Engineering 27 (4): 1747–1762. https://doi.org/10.1080/19648189.2022.2095034.
- Awang, A. Z., W. Omar, L. Hoong-Pin, and C. K. Ma. 2012. “Behaviour of Externally-Confined High Strength Concrete Column Under Uniaxial Compression Load.” Apsec-Iccer 2012 (October): 1–9. https://doi.org/10.1978/983/44826.
- Bahraq, A. A., J. Jose, M. Shameem, and M. Maslehuddin. 2022. “A Review on Treatment Techniques to Improve the Durability of Recycled Aggregate Concrete: Enhancement Mechanisms, Performance and Cost Analysis.” Journal of Building Engineering 55:104713. https://doi.org/10.1016/j.jobe.2022.104713.
- Bai, G., C. Zhu, C. Liu, and B. Liu. 2020. “An Evaluation of the Recycled Aggregate Characteristics and the Recycled Aggregate Concrete Mechanical Properties.” Construction and Building Materials 240:117978. https://doi.org/10.1016/j.conbuildmat.2019.117978.
- Behera, M., S. K. Bhattacharyya, A. K. Minocha, R. Deoliya, and S. Maiti. 2014. “Recycled Aggregate from C&D Waste & Its Use in Concrete – a Breakthrough Towards Sustainability in Construction Sector: A Review.” Construction and Building Materials 68:501–516. https://doi.org/10.1016/j.conbuildmat.2014.07.003.
- BSI. 2009a. EN BS 12390-3 Testing Hardened Concrete–Part 3: Compressive Strength of Test Specimens. London, UK: British Standard Institution.
- BSI. 2009b. EN BS 12390-5, Testing Hardened Concrete.5: Flexural Strength of Test Specimens. London, UK: British Standards.
- BSI. 2009c. EN BS 12390-6. Testing Hardened Concrete–Part 6: Splitting Tensile Strength of Test Specimens. London,UK: British Standards.
- Butler, L., J. S. West, and S. L. Tighe. 2013. “Effect of Recycled Concrete Coarse Aggregate from Multiple Sources on the Hardened Properties of Concrete with Equivalent Compressive Strength.” Construction and Building Materials 47:1292–1301. https://doi.org/10.1016/j.conbuildmat.2013.05.074.
- Cachim, P. B. 2009. “Mechanical Properties of Brick Aggregate Concrete.” Construction and Building Materials 23 (3): 1292–1297. https://doi.org/10.1016/j.conbuildmat.2008.07.023.
- Cao, Y., Y. F. Wu, and C. Jiang. 2018. “Stress-Strain Relationship of FRP Confined Concrete Columns Under Combined Axial Load and Bending Moment.” Composites Part B Engineering 134:207–217. https://doi.org/10.1016/j.compositesb.2017.09.063.
- Chakradhara Rao, M. 2018. “Properties of Recycled Aggregate and Recycled Aggregate Concrete: Effect of Parent Concrete.” Asian Journal of Civil Engineering 19 (1): 103–110. https://doi.org/10.1007/s42107-018-0011-x.
- Chau-Khun, M., A. Z. Awang, W. Omar, K. Pilakoutas, M. M. Tahir, and R. Garcia. 2015. “Elastic Design of Slender High-Strength RC Circular Columns Confined with External Tensioned Steel Straps.” Advances in Structural Engineering 18 (9): 1487–1499. https://doi.org/10.1260/1369-4332.18.9.1487.
- Chen, Z., Z. Gong, Y. Jiao, Y. Wang, K. Shi, and J. Wu. 2020. “Moisture Stability Improvement of Asphalt Mixture Considering the Surface Characteristics of Steel Slag Coarse Aggregate.” Construction and Building Materials 251:118987. https://doi.org/10.1016/j.conbuildmat.2020.118987.
- Chen, M. Z., J. T. Lin, S. P. Wu, and C. H. Liu. 2011. “Utilization of Recycled Brick Powder as Alternative Filler in Asphalt Mixture.” Construction and Building Materials 25 (4): 1532–1536. https://doi.org/10.1016/j.conbuildmat.2010.08.005.
- China Building Industry Press. 2010. Recycled Coarse Aggregate for Concrete (GB/T 25177-2010). China: National Standards of People's Republic of China.
- Chinnu, S. N., S. N. Minnu, A. Bahurudeen, and R. Senthilkumar. 2021. “Recycling of Industrial and Agricultural Wastes as Alternative Coarse Aggregates: A Step Towards Cleaner Production of Concrete.” Construction and Building Materials 287:123056. https://doi.org/10.1016/j.conbuildmat.2021.123056.
- de Andrade Salgado, F., and F. de Andrade Silva. 2022. “Recycled Aggregates from Construction and Demolition Waste Towards an Application on Structural Concrete: A Review.” Journal of Building Engineering 52 (December 2021): 1–20. https://doi.org/10.1016/j.jobe.2022.104452.
