Abstract
Nanoparticles (NPs) have emerged as extremely promising materials to alter and improve the properties of liquid crystals (LCs) used, for example, in device applications. In this paper, we summarise recent work from our lab that aims to provide a fundamental understanding of structure–property and composition–property relationships governing LC–NP interactions, which may point to new directions for major improvements in the efficiency of LCs used in display applications. A variety of LC hosts (phases) doped with surface-functionalised gold NPs have been systematically studied ranging from one-dimensionally ordered nematic over two-dimensionally ordered smectic to three-dimensionally ordered columnar phases. Significant progress with respect to LC–NP interactions was made for NP-doped nematic phases. Here, the observation of an unusual texture for Au NP-doped nematic LCs, that is, the formation of birefringent stripe textures and the induction of homeotropic alignment of the nematic LC similar to chiral finger (or fingerprint) textures, provided the basis for numerous experimental studies using Au NPs with different core sizes and surface functionalities.
1. Introduction
In recent years, liquid crystal (LC)–nanoparticle (NP) composites have drawn significant attention and are now one of the hot topics in LC research (Citation 1 , Citation 2 ). The study of LC–NP systems allows for investigating a mutually beneficial combination of the unique properties of LCs and particles at the nanoscale. On the one hand, NP properties can be tuned by surrounding them with LCs, introducing changes such as the localised surface plasmon resonance (SPR) of metal NPs (Au, Ag, Pt) (Citation 3 ). More importantly, LCs are unique candidates to guide NP assembly, which is important for manipulating the spatial arrangement of NPs, as well as their related unique electronic and optical properties with respect to constructing novel nanoscale devices (Citation 4 , Citation 5 ). On the other hand, the properties of LCs can also be affected by NPs such as, for example, the electro-optic properties and the alignment of LCs used in display applications (see Qi and Hegmann (Citation 6 ) and references therein).
Starting in 2004, our group at the University of Manitoba began to systemically study LC–NP composites, with NPs including metal NPs (Au, Ag) and semiconductor quantum dots (CdTe, CdSe) as additives for rod-like nematic and smectic, T-shaped bolaamphiphilic, as well as bent-core LCs (Citation 7 – Citation 16 ). In particular, the results of early experiments on nematic LC–Au NP composites turned out to be of significant relevance. Doping alkylthiol-capped Au NPs into nematic LCs resulted in the formation of unusual textures featuring the formation of birefringent stripe patterns and the induction of homeotropic alignment, altogether resembling chiral finger (or fingerprint) textures (Citation 7 ).
The first part of this paper will provide a brief literature review of LC–NP composites, but focus only on a few key examples. This short review is not intended to give a comprehensive overview of the large body of published work, which readers can find in recent review articles (Citation 1 , Citation 2 ). Rather, these examples were selected to help the reader position our work in the big picture of recent advances in LC–NP composites. The second part of this paper will then review our own work on LC–Au NP composites, with a special focus on nematic LC materials. Finally, a brief summary and an outlook to possible future work will follow at the end.
2. Liquid Crystal–nanoparticle composites
2.1 Tuning NP properties using LCs
The assembly of NPs has been extensively studied over the past two decades, particularly with the aim of forming ordered and predicable arrays that can utilise the unique properties of NPs, as well as the collective properties of NP arrays for the construction of nano-devices (Citation 4 , Citation 5 ). LCs are unique candidates due to the great variety of mesophase morphologies and fast response to external stimuli, such as temperature and electric or magnetic fields (Citation 17 ).
To introduce anisotropic and self-assembling properties of organic LCs to NPs, one approach is to directly link NPs to LCs. Kanayama et al. (Citation 18 ) were the first to report an example of Au NPs covered (protected) with LC molecules using the Brust–Schiffrin two-phase method to synthesise such LC-coated Au NPs (Citation 18 ). As revealed by differential scanning calorimetry (DSC), the prepared NPs showed a double melting that was associated by the authors with mesomorphic properties of the Au NPs, although a typical LC texture was not observed. Using post-synthesis modification of Au NPs (i.e. Au NPs modified using a place exchange reaction with a thiolated thermotropic LCs in a side-on fashion), Cseh and Mehl (Citation 19 ) and Zeng et al (Citation 20 ) observed the formation of typical nematic marble and schlieren textures. More recently, the same group discovered three-dimensional ordering of the NPs within this nematic phase. Aside from Au NPs, other spherical NPs have also been investigated for their ability to form LC phases. In particular, fullerenes forming LC phases have been extensively studied (see Campidelli et al. (Citation 21 ) and references therein).
Many NPs intrinsically self-assemble. In some cases, certain types of LC ordering (mostly nematic and smectic) were observed (usually short range) (see, for example, Song et al. (Citation 22 ), Zhang and Kumar (Citation 23 ), Gabriel et al. (Citation 24 ) and Meuer et al. (Citation 25 )). In fact, in the 1940s, Onsager (Citation 26 ) theoretically assessed the formation of LC phases by rigid-rod objects. This theory predicts that a nematic phase can be formed in concentrated suspensions of rod-like materials above a certain threshold concentration, provided the aspect ratio of the rods is large enough.
Particles (not restricted to the nano-size) doped into LC hosts have been extensively studied, starting with the formation of defects and controlling the defect location, over movement and assembly of NPs in LC phases, to altering of electro-optic properties of these composites. Published work describing these or related effects will be discussed in the next section. There are also several examples of using LC materials to assemble NPs directly without describing electro-physical properties or defect formation. For example, Lynch and Patrick (Citation 27 ) demonstrated the use of thermotropic LCs for producing organised carbon nanotube arrays on surfaces. Dierking et al. (Citation 28 ) used the nematic mixture E7 to control the alignment and re-orientation of carbon nanotubes. Using lyotropic LCs to separate and align carbon nanotubes has also been of significant interest. For a review paper, see Lagerwall and Scalia (Citation 29 ).
LCs can not only be used to guide the assembly of NPs, but also employed to tune other NP properties. For example, Koenig et al. (Citation 30 ) reported that the SPR of Au NPs immersed in LCs can be tuned by a change of the ordering of the LC molecules around the NPs (i.e. planar or homeotropic orientation or anchoring). Tong and Zhao (Citation 31 ) used a LC gel to modulate the luminescence intensity of core-shell CdSe/ZnS quantum dots.
