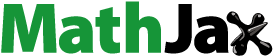
Abstract
Due to their wide range of clinical application possibilities, magnetic actuation technologies have grabbed the attention of researchers worldwide. The design, execution, and analysis of magnetic catheter systems have advanced significantly during the last decade. The review focuses on magnetic actuation for catheter steering and control of the device, which will be explored in detail in the following sections. There is a discussion of future work and the challenges of the review systems, and the conclusions are finally addressed.
Introduction
Early detection and treatment are critical to reducing the number of lung cancer deaths [Citation1,Citation2]. Since the capacity to precisely direct catheters to the regions where they are needed is essential to successful cancer treatment, catheters are typically utilised in clinical practice [Citation3–5].
Catheter technology is continuously evolving [Citation6–8]. In the case of deep-seated tumours requiring catheter insertion, however, there are significant limits to the catheter-guided procedure. A clinician could puncture the tissue if they apply too much effort to the surrounding tissues, which are more sensitive. Catheter steering gets increasingly difficult when the surgeon inserts the catheter further into the patient since typical steering capabilities are restricted. Using a catheter [Citation9] that could be freely steered would assist clinicians in precisely accessing target organs while minimising injury to healthy tissue in the surrounding area.
Electromagnetic coils and permanent magnets are appropriate options for magnetic field production. In order to improve the research and clinical outcomes of magnetic steerable catheters, efficient and appropriate actuation systems must be developed to provide the necessary magnetic fields [Citation10–13].
The search for keywords including “Magnetic” resulted in 128 articles to be reviewed; following the selection of the initial articles, a list of 68 papers was included. Afterwards, articles that did not specify magnetically actuated systems were eliminated, and articles published in a journal were excluded. A total of 46 articles were generated. The magnetically actuated system papers were split into three categories; commercial systems, systems with permanent magnets and systems with electromagnetics, and all articles were studied in detail.
The magnetic actuation catheter system
Through the application of magnetic fields, magnetic actuation technologies allow interventional equipment to be controlled from a distance. For the remote navigation of magnetic catheters, several magnetic actuation methods have been developed. These systems can manipulate magnetic catheters accurately. However, each system has its limitations. lists several magnetic actuation catheter systems described in the literature.
Table 1. lists of the magnetic actuation catheter system presented in the literature.
Commercial magnetic actuation systems
In over a hundred hospitals worldwide, the Niobe system () has been used in over 100,000 patients suffering from heart disease or cancer [Citation24,Citation26]. It comprises two massive permanent magnets, computer-aided motors that mechanically rotate magnets, and magnetic fields generated by the permanent magnets that are applied to guide magnetic catheters. The Niobe system comes with an image fluoroscopy system. The Niobe system’s main drawback is the magnets’ slow rotation speed and the limited response time, which may influence the accuracy and result in a prolonged treatment period.
Figure 1. Commercial magnetic actuation systems. (a) The Stereotaxis Niobe Magnetic Navigation System. The SIEMENS Axiom Artis dFC Single plane D-arm fluoroscopy machine is in the middle [Citation24]. (b) The Catheter Guidance Control and Imaging system [Citation25].
![Figure 1. Commercial magnetic actuation systems. (a) The Stereotaxis Niobe Magnetic Navigation System. The SIEMENS Axiom Artis dFC Single plane D-arm fluoroscopy machine is in the middle [Citation24]. (b) The Catheter Guidance Control and Imaging system [Citation25].](/cms/asset/97f3a92d-3f70-4374-a43f-5880c48dc101/imit_a_2198004_f0001_c.jpg)
In addition to the Niobe system, Genesis is an improved version of Niobe but is smaller, lighter and has greater flexibility in its movement, resulting in an enhanced response. Furthermore, Genesis generates a larger magnetic field by rotating two permanent magnets around its mass centre. This results in less space being displaced and less momentum needed to initiate or change motion, leading to faster speeds and shorter response times as the magnets are rotated effectively.
There are commercially available products for the electromagnet-based distributed actuation system such as Catheter Guidance Control and Imaging (CGCI) () [Citation25]. A set of eight electromagnets arranged in a spherical shape enables the system to produce isotropic magnetic fields, which can be controlled nearly in real-time. As opposed to other moving magnetic actuation systems, the main drawbacks of the fixed position of magnets are the restricted motions
Aeon Phocus is another widely available product (Aeon Scientific AG, Zurich, Switzerland), which has four semi-spherical electromagnets above the body and four symmetrical electromagnets below the torso. Two frames (one with four electromagnets and the other with three) surround the torso while performing magnetic actuation. While the Aeon Phocus has certain efficiency advantages, it also has limitations. Direct access to the patient is critically important during surgeries where interventions are always required for patient monitoring, instrument adjustment and other standard clinical procedures.