- De Brito, J., and R. Silva. 2016. “Current Status on the Use of Recycled Aggregates in Concrete: Where Do We Go from Here?” RILEM Technical Letters 1:1. https://doi.org/10.21809/rilemtechlett.2016.3.
- Deresa, S. T., J. Xu, C. Demartino, Y. A. Heo, Z. Li, and Y. Xiao. 2020. “A Review of Experimental Results on Structural Performance of Reinforced Recycled Aggregate Concrete Beams and Columns.” Advances in Structural Engineering 23 (15): 3351–3369. https://doi.org/10.1177/1369433220934564.
- Dimitriou, G., P. Savva, and M. F. Petrou. 2018. “Enhancing Mechanical and Durability Properties of Recycled Aggregate Concrete.” Construction and Building Materials 158:228–235. https://doi.org/10.1016/j.conbuildmat.2017.09.137.
- Duan, Z. H., and C. S. Poon. 2014. “Properties of Recycled Aggregate Concrete Made with Recycled Aggregates with Different Amounts of Old Adhered Mortars.” Materials & Design 58:19–29. https://doi.org/10.1016/j.matdes.2014.01.044.
- Effenberger, F., G. Kiefer, and Eni. 1967. “Stereochemistry of the Cycloaddition of Sulfonyl Isocyanates AndN-Sulfinylsulfonamides to Enol Ethers.” Angewandte Chemie International Edition in English 6 (11): 951–952. https://doi.org/10.1002/anie.196709511.
- Etxeberria, M., E. Vázquez, A. Marí, and M. Barra. 2007. “Influence of the Amount of Recycled Coarse Aggregates and the Production Process on the Properties of Recycled Aggregate Concrete.” Cement and Concrete Research 37 (5): 735–742. https://doi.org/10.1016/j.cemconres.2007.02.002.
- Gabryś, K., E. Soból, and W. Sas. 2021. “Physical, Deformation, and Stiffness Properties of Recycled Concrete Aggregate.” Sustainability 13 (8): 4245. https://doi.org/10.3390/su13084245.
- Garcia, R., Y. Helal, K. Pilakoutas, and M. Guadagnini. 2015a. “Bond Strength of Short Lap Splices in RC Beams Confined with Steel Stirrups or External CFRP.” Materials and Structures 48 (1–2): 277–293. https://doi.org/10.1617/s11527-013-0183-5.
- Garcia, R., Y. Helal, K. Pilakoutas, and M. Guadagnini. 2015b. “Bond Strength of Short Lap Splices in RC Beams Confined with Steel Stirrups or External CFRP.” Materials and Structures/Materiaux Et Constructions 48 (1–2): 277–293. https://doi.org/10.1617/s11527-013-0183-5.
- Garcia, R., K. Pilakoutas, I. Hajirasouliha, M. Guadagnini, N. Kyriakides, and M. A. Ciupala. 2017. “Seismic Retrofitting of RC Buildings Using CFRP and Post-Tensioned Metal Straps: Shake Table Tests.” Bulletin of Earthquake Engineering 15 (8): 3321–3347. https://doi.org/10.1007/s10518-015-9800-8.
- Gencel, O., O. Karadag, O. H. Oren, and T. Bilir. 2021. “Steel Slag and Its Applications in Cement and Concrete Technology: A Review.” Construction and Building Materials 283:122783. https://doi.org/10.1016/j.conbuildmat.2021.122783.
- Geng, Z. J., M. J. Chajes, T. W. Chou, and D. Y. C. Pan. 1998. “The Retrofitting of Reinforced Concrete Column-To-Beam Connections.” Composites Science and Technology 58 (8): 1297–1305. https://doi.org/10.1016/S0266-3538(98)00014-1.
- Ghobarah, A., and T. El-Amoury. 2005. “Seismic Rehabilitation of Deficient Exterior Concrete Frame Joints.” Journal of Composites for Construction 9 (5): 408–416. https://doi.org/10.1061/(ASCE)1090-0268(2005)9:5(408).
- Gonzalez-Corominas, A., and M. Etxeberria. 2014. “Properties of High-Performance Concrete Made with Recycled Fine Ceramic and Coarse Mixed Aggregates.” Construction and Building Materials 68:618–626. https://doi.org/10.1016/j.conbuildmat.2014.07.016.
- González-Taboada, I., B. González-Fonteboa, F. Martínez-Abella, and D. Carro-López. 2016. “Study of Recycled Concrete Aggregate Quality and Its Relationship with Recycled Concrete Compressive Strength Using Database Analysis.” Materiales de Construcción 66 (323): e089. https://doi.org/10.3989/mc.2016.06415.