2.2 Modulating LC properties using NPs
Theoretical studies concerned with doping particles into a LC matrix date back to work in the 1970s by Brochard and de Gennes (Citation 32 ) with the aim of lowering the magnetic field intensities required for LC (re)-orientation. In recent years, composite systems, that is, a LC host doped with particles (in most cases, the particles studied were not at the nanoscale), have been widely studied with respect to LC defect formation induced by the immersed particles (see Stark (Citation 33 ) and references therein). The majority of studies dealt with non-chiral nematic LCs (see, for example, Poulin et al. (Citation 34 ) and Kossyrev et al. (Citation 35 )), but also included chiral nematic (Citation 36 ) and lyotropic LC phases (Citation 37 ). Particles studied included emulsions of water microdroplets (see, for example, Poulin et al. (Citation 34 ) and Kossyrev et al. (Citation 35 )), silicon oil (Citation 38 ), gold-coated glass spheres (Citation 39 ), magnetic particles (Citation 40 ), silica particles (Citation 41 ) and ZnS nanocrystals (Citation 42 ), among others. Depending on the chemical environment and the size of particles, surface anchoring of LC molecules is different. Thus, different types of localised defects were observed, including hedgehog, Saturn ring and boojum defects (see Stark (Citation 33 ) and references therein). Recently, laser beam steering (optical tweezers) have also been successfully applied to control particles and defects in LC matrices (Citation 43 ).
Using the forces created by the formation of defects, together with the tendency to minimise the LC's elastic distortion (approaching minima in the elastic energy landscape), particles of different size and shape have been moved (dragged) within LC host matrices. Examples for these particle motions in LCs include the levitation of metal nanowires (Citation 44 , Citation 45 ), the electro-rotation of glass beads (Citation 46 ) and shape-controlled colloid interaction (Citation 47 ).
Contrary to the approach of using LC-decorated NPs for the assembly of NPs, the preparation of LC–NP composites by doping NPs into LC hosts has been studied by a large number of groups. Significant attention has here been paid on altering display-related electro-optic properties of the LC host phase. The reported effects include increasing electrical conductivity in nematic LCs (Citation 48 ), lower threshold voltages in nematic, ferroelectric and discotic LCs (Citation 49 –Citation 51 ) and controlling the alignment of nematic LCs (Citation 52 –Citation 54 ), as well as a memory effect related to ferroelectric LCs (Citation 55 , Citation 56 ). Several types of particles have been extensively studied, and these include carbon nanotubes as well as silica, ferroelectric and metal NPs (see Qi and Hegmann (Citation 6 ) and references therein).
It seems quite obvious that LC–NP composites have been a key focus over the past decade. Numerous new phenomena, as well as applications, emerged from these studies. Research in this field is ongoing and growing worldwide. More focused studies are needed to drive economical breakthroughs of LC–NP composites that would have to focus on such possibilities as macroscopic alignment of LC–NP hybrids, detailed structure–property relationship studies, theoretical investigations of defects in LCs with nano-sized particles and the prevention of NP aggregation in bulk LC phases.
3. Liquid crystal–Au nanoparticle composites: summary of our research results
3.1 Bolaamphiphilic LC/Au NP composites
Starting in 2004, different LC hosts doped with functionalised Au NPs have been systematically studied as part of Hao Qi's thesis, ranging from one-dimensional ordered LCs (nematic), over two-dimensional (smectic), to three-dimensional (columnar) ordering. One particular type of LC host was chosen first, so-called bolaamphiphilic LCs. Earlier work by Cheng et al. (Citation 57 ) and Kölbel et al. (Citation 58 , Citation 59 ) demonstrated that changing the length of a lateral alkyl chain in these molecules produces a different LC phase morphology, along with different phase transition temperatures. The phases formed range from a smectic-A (SmA) phase, over a rectangular columnar phase to a hexagonal columnar phase. Two kinds of LCs were chosen from this series, one forming a SmA phase and a second one forming a rectangular columnar phase (). Using these two LC materials, the effects of hydrophilic and hydrophobic Au NPs on the stability and ordering of these two LC hosts were studied () (Citation 8 ).
Figure 1. (a) Structure and LC phase morphology of the SmA and Colr (c2mm) phases formed by LC 1 and LC 2 (abbreviations: SmA = smectic-A phase, Colr = rectangular columnar phase). (b) Au NPs Au1 and Au2 used in dispersions with the bolaamphiphilic LC 1 and LC 2. (c) Stability of the columnar phase of LC 2 versus concentration of suspended Au NPs obtained by DSC: Δ: high-T onset of the phase transitions, first heating; □: peak maximum, first heating; ◊ peak maximum, first cooling: (left plot) Au1 in LC 2, (right plot) Au2 in LC 2. Reproduced with permission from Qi et al. (Citation 8 ).
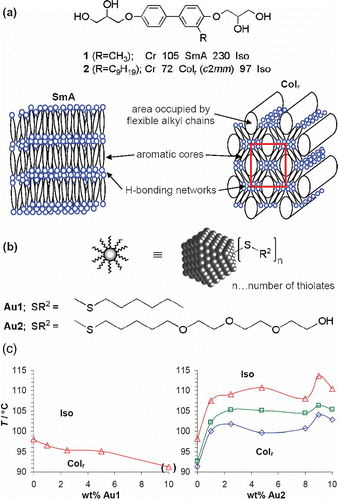
The results indicated random inclusion of the NPs in the LC matrix with a NP concentration- and monolayer-type-dependent effect on the mesophase stability of the LC host. Both types of nanoclusters showed no obvious effect on the thermal properties of the LC forming a SmA phase (LC 1). In contrast, for LC 2 forming a rectangular columnar phase, the LC phase is gradually destabilised with an increasing concentration of the hydrophobic hexane thiolate gold clusters, but gradually stabilised with an increasing concentration of the hydrophilic ethyleneglycol-terminated gold nanoclusters. In addition, mixtures of 9–10 wt% of Au2 in LC 2 showed no texture resemblance to pure LC 2 or LC 2 with lower particle content; that is, no dendritic or pseudo-focal conic domains were observed. Structural characterisation using small-angle x-ray scattering (SAXS) indicated no change in the lattice of the rectangular columnar phase in mixtures with both NPs, but provided evidence for a macroscopic phase separation into particle-rich and particle-poor domains with the same rectangular lattice but slightly different lattice parameters for mixtures with higher concentrations of the hydrophilic Au NPs (Au2). This study provided the first data hinting at the critical role of the NP capping agent in mixtures with LC phases of different symmetry. The LC materials used in this study also present by far the most delicate LC hosts we used for LC–NP composites. The results show that both the LCs (e.g. different LC phase; SmA versus Corr) and the NPs (hydrophobic versus hydrophilic) will have great impact on the properties of LC–NP composites.