Permanent magnets actuation systems
Single magnet
One permanent external magnet is compact in steering, but there are still challenges. For example, the system’s performance is still very uncertain, and extended operating hours and exposure to imaging radiation provide a physical barrier for the operator. Several systems have developed robots featuring commercial manipulators to compensate for these limitations. The conventional computer-assisted surgery-based technique () has been improved for intravascular procedures [Citation17]. Preliminary research has been undertaken to identify the optimal configuration employing a specialised setup for monitoring locomotion and navigating with and against the vascular flow. The empirical findings examine the consequences of dragging a device down and allow clinicians to identify different operating conditions.
Figure 2. Magnetic actuation systems rely only on a single permanent magnet. (a) The robotic platform’s design includes an HMI and two manipulators with 6-DOF each [Citation27]. (b) Schematic diagram of magnetically steerable guidewire actuating system [Citation28]. (c) A system using a robotic arm manufactured by KUKA Robotics Corporation. The internal permanent magnet (IPM) is located at the catheter tip [Citation29]. (d) Robotic manipulator Yaskawa Motoman MH5 6-DOF with single-axis rotary actuator. [Citation30]. (e) Experimental platform from which an EM is controlled by one manipulator and an IM by another. A force and torque sensor detects how much torque is applied to the IM using a wrench [Citation31]. (f) Experimental platform with MFS adjacent to driving magnet location [Citation32].
![Figure 2. Magnetic actuation systems rely only on a single permanent magnet. (a) The robotic platform’s design includes an HMI and two manipulators with 6-DOF each [Citation27]. (b) Schematic diagram of magnetically steerable guidewire actuating system [Citation28]. (c) A system using a robotic arm manufactured by KUKA Robotics Corporation. The internal permanent magnet (IPM) is located at the catheter tip [Citation29]. (d) Robotic manipulator Yaskawa Motoman MH5 6-DOF with single-axis rotary actuator. [Citation30]. (e) Experimental platform from which an EM is controlled by one manipulator and an IM by another. A force and torque sensor detects how much torque is applied to the IM using a wrench [Citation31]. (f) Experimental platform with MFS adjacent to driving magnet location [Citation32].](/cms/asset/b810464f-9929-4b05-9a52-6c920fdcbab9/imit_a_2198004_f0002_c.jpg)
Magnetically steerable guidewires were also introduced [Citation28]. The actuator is built of Ecoflex and magnetic powder and connects to a commercial guidewire. Ecoflex is platinum-catalyzed silicones approved for human skin use [Citation29]. The actuation system’s two symmetrical parts include three linear motors (), allowing multi-directional deflection angles. Magnetic field direction and magnitude are studied regarding the deflection angle. Guidewire’s ability allows it to perform sharp 180-degree turns and navigate through rings and a complicated and confined phantom of blood vessels using magnetic fields that have been configured. Optimising the system’s image processing algorithm will allow the proposed magnetically steerable guidewire to be automatically controlled.
A magnetically driven soft-tethered device () with a new control technique for colonoscopy was then addressed [Citation30]. Actuation is achieved by utilising a serial manipulator to impart force and torque to the internal magnetic device. Another control method was developed, which operates against gravity by having the device float. The experiment confirms the method using a specialised setup comparable to a typical colonoscopy scenario. The catheter was in touch with the tube for an average of 19.5% of the time. The process took an average of 346.78 s, with a standard deviation of 119.37 s. As a result of less interaction with the surroundings, smoother movement can be achieved. The control can also move the device inside a colon phantom, creating a more realistic simulation environment. Untethered magnetic devices (UMD) that utilise a single spinning permanent magnet, on the other hand, are restricted to performing only when the rotating dipole field behaviour is straightforward and clear to see. A limitation of this approach is that it assumes tether-environment interactions are negligible. To include the interactions of the control approaches, such as allowing the tether to map its position and shape, real-time shape sensors installed in the tether could be considered. In the future, more realistic experiments could well be carried out. This restriction presents a single rotating magnet actuator () that can produce a rotating magnetic field in the space approach [Citation33]. The method was proven in an experiment in which a rotating actuator magnet was observed to generate a magnetic field, and both threaded and spherical rolling UMDs were pushed in two different scenarios: one in which the actuator magnet was set to spin in an unspecified direction. Its rotation axis was under automated control, and one in which the actuator magnet was locked in place and its rotation axis was under manual control. The results indicate that the methods can effectively control UMD without needing an untethered rotary actuator magnet.