- Guo, H., C. Shi, X. Guan, J. Zhu, Y. Ding, T. C. Ling, H. Zhang, and Y. Wang. 2018. “Durability of Recycled Aggregate Concrete – a Review.” Cement and Concrete Composites 89:251–259. https://doi.org/10.1016/j.cemconcomp.2018.03.008.
- Hansen, T. C. 1986. “Recycled Aggregates and Recycled Aggregate Concrete: Second State-Of-The-Art Report Developments 1945-1985.” Materials and Structures 19 (3): 201–246. https://doi.org/10.1007/BF02472036.
- Han, Q., W. Y. Yuan, T. Ozbakkaloglu, Y. L. Bai, and X. L. Du. 2020. “Compressive Behavior for Recycled Aggregate Concrete Confined with Recycled Polyethylene Naphthalate/Terephthalate Composites.” Construction and Building Materials 261:261. https://doi.org/10.1016/j.conbuildmat.2020.120498.
- Harrison, E., A. Berenjian, and M. Seifan. 2020. “Recycling of Waste Glass as Aggregate in Cement-Based Materials.” Environmental Science and Ecotechnology 4:100064. https://doi.org/10.1016/j.ese.2020.100064.
- Helal, Y., R. Garcia, K. Pilakoutas, M. Guadagnini, and I. Hajirasouliha. 2016a. “Bond of Substandard Laps in Reinforced Concrete Beams Retrofitted with Post-Tensioned Metal Straps.” ACI Structural Journal 113 (6). https://doi.org/10.14359/51689021.
- Helal, Y., R. Garcia, K. Pilakoutas, M. Guadagnini, and I. Hajirasouliha. 2016b. “Bond of Substandard Laps in Reinforced Concrete Beams Retrofitted with Post-Tensioned Metal Straps.” ACI Structural Journal 113 (6). https://doi.org/10.14359/51689021.
- Hou, S., Z. Duan, J. Xiao, L. Li, and Y. Bai. 2021. “Effect of Moisture Condition and Brick Content in Recycled Coarse Aggregate on Rheological Properties of Fresh Concrete.” Journal of Building Engineering 35:102075. https://doi.org/10.1016/j.jobe.2020.102075.
- Hou, Y., X. Ji, and X. Su. 2019. “Mechanical Properties and Strength Criteria of Cement-Stabilized Recycled Concrete Aggregate.” International Journal of Pavement Engineering 20 (3): 339–348. https://doi.org/10.1080/10298436.2017.1293266.
- Huang, H., R. Garcia, S. S. Huang, M. Guadagnini, and K. Pilakoutas. 2019. “A Practical Creep Model for Concrete Elements Under Eccentric Compression.” Materials and Structures 52 (6): 119. https://doi.org/10.1617/s11527-019-1432-z.
- Huda, S. B., and M. S. Alam. 2014. “Mechanical Behavior of Three Generations of 100% Repeated Recycled Coarse Aggregate Concrete.” Construction and Building Materials 65:574–582. https://doi.org/10.1016/j.conbuildmat.2014.05.010.
- Hu, B., and T. Kundu. 2019. “Seismic Performance of Interior and Exterior Beam–Column Joints in Recycled Aggregate Concrete Frames.” Journal of Structural Engineering 145 (3): 1–16. https://doi.org/10.1061/(asce)st.1943-541x.0002261.
- Hunt, J. 1996. “List of Contents.” In Garden and Grove: The Italian Renaissance Garden in the English Imagination, 1600-1750, pp. v–vi. Philadelphia: University of Pennsylvania Press. https://doi.org/10.9783/9780812292787-toc.
- Ibrahim, M., W. Alimi, R. Assaggaf, B. A. Salami, and E. A. Oladapo. 2023. “An Overview of Factors Influencing the Properties of Concrete Incorporating Construction and Demolition Wastes.” Construction and Building Materials 367 (January): 130307. https://doi.org/10.1016/j.conbuildmat.2023.130307.
- Ilki, A., O. Peker, E. Karamuk, C. Demir, and N. Kumbasar. 2008. “FRP Retrofit of Low and Medium Strength Circular and Rectangular Reinforced Concrete Columns.” Journal of Materials in Civil Engineering 20 (2): 169–188. https://doi.org/10.1061/(ASCE)0899-1561(2008)20:2(169).
- Imjai, T., U. Chaisakulkiet, R. Garcia, and K. Pilakoutas. 2018. “Strengthening of RC Members Using Post-Tensioned Metal Straps: State of the Research.” Lowland Technology International 20 (2, Sep): 109–118. https://cot.unhas.ac.id/journals/index.php/ialt_lti/article/view/203.
- Imjai, T., R. Garcia, B. Kim, C. Hansapinyo, and P. Sukontasukkul. 2023a. “Serviceability Behaviour of FRP-Reinforced Slatted Slabs Made of High-Content Recycled Aggregate Concrete.” Structures 51:1071–1082. https://doi.org/10.1016/j.istruc.2023.03.075.