3.2 Nematic LC/Au NP composites
As highlighted already in the introduction, nematic LCs are the most widely investigated systems with respect to defect formation as a result of NP doping. Surprisingly, even after more than a decade and a half of extensive studies of LC defect formation as a result of particle inclusion (see Stark (Citation 33 ) and references therein; research starting in 1994 (Citation 4 , Citation 5 )), to the best of our knowledge, no prior study reported similar defect or pattern formation by doping Au NPs into nematic LC hosts. In general, prior to 2004, there were only a very few experimental and theoretical studies concerned with doping LCs using well-dispersed nano-sized particles.
To investigate this, we chose three LC materials (LC 1–3, ), with two nematic LC hosts also forming a SmA phase below the nematic (Citation 7 ). In analogy to the bolaamphiphilic LCs we used in the first study, we were interested if the more ‘rigid’ layer structure of the SmA phase will respond differently to the doping with NPs in comparison to the less ordered, more fluid nematic phase.
Figure 2. (a) Structures and phase transition temperatures (°C) of LC 1–LC 3 (Cr = crystalline solid, SmA = smectic-A phase, N = nematic phase and Iso = isotropic liquid phase) and the structure of (S)-Naproxen. (b) General (truncated) cuboctahedral shape of the Au NPs, and capping of the Au NPs used for dispersion. Reproduced with permission from Qi and Hegmann (Citation 7 ).
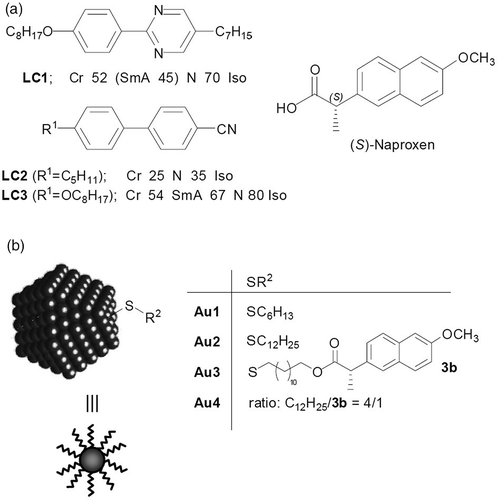
The results were not what we expected. While we did not observe any texture change in the SmA phase (we also investigated another LC material with a Cr-SmC-SmA-Iso phase sequence, unpublished result) and there was no dramatic change in the phase transition temperatures of the nematic phase, the textures we observed after NP doping were significantly different from the texture of the pure nematic host. The first set of Au NPs we used in this study that showed this effect were ‘simple’ hexane (Au1) and dodecane- (Au2 in ) thiolate-protected NPs (). The textures observed for these nematic liquid crystal (N-LC)/NP composites featured the formation of uniform birefringent stripes separated by areas of homeotropic alignment, quite similar to chiral finger textures, although neither the LC nor the NPs were chiral (). In parallel, we also investigated another set of NPs, that is, (S)-naproxen-functionalised Au NPs (Au3, Au4) with the purpose of studying the possibility of transferring chirality from Au NPs to N-LC hosts. Maybe not surprisingly, we found the same chiral-like textures as in the case of Au1 and Au2 doped in these N-LCs. Furthermore, similarly, characteristic textures were also observed for mixtures of the chiral nematic phase produced by doping either only the (S)-naproxen-functionalised dodecane thiol or (S)-naproxen and, additionally, dodecylthiol-protected gold NPs (Au2 in ).
Figure 3. POM photomicrographs (crossed polarisers) of the N phase taken on cooling from below the Iso–N phase transition (T NI): (a) schlieren texture of LC 1 (68°C); (b) fingerprint texture of the N* phase of LC 1 doped with 5 wt% (S)-naproxen (44°C); (c) stripe texture and coexisting areas with homeotropic alignment of the N phase of 5 wt% Au2 in LC 1 (64°C); (d) 5 wt% Au2 in LC 1 at different areas (61°C); (e) 5 wt% Au3 in LC 1 (51°C, large circular areas are air bubbles in the LC film); (f) fingerprint texture of the N* phase of LC 1 doped with 5 wt% of (S)-naproxen-functionalised dodecane thiol (54°C); (g) coexisting finger texture of sample in (f) at different area (56°C); (h) finger-like texture of the N* phase of 5 wt% Au2 in LC 1 doped with 5 wt% (S)-naproxen (55°C, inset: magnified area); (i) stripe pattern of the N phase of 5 wt% Au2 in LC 1 in rubbed polyimide indium tin oxide (ITO) glass cell (cell gap: 5 μm). Reproduced with permission from Qi and Hegmann (Citation 7 ).
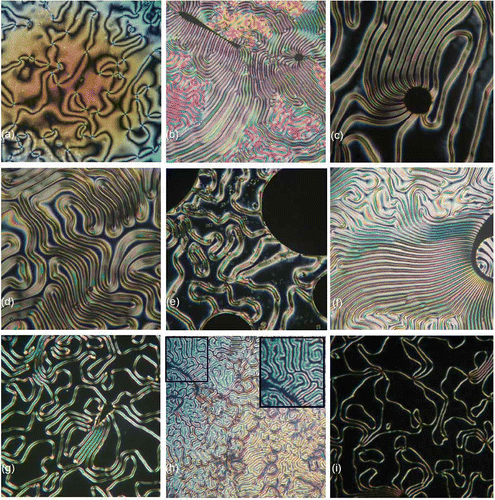
After observing these intriguing textures, two questions arose quickly: (1) Are these textures chiral textures, and if so, what could be the cause? (2) Are the used Au NPs (especially Au1 and Au2 in ) truly non-chiral or racemic NPs, or did we observe a chiral bias such as some chiral impurities within these NP systems? A careful literature review revealed several theoretical studies of Au NPs pointing out intrinsic chirality related to the packing of Au atoms in Au NPs (not due to chiral thiols protecting the Au NP surface) (Citation 60 ). Assuming the theoretical studies are true fact, overall, the Au NPs must be racemic if there is no chiral bias in the synthetic environment, which was experimentally confirmed two years after we published our results (Citation 61 ). To characterise the electronic and chiral properties of all Au NPs used in this study (Au1–Au4), we performed circular dichroism (CD) spectropolarimetry experiments.
CD spectra confirmed the optical activity for both (S)-naproxen-functionalised gold NPs (Au3 and Au4). However, we also observed small CD signals around 400 nm for all Au NPs. These rather small signals were attributed to scattering artefacts of dense particles aggregating in solution. On the basis of these findings, two different scenarios were suggested. In the first scenario, the optically active Au NPs Au3 and Au4 transfer chirality to the non-chiral nematic LC host. In the second scenario, all functionalised Au NPs Au1–Au4 form topological defects resulting in chain-like particle aggregates, separated by areas of homeotropic alignment due to particles residing at the LC–glass interface (). Differentiating between and understanding both suggested scenarios largely motivated the research that followed.