Additionally, uniform magnetic fields can be generated from the direct orthogonal decomposition of the magnetic field [Citation31]. In order to provide a lateral force, the actuator dipole can be rotated through a spinning magnet dipole. Experiments to validate this approach demonstrate that the lateral force is capable of causing a rotating magnetic device to roll while simultaneously pushing it along a surface, increasing the velocity. In addition, the lateral force magnitude is also adequate to lift the magnetic device and rotating magnetic devices of any size are valid for applications where inertia does not affect dynamics. The magnetic force can be used for levitation and control to minimise the attracting component and increase the device’s rolling velocity.
A magnetic medical robot navigates utilising actuation localisation, ferromagnetic body, and the necessary magnetic field and gradient generation presented [Citation32]. The precision of force transmission is reduced due to the uncertainty in this localisation. Using magnetic force sensitivity ellipsoids, it is feasible to investigate force uncertainty (). The method developed shifting torques while spinning the intracorporeal magnet (IM) to work in the force null space, reducing force uncertainty. When the IM was limited, the force’s uncertainty on the platform decreased. Actuators are magnetically linked to end-effectors; thus, no physical connection is needed. Since magnetically guided catheters have some mechanical limitations on the direction of the guiding IM, this method could be used. Regarding the mobility of magnetic robots, a lower degree of localisation uncertainty will result in greater accuracy. The mobility of magnetic robots will be altered to varying degrees by improved force accuracy, depending on the amount of localisation uncertainty.
The use of local magnetic actuation (LMA) to control surgical tools was presented [Citation34]. An LMA actuation can be felt within the abdominal wall (). In the dynamic model, two approaches were studied: one based on the angular velocity of the motor and the other on the torque load. The dynamic model predicted the load torque with an error of less than 7.5%. Furthermore, both presented closed-loop control approaches effectively managed the technique's higher torque values while displaying less angular velocity dependence. When the two magnets are not stationary or parallel, a further expansion of the approach is recommended. Both horizontal and vertical vibrations must be considered while doing laparoscopic surgery. For example, the model must account for the vertical attraction force between the driving and driven magnets.
Multiple magnets
For torque actuation, multiple magnets generate a magnetic field with excellent uniformity since symmetric distribution helps minimise the field’s gradient. The challenge of multiple degrees of freedom systems with independent control was addressed by utilising local magnetic actuation (LMA) [Citation35]. Multiple-DoF LMAs () are intended to offer a wider variety of applications owing to an effective modular disturbance rejection scheme to deal with unanticipated forces that cause an object to move away from its target; a control technique is used to respond to the disturbance and provides strategies for coping with these unknown forces. This study looks at the link between the LMA and time-varying magnetic disturbances caused by several units. The developed model is used to verify that the state of the inner side of the LMA can be inferred while building the sensor-free manipulator. Successful validation of the presented technique demonstrates the method’s viability. The validation shows that the internal magnet’s estimated rotational velocity and load torque vary from 28 to 47 and 1.18 to 1.41
respectively. The disturbance compensation mechanism also reduces the effect of two LMA units interacting. Shielding has a consistent effect on torque transmission. However, that influence decreases with distance. Because the shielding effect is less than 2.5
it is minor in comparison to the maximum transmittable torques without shielding. Shielding’s impact on torque transmission can be regarded as acceptable. With the present technique, sinusoidal disturbances in multiple rotating magnetic fields could be controlled and compensated for in various applications.
Figure 3. Magnetic actuation systems rely on multiple permanent magnets. (a) Experimental platform with a single DoF of LMA [Citation34]. (b) A rotating magnetic field generator [Citation35]. (c) A prototype system of rotating magnets composed of eight magnets [Citation13]. (d) A magnetic representation of a robotic system. The dashed arrows show that the IPM’s pivot point is subject to various forces and torques. The magnets on the exterior are long-lasting [Citation12].
![Figure 3. Magnetic actuation systems rely on multiple permanent magnets. (a) Experimental platform with a single DoF of LMA [Citation34]. (b) A rotating magnetic field generator [Citation35]. (c) A prototype system of rotating magnets composed of eight magnets [Citation13]. (d) A magnetic representation of a robotic system. The dashed arrows show that the IPM’s pivot point is subject to various forces and torques. The magnets on the exterior are long-lasting [Citation12].](/cms/asset/a0d5483e-3d37-49d4-9630-cfd43175501c/imit_a_2198004_f0003_c.jpg)
The Genesis configuration provides a more uniform magnetic field, resulting in better motion stability. A new approach was developed in which six identical circumferential permanent magnets are placed circumferentially around the circular array () and then adjust each magnet to its original angle before spreading the magnets equally in a circle [Citation14]. The polarity stays constant at the circle’s centre, resulting in a synchronised and constant intensity magnetic field. These techniques are still in their early phases of development; however, they are robust enough to evaluate effectiveness.