- Imjai, T., R. Garcia, B. Kim, C. Hansapinyo, and P. Sukontasukkul. 2023b. “Serviceability Behaviour of FRP-Reinforced Slatted Slabs Made of High-Content Recycled Aggregate Concrete.” Structures 51:1071–1082. https://doi.org/10.1016/j.istruc.2023.03.075.
- Imjai, T., M. Guadagnini, R. Garcia, and K. Pilakoutas. 2016. “A Practical Method for Determining Shear Crack Induced Deformation in FRP RC Beams.” Engineering Structures 126:353–364. https://doi.org/10.1016/j.engstruct.2016.08.007.
- Imjai, T., F. Kefyalew, P. Aosai, R. Garcia, B. Kim, H. M. Abdalla, and S. N. Raman. 2023. “A New Equation to Predict the Shear Strength of Recycled Aggregate Concrete Z Push-Off Specimens.” Cement and Concrete Research 169:107181. https://doi.org/10.1016/j.cemconres.2023.107181.
- Islam, M. J., and M. Shahjalal. 2021. “Effect of Polypropylene Plastic on Concrete Properties as a Partial Replacement of Stone and Brick Aggregate.” Case Studies in Construction Materials 15:e00627. https://doi.org/10.1016/J.CSCM.2021.E00627.
- Jagan, S., T. R. Neelakantan, L. Reddy, and R. G. Kannan. 2020. “Characterization Study on Recycled Coarse Aggregate for Its Utilization in Concrete - a Review.” Journal of Physics Conference Series 1706 (1): 012120. https://doi.org/10.1088/1742-6596/1706/1/012120.
- JIS A 5021. 2011. “Recycled Aggregate for Concrete–Class H.” In: Japanese Standards Association. Tokyo, Japan: Japanese Standards Association (JSA).
- JIS A 5022. 2012a. “Recycled Aggregate for Concrete–Class L.” In: Japanese Standards Association. Tokyo, Japan: Japanese Standards Association (JSA).
- JIS A 5022. 2012b. “Recycled Aggregate for Concrete–Class M.” In: Japanese Standards Association. Tokyo, Japan: Japanese Standards Association (JSA).
- Joseph, H. S., T. Pachiappan, S. Avudaiappan, and E. I. S. Flores. 2022. “A Study on Mechanical and Microstructural Characteristics of Concrete Using Recycled Aggregate.” Materials 15 (21): 7535. https://doi.org/10.3390/ma15217535.
- Junaid, M. F., Z. U. Rehman, M. Kuruc, I. Medveď, D. Bačinskas, J. Čurpek, M. Čekon, N. Ijaz, and W. S. Ansari. 2022. “Lightweight Concrete from a Perspective of Sustainable Reuse of Waste Byproducts.” Construction and Building Materials 319:126061. https://doi.org/10.1016/j.conbuildmat.2021.126061.
- Kakizaki, M., M. Harada, T. Soshiroda, S. Kubota, T. Ikeda, and Y. Kasai. 2023. “Strength and Elastic Modulus of Recycled Aggregate Concrete.” Proceedings of the 2nd International RILEM Symposium on Demolition and Reuse of Concrete and Masonry, 565–574.
- Katkhuda, H., and N. Shatarat. 2017. “Improving the Mechanical Properties of Recycled Concrete Aggregate Using Chopped Basalt Fibers and Acid Treatment.” Construction and Building Materials 140:328–335. https://doi.org/10.1016/j.conbuildmat.2017.02.128.
- Kazmi, S. M. S., M. J. Munir, Y. F. Wu, I. Patnaikuni, Y. Zhou, and F. Xing. 2019. “Influence of Different Treatment Methods on the Mechanical Behavior of Recycled Aggregate Concrete: A Comparative Study.” Cement and Concrete Composites 104:103398. https://doi.org/10.1016/j.cemconcomp.2019.103398.
- Kisku, N., H. Joshi, M. Ansari, S. K. Panda, S. Nayak, and S. C. Dutta. 2017. “A Critical Review and Assessment for Usage of Recycled Aggregate as Sustainable Construction Material.” Construction and Building Materials 131:721–740. https://doi.org/10.1016/j.conbuildmat.2016.11.029.
- Knoeri, C., E. Sanyé-Mengual, and H. J. Althaus. 2013. “Comparative LCA of Recycled and Conventional Concrete for Structural Applications.” The International Journal of Life Cycle Assessment 18 (5): 909–918. https://doi.org/10.1007/s11367-012-0544-2.
- Koji, S. 2010. “The Current State and Future Prospects of Waste and Recycling in Japan.” Proceedings of the First International Conference on Sustainable Urbanization, Hong Kong, 837–845.