Figure 4. Simplified model for the organisation of the Au NPs in the N-LCs (not to scale). (a) Isolated stripe domain (centre) between two homeotropically aligned domains (left and right); (b) top view indicating particle-rich (stripe domain) and particle-poor areas (homeotropic domains) – LC molecules are omitted for clarity; (c) three-dimensional model with Au NPs residing at both glass–N-LC interfaces in the homeotropic domains. Reproduced with permission from Qi and Hegmann (Citation 7 ).
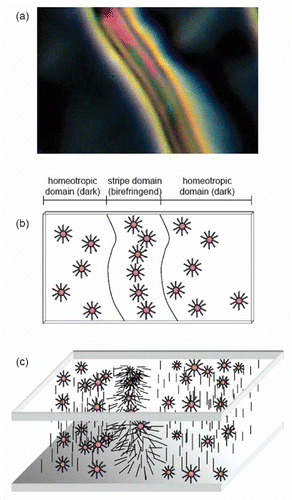
Induced circular dichroism (ICD) experiments provided clear evidence supporting the first scenario. ICD spectra obtained from thin films of the LC–chiral Au NP composites (i.e. (S)-naproxen-functionalised Au NPs, Au3 and Au4, as shown in ) clearly demonstrated a chirality transfer from the chiral Au NPs to the bulk N-host, in comparison to the pure LC, as well as to LC–non-chiral Au NP composites (hexane thiolate-protected Au NPs Au1, as shown in ) (Citation 9 ). 5CB, which shows a nematic phase at room temperature, was used as a LC host. All samples (both pure LC and the LC–NP mixtures) were sandwiched between two quartz slides. CD measurements were pursued with the light path perpendicular to the sample surface. Samples were also rotated in 45° intervals to eliminate contributions from birefringence and linear dichroism. As shown in , both Au3- and Au4-doped 5CB mixtures show positive CD signals at any sample rotation angle, thus showing the transfer of chirality from the chiral Au NPs to the N-LC host. To the best of our knowledge, this was the first example demonstrating the transfer of chirality from suspended NPs to a bulk LC host. It is worth mentioning that such CD studies require a careful interpretation of the measured data including the elimination of other effects, such as linear dichroism, birefringence and local as well as sample-to-sample variations in film thickness (). Thus, care needs to be taken prior to claiming a chiral induction in such thin-film samples. Particularly noteworthy was the observation that the LC–NP composite containing (S)-naproxen-capped Au NPs show throughout positive CD signals, in comparison to 5CB doped with just (S)-naproxen showing always negative CD signals. One could possibly argue about a different chirality transfer mechanism, but similar results have been reported for other chiral macromolecules in the past. Nevertheless, this study presented a new possibility of using chiral NPs in LC-related application, such as the use of NPs as chiral dopants.
Figure 5. CD spectra at different sample angular rotation angles of (a) 5CB doped with 5 wt% Au3, (b) 5CB doped 1 wt% Au3, (c) 5CB doped with 5 wt% Au4 and (d) 5CB doped with 0.5 wt% Au4. All measurements were taken at room temperature. Reproduced with permission from Qi et al. (Citation 9 ).
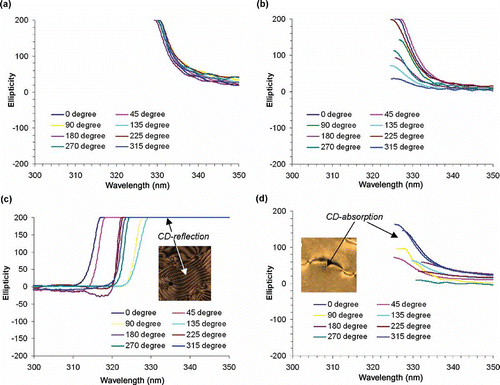
The second scenario, that is, defect formation, described earlier has become the main research theme in our group ever since its inception. Polarised optical microscopy (POM) and fluorescence confocal polarising microscopy (FCPM) experiments pursued in collaboration with the Kitzerow group in Paderborn were shining more light onto this pattern formation. Results of two- and three-dimensional imaging indicate that the NP-induced birefringent stripes correspond to twist disclinations () located at the LC–substrate interface. The luminescence of dispersed semiconductor quantum dots (CdSe) shows that the ends of disclination threads are pinned to conglomerates of NPs, which stabilise these line defects (Citation 62 ).
Figure 6. Schematic drawings of possible director configurations that can lead to birefringent stripes appearing in a homeotropically aligned nematic liquid crystal. (a) Director field with bend deformation. (b) Splay, twist and bend deformation. (c) Twist deformation. Adapted from Urbanski et al. (Citation 16 ).
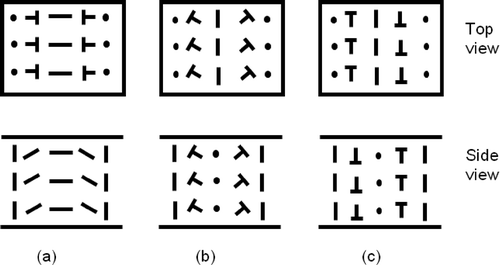
Returning to the observed birefringent stripe textures (–), we then focused our attention to the domains showing homeotropic alignment of the nematic LCs. This led us to demonstrate that this phenomenon can be exploited to promote and control the alignment of nematic LC molecules via NP doping. Traditionally, LC alignment is a necessity for display application of LCs (with the exception of blue phases). To macroscopically orient LC molecules, substrate treatment is normally necessary (Citation 63 ). For example, LC molecules can be aligned in a planar orientation using rubbed polyimide surfaces, and given a N-LC with positive dielectric anisotropy, they can be re-oriented to a vertical orientation by applying an electric (or magnetic) field above a certain threshold (a Freedericksz transition). LC molecules can also be aligned vertically (homeotropic alignment), for example using substrates treated with a surfactant, and N-LCs with a negative dielectric anisotropy can be re-oriented to a planar orientation accordingly under an applied electric field (Citation 64 ). Using NPs as dopants to control the orientation of LCs without using any surface or substrate treatment has not been explored to any great extent before. Thus, we were intrigued whether the textural phenomena we observed could be applied for such LC alignment applications.