- Korea Standards Association. 2020. Concrete Aggregate (KSF 2527). Seoul, Korea: Korea Standards Association.
- Kothari, B. R., and S. Abhay. 2016. “Experimental Investigation of Recycle Concrete Aggregate.” International Journal for Innovative Research in Science and Technology 3:511–515. ISSN: 2349-6010.
- Lai, M. H., J. Zou, B. Yao, J. C. M. Ho, X. Zhuang, and Q. Wang. 2021. “Improving Mechanical Behavior and Microstructure of Concrete by Using BOF Steel Slag Aggregate.” Construction and Building Materials 277:122269. https://doi.org/10.1016/j.conbuildmat.2021.122269.
- Lee, H. P., A. Abdullah Zawawi, and O. Wahid. 2016. “Short-Term Durability of Steel-Strap Confined Concrete.” Jurnal Teknologi (Sciences and Engineering) 78 (7): 99–106.
- Leelatanon, S., T. Imjai, M. Setkit, R. Garcia, and B. Kim. 2022. “Punching Shear Capacity of Recycled Aggregate Concrete Slabs.” Buildings 12 (10): 1584. https://doi.org/10.3390/buildings12101584.
- Liu, C., J. Fan, G. Bai, Z. Quan, G. Fu, C. Zhu, and Z. Fan. 2019. “Cyclic Load Tests and Seismic Performance of Recycled Aggregate Concrete (RAC) Columns.” Construction and Building Materials 195:682–694. https://doi.org/10.1016/j.conbuildmat.2018.10.078.
- Liu, K., J. Yan, and C. Zou. 2018. “A Pilot Experimental Study on Seismic Behavior of Recycled Aggregate Concrete Columns After Freeze-Thaw Cycles.” Construction and Building Materials 164:497–507. https://doi.org/10.1016/j.conbuildmat.2017.12.160.
- Lotfy, A., K. M. A. Hossain, and M. Lachemi. 2016. “Durability Properties of Lightweight Self-Consolidating Concrete Developed with Three Types of Aggregates.” Construction and Building Materials 106:43–54. https://doi.org/10.1016/j.conbuildmat.2015.12.118.
- Ma, C. K., A. Z. Awang, R. Garcia, W. Omar, K. Pilakoutas, and M. Azimi. 2016. “Nominal Curvature Design of Circular HSC Columns Confined with Post-Tensioned Steel Straps.” Structures 7:25–32. https://doi.org/10.1016/j.istruc.2016.04.002.
- Ma, C. K., R. Garcia, S. C. S. Yung, A. Z. Awang, W. Omar, and K. Pilakoutas. 2019. “Strengthening of Pre-Damaged Concrete Cylinders Using Post-Tensioned Steel Straps.” Proceedings of the Institution of Civil Engineers - Structures and Buildings 172 (10): 703–711. https://doi.org/10.1680/jstbu.18.00031.
- Makul, N., R. Fediuk, H. M. M. Amran, A. M. Zeyad, A. R. G. de Azevedo, S. Klyuev, N. Vatin, and M. Karelina. 2021. “Capacity to Develop Recycled Aggregate Concrete in South East Asia.” Buildings 11 (6): 234. https://doi.org/10.3390/buildings11060234.
- Ma, H., J. Qiang, J. Xi, and Y. Zhao. 2022. “Cyclic Loading Tests and Horizontal Bearing Capacity of Recycled Concrete Filled Circular Steel Tube and Profile Steel Composite Columns.” Journal of Constructional Steel Research 199 (May): 107572. https://doi.org/10.1016/j.jcsr.2022.107572.
- Marinković, S., I. Josa, S. Braymand, and N. Tošić. 2023. “Sustainability Assessment of Recycled Aggregate Concrete Structures: A Critical View on the Current State‐Of‐Knowledge and Practice.” Structural Concrete 24 (2): 1956–1979. https://doi.org/10.1002/suco.202201245.
- Matsagar, V. 2015. “Advances in Structural Engineering: Materials, Volume Three.” Advances in Structural Engineering: Materials 3:1619–2647. https://doi.org/10.1007/978-81-322-2187-6.
- Mistri, A., S. K. Bhattacharyya, N. Dhami, A. Mukherjee, and S. V. Barai. 2020. “A Review on Different Treatment Methods for Enhancing the Properties of Recycled Aggregates for Sustainable Construction Materials.” Construction and Building Materials 233:117894. https://doi.org/10.1016/j.conbuildmat.2019.117894.
- Moghaddam, H., M. Samadi, K. Pilakoutas, and S. Mohebbi. 2010. “Axial Compressive Behavior of Concrete Actively Confined by Metal Strips; Part A: Experimental Study.” Materials and Structures/Materiaux Et Constructions 43 (10): 1369–1381. https://doi.org/10.1617/s11527-010-9588-6.
- Mohanta, N. R., and M. Murmu. 2022. “Alternative Coarse Aggregate for Sustainable and Eco-Friendly Concrete - a Review.” Journal of Building Engineering 59 (August): 105079. https://doi.org/10.1016/j.jobe.2022.105079.
- Monier, V., M. Hesstin, A. Impériale, L. Prat, G. Hobbs, and K. A. M. Ramos. 2017. “Resource Efficient Use of Mixed Wastes: Improving Management of Construction and Demolition Waste.” European Union.
- Nahhab, A. H., and A. K. Ketab. 2020. “Influence of Content and Maximum Size of Light Expanded Clay Aggregate on the Fresh, Strength, and Durability Properties of Self-Compacting Lightweight Concrete Reinforced with Micro Steel Fibers.” Construction and Building Materials 233:117922. https://doi.org/10.1016/j.conbuildmat.2019.117922.
- Nepomuceno, M. C. S., R. A. S. Isidoro, and J. P. G. Catarino. 2018. “Mechanical Performance Evaluation of Concrete Made with Recycled Ceramic Coarse Aggregates from Industrial Brick Waste.” Construction and Building Materials 165:284–294. https://doi.org/10.1016/j.conbuildmat.2018.01.052.
- Noguchi, T., W. J. Park, and R. Kitagaki. 2015. “Risk Evaluation for Recycled Aggregate According to Deleterious Impurity Content Considering Deconstruction Scenarios and Production Methods.” Resources, Conservation & Recycling 104:405–416. https://doi.org/10.1016/j.resconrec.2015.08.002.
- Padmini, A. K., K. Ramamurthy, and M. S. Mathews. 2009. “Influence of Parent Concrete on the Properties of Recycled Aggregate Concrete.” Construction and Building Materials 23 (2): 829–836. https://doi.org/10.1016/j.conbuildmat.2008.03.006.
- Pandurangan, K., A. Dayanithy, and S. Om Prakash. 2016. “Influence of Treatment Methods on the Bond Strength of Recycled Aggregate Concrete.” Construction and Building Materials 120:212–221. https://doi.org/10.1016/j.conbuildmat.2016.05.093.
- Papachristoforou, M., E. K. Anastasiou, and I. Papayianni. 2020. “Durability of Steel Fiber Reinforced Concrete with Coarse Steel Slag Aggregates Including Performance at Elevated Temperatures.” Construction and Building Materials 262:120569. https://doi.org/10.1016/j.conbuildmat.2020.120569.
- Parvin, A., S. Altay, C. Yalcin, and O. Kaya. 2010. “CFRP Rehabilitation of Concrete Frame Joints with Inadequate Shear and Anchorage Details.” Journal of Composites for Construction 14 (1): 72–82. https://doi.org/10.1061/(ASCE)CC.1943-5614.0000055.
- Pauzi, N. N. M., R. Hamid, M. Jamil, and M. F. M. Zain. 2021. “The Effect of Melted-Spherical and Crushed CRT Funnel Glass Waste as Coarse Aggregates on Concrete Performance.” Journal of Building Engineering 35:102035. https://doi.org/10.1016/j.jobe.2020.102035.
- Ponnada, M. R., and P. L. Kameswari. 2015. “Construction and Demolition Waste Management – a Review.” International Journal of Advanced Science & Technology 84:19–46. https://doi.org/10.14257/ijast.2015.84.03.
- Prince, M. J. R., and B. Singh. 2015a. “Bond Behaviour of Normal- and High-Strength Recycled Aggregate Concrete.” Structural Concrete 16 (1): 56–70. https://doi.org/10.1002/suco.201300101.
- Prince, M. J. R., and B. Singh. 2015b. “Bond Strength of Deformed Steel Bars in High-Strength Recycled Aggregate Concrete.” Materials and Structures/Materiaux et Constructions 48 (12): 3913–3928. https://doi.org/10.1617/s11527-014-0452-y.
- Purushothaman, R., R. R. Amirthavalli, and L. Karan. 2015. “Influence of Treatment Methods on the Strength and Performance Characteristics of Recycled Aggregate Concrete.” Journal of Materials in Civil Engineering 27 (5): 4014168. https://doi.org/10.1061/(ASCE)MT.1943-5533.0001128.
- Raffoul, S., D. Escolano-Margarit, R. Garcia, M. Guadagnini, and K. Pilakoutas. 2019. “Constitutive Model for Rubberized Concrete Passively Confined with FRP Laminates.” Journal of Composites for Construction 23 (6): 4019044. https://doi.org/10.1061/(ASCE)CC.1943-5614.0000972.
- Rahal, K. 2007a. “Mechanical Properties of Concrete with Recycled Coarse Aggregate.” Building & Environment 42 (1): 407–415. https://doi.org/10.1016/j.buildenv.2005.07.033.