As shown in , at the initial stage, after filling the planar cell with the LC–Au NP mixture (LC 1 and Au2, as shown in , were used here), the entire cell area (with the exception of the birefringent stripes) is homeotropically aligned despite the rubbed polyimide alignment layers commonly favouring planar alignment of rod-like N-LCs. Quite surprisingly, considering the use of a N-LC with positive dielectric anisotropy, the LC molecules can still be re-oriented to assume planar orientation after applying an electric field. Moreover, heating the LC–Au NP mixture above the nematic-isotropic phase transition temperature, followed by cooling to the nematic phase under an applied electric field, resulted in the generation of planar alignment, which was stable also in the absence of an applied electric field (bistable). Similar to the non-doped, pure N-LC in a planar cell, applying an electric field to this, now planar-aligned, nematic LC–Au NP composite film could again be re-oriented in the bulk to a vertical (homeotropic) state – a conventional Freedericksz transition for a N-LC with positive dielectric anisotropy. Thus, we succeeded to demonstrate a unique dual alignment and electro-optic switching mode in planar LC cells (Citation 10 ). By fine-tuning experimental conditions (temperature, electric field and alignment), N-LCs doped with Au NPs can be aligned and electrically re-oriented either like N-LCs, with a positive dielectric anisotropy in a planar cell or, alternatively, as N-LCs, with a negative dielectric anisotropy in a homeotropic cell, both at lower threshold voltages than the pure LC host (Citation 10 ).
Figure 7. Demonstration of the initial orientation and electric-field-driven reorientation of the N-LC molecules under cell preparation conditions described in the experiment, that is, filling and cooling the cell at field-OFF (upper section), and after heating above the clearing point and subsequent cooling to the N-phase at field-ON (lower section). Representative thin-film textures (as observed between crossed polarisers) accompany each model. Reproduced with permission from Qi et al. (Citation 10 ).
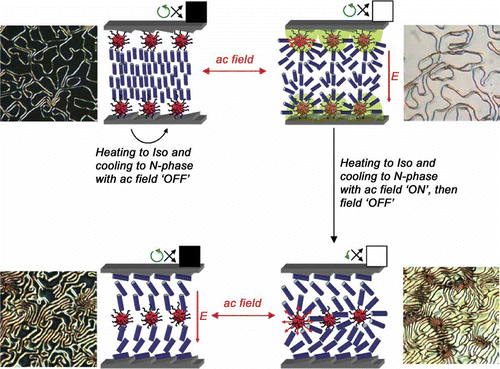
To explain this phenomenon, considering all qualitative optical observations and quantitative electro-optical data we collected, we have formulated a model that, in principle, highlights the interplay and competition between the various perturbations (interactions) exerted on the N-LC molecules by the alignment layers, applied electric field, anchoring to the NP surface and a local contact between the N-LC and the charged (capacitive charging of Au NPs in the applied electric field) and conducting Au NPs in the cell's field-ON or field-OFF states (). In the first set of experiments (initial homeotropic alignment, top section in ), the majority of Au NPs, initially well dispersed in the isotropic state, migrated to the LC-substrate (alignment layer) interface at the phase transition to the nematic phase on cooling. The alkyl chains on the NP surface are most likely responsible for the induced homeotropic alignment of the N-LC molecules, similar to the case of a self-assembled monolayer (SAM) on a gold surface (Citation 65 ). Upon applying an electric field, the electronically charged Au NP cores may behave like local capacitors, promoting the re-orientation of the LC molecules. After cooling the cell under an applied electric field, the Au NPs are ‘pushed’ to the bulk of the N-LC film. At this stage, the polyimide layer, not the Au NPs, control the alignment of the LC molecules, and the switching under an applied electric field is analogous to a pure, non-doped N-LC, with a lower threshold voltage as a benefit arising from the electronic property of the suspended Au NPs (Citation 66 , Citation 67 ).
This dual alignment phenomenon has also been further investigated in our laboratory, and in collaboration with the Kitzerow group (Citation 16 ). The results of this work showed that the reverse switching behaviour of Au NP–LC composites might be coupled to electrohydrodynamic instabilities (or convection rolls).
Detailed alignment and electro-optic studies have also been extended to Ag NPs and quantum dots (CdSe, CdTe), which implies that this phenomenon is likely universal to NPs with a metal or semiconductor core at a certain size regime, and with a certain degree of solubility in a given N-LC host (Citation 11 ). Indeed, in the same period of time, a series of studies by Jeng et al. (Citation 52 ), Kuo et al. (Citation 53 ) and Teng et al. (Citation 54 ) showed that NPs made of polyhedral oligomeric silsesquioxanes also induce vertical alignment of LCs. The authors proposed that this NP-induced alignment phenomenon could be used in areas such as flexible or guest-host LC displays (Citation 52 –Citation 54 ).
In a continuation of this study, we also examined the effect of different concentrations of Au NPs in the N-LC host, and observed a thermally controlled alignment switch phenomenon at lower concentrations of suspended Au NPs (i.e. around 1–2 wt%), which we found to be concentration dependent and thermally reversible (Citation 14 ). As shown in , for LC 1 (as shown in ) doped with 1 or 2 wt% of dodecane thiolate-protected Au NPs Au2 (in ), within the nematic temperature range the LC texture changes from a typical nematic schlieren texture at higher temperatures to vertical alignment with very few birefringent stripe defects as the temperature was lowered by a few degrees. Observing the texture in with a parallel polariser () shows no noticeable NP aggregation for this sample. This thermal switch from parallel (schlieren texture, optical ON state) to vertical alignment (optical OFF state) is likely related to the change of solubility of the NPs in the N-LC host. At higher temperatures, the NPs are better dispersed in the LC host, and the LC molecules interacting with the glass surface dominate the alignment. At lower temperatures, the increased order (i.e. higher order parameter, S) of the nematic phase would lower the ‘solubility’ of the NPs in the N-LC host and expel a certain amount of the NPs to the glass–LC interface. As the result, the NPs control the LC alignment at this stage. Another possibility, which we cannot excluded, is a change in the temperature-dependent ordering of the LC molecules near the NPs that are permanently localised near the LC/alignment layer interface. This thermal switch is reversible (–) and was observed with both untreated microscopy glass slides and in LC test cells with rubbed polyimide alignment layers. This thermally dependent alignment switch phenomenon could potentially be exploited for sensor applications.
Figure 8. POM photomicrographs: LC 1 with 2 wt% Au2 (from ) from cooling at: (a) 64.0, (b) 61.0, (c) 58.9 and (d) 56.0°C with crossed polarisers, and at (e) 56.0°C with parallel polarisers (lower light intensity). The same area observed on heating at (f) 59.0 and (g) 62.0°C. LC 1 with 1 wt % Au2 from cooling at (h) 49.8 and (i) 48.5°C (white or black arrows indicate polariser and analyser position). Reproduced with permission from Qi and Hegmann (Citation 14 ).