- Rahal, K. 2007b. “Mechanical Properties of Concrete with Recycled Coarse Aggregate.” Building & Environment 42 (1): 407–415. https://doi.org/10.1016/j.buildenv.2005.07.033.
- Rahal, K. N., and Y. T. Alrefaei. 2018. “Shear Strength of Recycled Aggregate Concrete Beams Containing Stirrups.” Construction and Building Materials 191:866–876. https://doi.org/10.1016/j.conbuildmat.2018.10.023.
- Ray, S., M. Haque, S. A. Soumic, A. F. Mita, M. M. Rahman, and B. B. Tanmoy. 2021. “Use of Ceramic Wastes as Aggregates in Concrete Production: A Review.” Journal of Building Engineering 43:102567. https://doi.org/10.1016/j.jobe.2021.102567.
- RILEM, T. C. 1994. “RC 6 Bond Test for Reinforcement Steel. 2.” Pull-out test, 1983.” RILEM recommendations for the testing and use of constructions materials. 218–220.
- Roziere, E., A. Z. Bendimerad, H. Samouh, and A. Loukili. 2023. “Assessing the Relaxation of Recycled Aggregates Concrete from Free and Restrained Shrinkage Tests.” Journal of Building Engineering 64:105549. https://doi.org/10.1016/j.jobe.2022.105549.
- Sahoo, S., and B. Singh. 2021. “Punching Shear Capacity of Recycled-Aggregate Concrete Slab-Column Connections.” Journal of Building Engineering 41 (3): 102430. https://doi.org/10.1016/j.jobe.2021.102430.
- Saribas, I., C. Goksu, E. Binbir, and A. Ilki. 2021. “Shear-Flexure Interaction in RAC Columns Under Simulated Seismic Actions.” Engineering Structures 231 (May 2020): 111746. https://doi.org/10.1016/j.engstruct.2020.111746.
- Setkit, M., S. Leelatanon, T. Imjai, R. Garcia, and S. Limkatanyu. 2021. “Prediction of Shear Strength of Reinforced Recycled Aggregate Concrete Beams without Stirrups.” Buildings 11 (9): 402–418. https://doi.org/10.3390/buildings11090402.
- Sezen, H. 2012. “Repair and Strengthening of Reinforced Concrete Beam-Column Joints with Fiber-Reinforced Polymer Composites.” Journal of Composites for Construction 16 (5): 499–506. https://doi.org/10.1061/(asce)cc.1943-5614.0000290.
- Shatkin, G. 2016. “The Real Estate Turn in Policy and Planning: Land Monetization and the Political Economy of Peri-Urbanization in Asia.” Cities 53:141–149. https://doi.org/10.1016/j.cities.2015.11.015.
- Silva, R. V., J. de Brito, and R. K. Dhir. 2018. “Fresh-State Performance of Recycled Aggregate Concrete: A Review.” Construction and Building Materials 178:19–31. https://doi.org/10.1016/j.conbuildmat.2018.05.149.
- Slattery, K. 2014. “Global Developments in the Aggregate Industry.” Global Aggregates Information, Network.
- Takahashi, T., and M. Abe. 1995. “States of the Arts on the Study of Recycled Aggregate of Waste Concrete and Its Reuse for Concrete Structure.” Concrete Journal 33 (2): 20–28. https://doi.org/10.3151/coj1975.33.2_20.
- Tam, V. W. Y., M. Soomro, and A. C. J. Evangelista. 2018. “A Review of Recycled Aggregate in Concrete Applications (2000–2017).” Construction and Building Materials 172:272–292. https://doi.org/10.1016/j.conbuildmat.2018.03.240.
- Tejas, S., and D. Pasla. 2023. “Assessment of Mechanical and Durability Properties of Composite Cement-Based Recycled Aggregate Concrete.” Construction and Building Materials 387 (April 2023): 131620. https://doi.org/10.1016/j.conbuildmat.2023.131620.
- Thomas, J., N. N. Thaickavil, and P. M. Wilson. 2018. “Strength and Durability of Concrete Containing Recycled Concrete Aggregates.” Journal of Building Engineering 19:349–365. https://doi.org/10.1016/j.jobe.2018.05.007.
- Verian, K. P., W. Ashraf, and Y. Cao. 2018. “Properties of Recycled Concrete Aggregate and Their Influence in New Concrete Production.” Resources, Conservation & Recycling 133 (October 2017): 30–49. https://doi.org/10.1016/j.resconrec.2018.02.005.
- Wang, L., S. Nagarajaiah, Y. Zhou, and W. Shi. 2023. “Experimental Study on Adaptive-Passive Tuned Mass Damper with Variable Stiffness for Vertical Human-Induced Vibration Control.” Engineering Structures 280 (September 2022): 115714. https://doi.org/10.1016/j.engstruct.2023.115714.