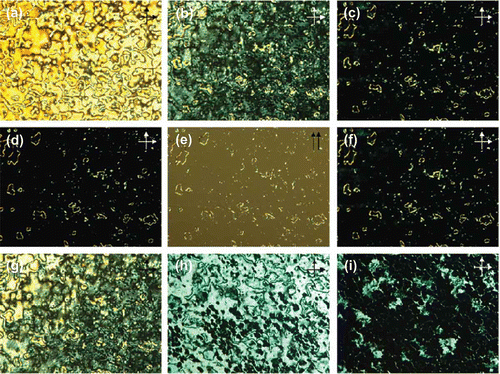
To use LC–NP composites for commercial LC applications, one has to overcome a number of critical requirements. One of these requirements is the long-term stability of the NP dispersions in LC hosts, for example, to electric fields, elevated temperatures and manufacturing conditions. It is widely believed that decorating nanomaterials with liquid crystalline surface ligands would help improve solubility and miscibility of the NPs in the nematic solvent, and hence the stability of the final dispersion (Citation 17 ). An assessment of the validity of this assumption was made by introducing Au NPs capped with liquid crystalline, nematic cyanobiphenyl thiols () (Citation 13 ). Surprisingly, our experiments revealed that common miscibility rules known from organic dopants in nematics do not strictly apply to Au NPs in nematic phases, because these CB-decorated Au NPs (Au3, Au4, as shown in ) showed an inferior solubility in CB hosts (e.g. 5CB) than Au NPs decorated with ‘simple’ alkanethiols (Au1 and Au2 in ). Images showing these mixtures in V-shaped sample preparation vials are shown in and . More details, including LC textures of these mixtures, can be found in Qi et al. (Citation 13 ). Based on these results, we argued that tuning rather than improving the compatibility will result in NP–LC formulations that could help improve the characteristics of LC mixtures used in current and future LC display modes and other related applications.
Figure 9. (a) Structures and LC phase transition temperatures of the LC hosts 5CB and 8CB and of the nematic HS10OCB (top). Schematics of the homogeneous and mixed monolayer gold NPs Au1–Au4 (bottom). V-vials® containing 5CB doped with Au1 or Au3: (b) right after sample preparation, and (c) after standing at room temperature overnight (in the nematic phase of 5CB). The red arrow shows the settling of Au3 in 5CB after about 12 hours. Reproduced with permission from Qi et al. (Citation 13 ).
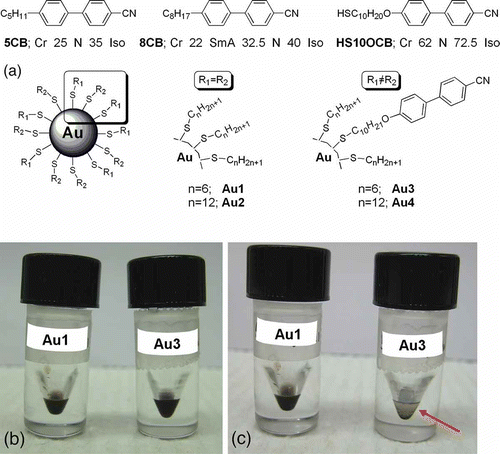
Finally, a rather independent report from our group following a series of studies on nematic LC–Au NP composites presents results that contributed to the understanding of the origin of chirality of chiral ligand-decorated Au NPs (Citation 68 ). The chirality of Au NPs has been introduced at our first report on the phenomenon of N-LC–Au NP composites. Apart from the initially puzzling textures we observed for these nematic LC–Au NP composites, the origin of chirality of Au NPs struck us as another intriguing issue. Schaaff and Whetten (Citation 69 ) reported the first example of chiral-ligand protected Au NPs and proposed three possible mechanisms for the origin of NP core chirality – an intrinsic chiral core, a chiral surface and the formation of a chiral supramolecular assembly or chiral footprint – to explain the observed optical response. Until now, the origin and the validity of one or several of these proposed mechanisms have been under debate, despite an increasing number of groups working in this field and a rising number of reports over recent years (for reviews, see Kitaev (Citation 70 ), Noguez and Garzón (Citation 71 ) and Gautier and Bürgi (Citation 72 ); for examples of recent reports, see Slocik et al. (Citation 73 ), Rrovorse and Aikens (Citation 74 ), Shukla et al. (Citation 75 ), Geroge and Thomas (Citation 76 ) and Oh et al. (Citation 77 )). Possibly x-ray crystallographic characterisation of discrete nanoclusters similar to the experiments described by Jadzinsky et al. (Citation 61 ) could help elucidate the detailed structure of chiral ligand-capped Au NPs. A comparison with Jadzinsky's data on non-chiral ligand-protected Au NPs would surely be a key step to solve this puzzle. In our contribution to comprehend Au NP chirality, we used (S)-naproxen-functionalised chiral Au NPs (similar to those used in earlier studies). We developed a simple, yet effective, methodology by showing that post-synthesis NP ligand shell modifications, such as racemisation and place exchange reactions, as a general approach are very powerful tools to unravel contributions of the different proposed Au NP chirality mechanisms. In essence, we showed that the formation of a supramolecular array of aromatic chiral ligands at the NP corona contributes to the so-called vicinal effect in our particular chiral ligand-capped Au NPs, and that this vicinal effect appears to be the only effect responsible for the observed chirality. Most importantly, however, we believe that the described methodology could easily be adapted to study and understand many other chiral-ligand protected metal NP systems.
4. Conclusions and outlook
Over recent years, LC–NP composites have emerged as one of the hot topics in LC research and development (R&D), with fascinating opportunities for both basic research and applied device applications. Our contributions to this area have been reviewed here, including topics such as tuning the functionality of the Au NP surface, novel alignment and defect formation phenomena for non-chiral nematic LCs doped with non-chiral, as well as chiral, Au NPs, transferring chirality from NPs to bulk N-LC hosts, as well as dual-alignment and thermal alignment toggling phenomena.
There are numerous questions and opportunities that will continue to drive exploration of LC nanoscience. These questions and opportunities include, but are not limited to the follow aspects: (1) the search for a direct proof and visualisation of the NP locations in LC hosts; (2) the development of other chiral ligand-capped nanomaterials that can be used to transfer chirality from NPs to other LC phases, such as the SmA or Smectic-C (SmC) phase (electroclinic and ferroelectric switching); (3) a more extensive and systematic study of the electro-optic properties of LC–NP composites in conjunction with industry partners will offer true benefits to the flat-panel liquid crystal device (LCD) industry; (4) a rational design of nanomaterials with different shapes (e.g. rods), core materials (e.g. Si) and other surface functionalities will provide new opportunities.