- Wang, B., L. Yan, Q. Fu, and B. Kasal. 2021. “A Comprehensive Review on Recycled Aggregate and Recycled Aggregate Concrete.” Resources, Conservation & Recycling 171 (September 2020): 105565. https://doi.org/10.1016/j.resconrec.2021.105565.
- Wang, X., X. Yang, J. Ren, N. Han, and F. Xing. 2021. “A Novel Treatment Method for Recycled Aggregate and the Mechanical Properties of Recycled Aggregate Concrete.” Journal of Materials Research and Technology 10:1389–1401. https://doi.org/10.1016/j.jmrt.2020.12.095.
- Wang, L., Y. Zhou, and W. Shi. 2023. “Seismic Control of a Smart Structure with Semiactive Tuned Mass Damper and Adaptive Stiffness Property.” Earthquake Engineering and Resilience 2 (1): 74–93. https://doi.org/10.1002/eer2.38.
- Wardeh, G., E. Ghorbel, and H. Gomart. 2015. “Mix Design and Properties of Recycled Aggregate Concretes: Applicability of Eurocode 2.” International Journal of Concrete Structures and Materials 9 (1): 1–20. https://doi.org/10.1007/s40069-014-0087-y.
- Wu, H., J. Zuo, G. Zillante, J. Wang, and H. Yuan. 2019. “Status Quo and Future Directions of Construction and Demolition Waste Research: A Critical Review.” Journal of Cleaner Production 240:240. https://doi.org/10.1016/j.jclepro.2019.118163.
- Xiao, J., Z. Cheng, Z. Zhou, and C. Wang. 2022. “Structural Engineering Applications of Recycled Aggregate Concrete: Seismic Performance, Guidelines, Projects and Demonstrations.” Case Studies in Construction Materials 17 (September). https://doi.org/10.1016/j.cscm.2022.e01520.
- Xiao, J., W. Li, Y. Fan, and X. Huang. 2012. “An Overview of Study on Recycled Aggregate Concrete in China (1996-2011).” Construction and Building Materials 31:364–383. https://doi.org/10.1016/j.conbuildmat.2011.12.074.
- Yang, Y., B. Chen, Y. Su, Q. Chen, Z. Li, W. Guo, and H. Wang. 2020. “Concrete Mix Design for Completely Recycled Fine Aggregate by Modified Packing Density Method.” Materials 13 (16): 3535. https://doi.org/10.3390/ma13163535.
- Zaetang, Y., V. Sata, A. Wongsa, and P. Chindaprasirt. 2016. “Properties of Pervious Concrete Containing Recycled Concrete Block Aggregate and Recycled Concrete Aggregate.” Construction and Building Materials 111:15–21. https://doi.org/10.1016/j.conbuildmat.2016.02.060.
- Zhang, H., L. Wang, and W. Shi. 2023. “Seismic Control of Adaptive Variable Stiffness Intelligent Structures Using Fuzzy Control Strategy Combined with LSTM.” Journal of Building Engineering 78 (August): 107549. https://doi.org/10.1016/j.jobe.2023.107549.
- Zhang, J., Y. Zhao, X. Li, Y. Li, and H. Dong. 2021. “Experimental Study on Seismic Performance of Recycled Aggregate Concrete Shear Wall with High-Strength Steel Bars.” Structures 33 (December 2020): 1457–1472. https://doi.org/10.1016/j.istruc.2021.05.033.
- Zhao, Y., J. Gao, F. Chen, C. Liu, and X. Chen. 2018. “Utilization of Waste Clay Bricks as Coarse and Fine Aggregates for the Preparation of Lightweight Aggregate Concrete.” Journal of Cleaner Production 201:706–715. https://doi.org/10.1016/j.jclepro.2018.08.103.
- Zheng, L., H. Wu, H. Zhang, H. Duan, J. Wang, W. Jiang, B. Dong, G. Liu, J. Zuo, and Q. Song. 2017. “Characterizing the Generation and Flows of Construction and Demolition Waste in China.” Construction and Building Materials 136:405–413. https://doi.org/10.1016/j.conbuildmat.2017.01.055.
- Zhou, C., and Z. Chen. 2017. “Mechanical Properties of Recycled Concrete Made with Different Types of Coarse Aggregate.” Construction and Building Materials 134:497–506. https://doi.org/10.1016/j.conbuildmat.2016.12.163.
- Zhou, Y. W., X. M. Liu, L. L. Sui, F. Xing, and H. J. Zhou. 2015. “Stress–Strain Model for Fibre Reinforced Polymer Confined Load-Induced Damaged Concrete.” Materials Research Innovations 19 (sup6): S6–125. https://doi.org/10.1179/1432891715Z.0000000001464.