At this point in time and considering worldwide research progress in the area of LC nanocomposites, there seems to be enough evidence that LC–NP composite R&D will become one of the most exciting areas within the LC community. The outcomes of these research initiatives will create benefits for both research themes, LCs and nanomaterials. New NP arrays that can be manipulated by electric fields or temperature using LCs will stimulate nanomaterial integration into devices, and new types of nanomaterials will likely lead to more energy-efficient applications of LCs in flat-panel as well as flexible displays, or lead to the creation of novel types of sensors – even for medical applications, such as optical sensing of proteins and other biomacromolecules.
Acknowledgements
This work was financially supported by the Natural Sciences and Engineering Research Council (NSERC) of Canada, by the Canada Foundation for Innovation (CFI), the Manitoba Research and Innovation Fund (MRIF) and the University of Manitoba (via IPM Demonstration Project Grants).
References
- Hegmann , T. , Qi , H. and Marx , V.M. 2007 . J. Inorg. Organomet. Polym. Mater , 17 : 483 – 508 .
- Bisoyi , H.K. and Kumar , S. 2011 . Chem. Soc. Rev , 40 : 306 – 309 .
- Koenig , G.M. Jr. , Gettelfinger , B.T. , de Pablo , J.J. and Abbott , N.L. 2008 . Nano Lett , 8 : 2362 – 2368 .
- Kumar , S. 2006 . Chem. Soc. Rev , 35 : 83 – 109 .
- Daniel , M. and Astruc , D. 2004 . Chem. Rev , 104 : 293 – 346 .
- Qi , H. and Hegmann , T. 2008 . J. Mater. Chem , 18 : 3288 – 3294 .
- Qi , H. and Hegmann , T. 2006 . J. Mater. Chem , 16 : 4197 – 4205 .
- Qi , H. , Lepp , A. , Heiney , P.A. and Hegmann , T. 2007 . J. Mater. Chem , 17 : 2139 – 2144 .
- Qi , H. , O'Neil , J. and Hegmann , T. 2008 . J. Mater. Chem , 18 : 374 – 380 .
- Qi , H. , Kinkead , B. and Hegmann , T. 2008 . Adv. Funct. Mater , 18 : 212 – 221 .
- Qi , H. , Kinkead , B. and Hegmann , T. 2008 . Proc. SPIE , 6911 : 691106
- Marx , V.M. , Girgis , H. , Heiney , P.A. and Hegmann , T. 2008 . J. Mater. Chem , 18 : 2983 – 2994 .
- Qi , H. , Kinkead , B. , Marx , V.M. , Zhang , H.R. and Hegmann , T. 2009 . ChemPhysChem , 10 : 1211 – 1218 .
- Qi , H. and Hegmann , T. 2009 . ACS Appl. Mater. Interfaces , 1 : 1731 – 1738 .
- Kinkead , B. and Hegmann , T. 2010 . J. Mater. Chem , 20 : 448 – 458 .
- Urbanski , M. , Kinkead , B. , Qi , H. , Hegmann , T. and Kitzerow , H.-S. 2010 . Nanoscale , 2 : 1118 – 1121 .
- Goodby , J.W. , Saez , I.M. , Cowling , S.J. , Gortz , V. , Draper , M. , Hall , A.W. , Sia , S. , Cosquer , G. , Lee , S.-E. and Raynes , E.P. 2008 . Angew. Chem. Int. Ed , 47 : 2754 – 2787 .
- Kanayama , N. , Tsutsumi , O. , Kanazawa , A. and Ikeda , T. 2001 . Chem. Commun , : 2640 – 2641 .
- (a) Cseh, L.; Mehl, G. J. Am. Chem. Soc. 2006, 128, 13376–13377; (b) Cseh, L.; Mehl, G. J. Mater. Chem. 2007, 17, 311–315.
- Zeng , X.P. , Liu , F. , Fowler , A.G. , Ungar , G. , Cseh , L. , Mehl , G.H. and Macdonald , J.E. 2009 . Adv. Mater , 21 : 1746 – 1750 .
- Campidelli , S. , Bourgun , P. , Guintchin , B. , Furrer , J. , Stoeckli-Evans , H. , Saez , I.M. , Goodby , J.W. and Deschenaux , R. 2010 . J. Am. Chem. Soc , 132 : 3574 – 3581 .
- Song , W. , Kinloch , I. and Windle , A. 2003 . Science , 302 : 1363 – 1363 .
- Zhang , S. and Kumar , S. 2008 . Small , 4 : 1270 – 1283 .
- Gabriel , J.-C.P. , Camerel , F. , Lemaire , B.J. , Desvaux , H. , Davidson , P. and Batail , P. 2001 . Nature , 413 : 504 – 508 .
- Meuer , S. , Oberle , P. , Theato , P. , Tremel , W. and Zentel , R. 2007 . Adv. Mater , 19 : 2073 – 2078 .
- Onsager , L. 1949 . Ann. N. Y. Acad. Sci , 51 : 627 – 659 .
- Lynch , M.D. and Patrick , D.L. 2002 . Nano Lett , 2 : 1197 – 1201 .
- Dierking , I. , Scalia , G. , Morales , P. and LeClere , D. 2004 . Adv. Mater , 16 : 865 – 869 .
- Lagerwall , J.P.F. and Scalia , G. 2008 . J. Mater. Chem , 18 : 2890 – 2898 .
- Koenig , G.M. , Meli , M.-V. , Park , J.-S. , de Pablo , J.J. and Abbott , N.L. 2007 . Chem. Mater , 19 : 1053 – 1061 .
- Tong , X. and Zhao , Y. 2007 . J. Am. Chem. Soc , 129 : 6372 – 6373 .
- Brochard , F. and de Gennes , P.G. 1970 . J. Phys. (Paris) , 31 : 691 – 708 .
- Stark , H. 2001 . Phys. Rep , 351 : 387 – 474 . and references therein
- Poulin , P. , Stark , H. , Lubensky , T.C. and Weitz , D.A. 1997 . Science , 275 : 1770 – 1773 .
- Kossyrev , P. , Ravnik , M. and Žumer , S. 2006 . Phys. Rev. Lett , 96 : 048301
- Zapotocky , M. , Ramos , L. , Poulin , P. , Lubensky , T.C. and Weitz , D.A. 1999 . Science , 283 : 209 – 212 .
- Mondain-Monval , O. , Dedieu , J.C. , Gulik-Krzywicki , T. and Poulin , P. 1999 . Eur. Phys. J. B , 12 : 167 – 170 .
- Loudet , J.-C. , Barois , P. and Poulin , P. 2000 . Nature , 407 : 611 – 613 .
- Gu , Y. and Abbott , N.L. 2000 . Phys. Rev. Lett , 85 : 4719 – 4722 .
- Poulin , P. , Cabuil , V. and Weitz , D.A. 1997 . Phys. Rev. Lett , 79 : 4862 – 4865 .
- Kreuzer , M. , Tschudi , T. , De Jeu , W.H. and Eidenshink , R. 1993 . Appl. Phys. Lett , 62 : 1712
- Lee , S.-W. , Mao , C.B. , Flynn , C.E. and Belcher , A.M. 2002 . Science , 296 : 892 – 895 .
- Smalyukh , I.I. , Lansac , Y. , Clark , N. and Trivedi , R. 2010 . Nat. Mater , 9 : 139 – 145 .
- Lapointe , C. , Hultgren , A. , Silevitch , D.M. , Felton , E.J. , Reich , D.H. and Leheny , R.L. 2004 . Science , 303 : 652 – 655 .
- Lapointe , C. , Reich , D.H. and Leheny , R.L. 2008 . Langmuir , 24 : 11175 – 11181 .
- Liao , G. , Smalyukh , I.I. , Kelly , J.R. , Lavrentovich , O.D. and Jákli , A. 2005 . Phys. Rev. E , 72 : 031704
- Lapointe , C. , Mason , T.G. and Smalyukh , I.I. 2009 . Science , 326 : 1083 – 1086 .
- Huang , C. , Hu , C. , Pan , H. and Lo , K. 2005 . Jpn. J. Appl. Phys , 44 : 8077 – 8081 .
- Lee , W. , Wang , C. and Shih , Y. 2004 . Appl. Phys. Lett , 85 : 513 – 515 .
- Huang , C. , Pan , H. and Hsieh , C. 2006 . Jpn. J. Appl. Phys , 45 : 6392 – 6394 .
- Scalia , G. , Lagerwall , J.P.F. , Schymura , S. , Haluska , M. , Giesselman , F. and Roth , S. 2007 . Phys. Status Solidi B , 244 : 4212 – 4217 .
- Jeng , S.-C. , Kuo , C.-W. , Wang , H.-L. and Liao , C.-C. 2007 . Appl. Phys. Lett , 91 : 061112
- Kuo , C.-W. , Jeng , S.-C. , Wang , H.-L. and Liao , C.-C. 2007 . Appl. Phys. Lett , 91 : 141103
- Teng , W.-Y. , Jeng , S.-C. , Kuo , C.-W. , Lin , Y.-R. , Liao , C.-C. and Chin , W.-K. 2008 . Opt. Lett , 33 : 1663 – 1665 .
- Huang , C.-Y. , Lai , C.-C. , Tseng , Y.-H. , Yang , Y.-T. , Tien , C.-J. and Lo , K.-Y. 2008 . Appl. Phys. Lett , 92 : 221908
- Sikharulidzea , D. 2005 . Appl. Phys. Lett , 86 : 033507
- Cheng , X. , Prehm , M. , Das , M.K. , Kain , J. , Baumeister , U. , Diele , S. , Leine , D. , Blume , A. and Tschierske , C. 2003 . J. Am. Chem. Soc , 125 : 10977 – 10996 .
- Kölbel , M. , Beyersdorff , T. , Cheng , X. , Tschierske , C. , Kain , J. and Diele , S. 2001 . J. Am. Chem. Soc , 123 : 6809 – 6818 .
- Kölbel , M. , Beyersdorff , T. , Sletvold , I. , Tschierske , C. , Kain , J. and Diele , S. 1999 . Angew. Chem. Int. Ed , 38 : 1077 – 1080 .
- Román-Velázques , C.E. , Noguez , C. and Garzón , I.L. 2003 . J. Phys. Chem. B , 107 : 12035 – 12038 .
- Jadzinsky , P.D. , Calero , G. , Ackerson , C.J. , Bushnell , D.A. and Kornberg , R.D. 2007 . Science , 318 : 430 – 433 .
- Urbanski , M. , Kinkead , B. , Hegmann , T. and Kitzerow , H.-S. 2010 . Liq. Cryst , 37 : 1151 – 1156 .
- Hoogboom , J. , Rasing , T. , Rowan , A.E. and Nolte , R.J.M. 2006 . J. Mater .Chem , 16 : 1305 – 1314 .
- Sage , I.C. 1998 . Handbook of Liquid Crystals , Edited by: Demus , D. , Goodby , J. , Gray , G.W. , Spiess , H.W. and Vill , V. Vol. 1 , 731 – 762 . Weinheim : Wiley-VCH .
- Drawhorn , R.A. and Abbott , N.L. 1995 . J. Phys. Chem , 99 : 16511
- Albrecht , T. , Mertens , S.F.L. and Ulstrup , J. 2007 . J. Am. Chem. Soc , 129 : 9162 – 9167 .
- Boettcher , S.W. , Strandwitz , N.C. , Schierhorn , M. , Lock , N. , Lonergan , M.C. and Stucky , G.D. 2007 . Nat. Mater , 6 : 592 – 596 .
- Qi , H. and Hegmann , T. 2008 . J. Am. Chem. Soc , 130 : 14201 – 14206 .
- Schaaff , T.G. and Whetten , R.L. 2000 . J. Phys. Chem. B , 104 : 2630 – 2641 .
- Kitaev , V. 2008 . J. Mater. Chem , 18 : 4745 – 4749 .
- Noguez , C. and Garzón , I.L. 2009 . Chem. Soc. Rev , 38 : 757 – 771 .
- Gautier , C. and Bürgi , T. 2009 . ChemPhysChem , 10 : 483 – 492 .
- Slocik , J.M. , Govorov , A.O. and Naik , R.R. 2011 . Nano. Lett , 11 : 701 – 705 .
- Rrovorse , M.R. and Aikens , C.M. 2010 . J. Am. Chem. Soc , 132 : 1302 – 1310 .
- Shukla , N. , Bartel , M.A. and Gellman , A.J. 2010 . J. Am. Chem. Soc , 132 : 8575 – 8580 .
- Geroge , J. and Thomas , K.G. 2010 . J. Am. Chem. Soc , 132 : 2502 – 2503 .
- Oh , H.S. , Liu , S. , Lee , H. , Baev , A. , Swihart , W.T. and Prasad , P.N. 2010 . J. Am. Chem. Soc , 132 : 17346 – 17348 .