Implementing a permanent magnet array allows for the complexity of the driving mechanism to be reduced. Eight independent rotatory permanent magnets (), which enable remote control of a microscale 5-DOF movement of magnetic devices, are developed [Citation13]. An actuation system based on rotating permanent magnets has been created, and it offers control over microscale devices with a higher force in any direction than other magnetic actuation systems. It can control magnetic devices within a 5-DOF. It is shown that the system can be tuned for a high degree of control even when the magnet locations are highly restricted, allowing it to be used in actuators with limited actuator area.
A magnetically actuated 5-DOF wired with stereovision () was also developed [Citation12]. After inserting the system inside the body and manipulating it, the position of the stereo camera can be approximated by moving the external permanent magnets. The internal mechanism allows for precise tilt adjustment from 0-70°, while the external permanent magnets hold the device in place. A method initially created to handle a conflict between surgical tools and vision-based instrumentation was validated in vivo for minimising this conflict and introducing an additional instrument via the same access site. Comparative studies were performed between the presented system and a standard 2-D laparoscope to validate the prototype’s performance. The systems significantly improved speed and efficiency. Image quality can be increased by raising the camera’s resolution. It is also recommended that the robot be made smaller to be connected using a standard surgical trocar and that noise filtering and image sharpness be enhanced. Furthermore, well-arranged magnets can be used to implement specific magnetic fields.
Electromagnetics actuation systems
Paired coil
Systems with paired coils have discal coils that fully encircle the workspace. This magnetic actuation system often uses specialised coils to achieve the desired effect. The most typical kind has perpendicular planes, with the axes of each pair perpendicular to one another. With miniature robots controlled by external magnetic fields, operations may be performed less invasively. However, resistive magnetic devices can generate large-volume, powerful magnetic fields, but they do so inefficiently and use energy and space. The construction and testing of a magnetic manipulator of six electromagnets equipped with cool liquid nitrogen (LN2) () are introduced [Citation36]. Reducing the electrical resistance of copper cables is done using this method. It reduces the quantity of heat produced. Because they are liquid nitrogen-cooled, air-cooled electromagnets are more miniature and require less power. During the experiment, the current and voltage of the electromagnet were monitored. LN2 has been shown to boost current in an electromagnet by 435%, increasing the magnetic field strength and leading to a higher steering ability. Air-cooled cases need an average of 0.218 W of instantaneous power, but LN2-cooled magnets require only 0.0378 W. The power reduction in results in a decrease in applied voltage. However, if a significant volume of liquid nitrogen evaporates, the oxygen level in the environment could decrease. In order to prevent anoxia, there should be a feature of a ventilation system and a low oxygen alert.
Figure 4. Magnetic actuation systems that rely on a pair coil. (a) The magnetic manipulator and a 3D model of a collinear dual-coil EM setup [Citation14]. (b) Setting up an experimental MRI scanner using an acrylic box and avascular phantom (Siemens, Erlangen, Germany) and the magnetic gradient coils set configuration on Magnetom Verio is represented. For the x and y-axes, there are two sets of saddle coils, and for the z-axis, there are two sets of Maxwell coils [Citation15].
![Figure 4. Magnetic actuation systems that rely on a pair coil. (a) The magnetic manipulator and a 3D model of a collinear dual-coil EM setup [Citation14]. (b) Setting up an experimental MRI scanner using an acrylic box and avascular phantom (Siemens, Erlangen, Germany) and the magnetic gradient coils set configuration on Magnetom Verio is represented. For the x and y-axes, there are two sets of saddle coils, and for the z-axis, there are two sets of Maxwell coils [Citation15].](/cms/asset/6f25aa9f-b220-4fbf-bb69-be2083be8364/imit_a_2198004_f0004_c.jpg)
Because of its effectiveness in medical imaging, the MRI scanner is widely employed for diagnosis in hospitals. It produces a dynamic 3D gradient field and a powerful static horizontal magnetic field. MRI scanners could be utilised with one scanner for simultaneous actuation, localisation, and imaging of systems [Citation15]. However, MRI technology has unavoidable technical limits and physiological disturbances, such as pulsatile flow limitations, overheating, and susceptibility artefacts. With this limitation, a Pareto-optimal navigation planning framework is used to implement a 2D autonomous navigation approach for an MRI scanner with an intricate vascular network [Citation37]. Real-time propulsion, control, and imaging investigations are carried out in an interventional medical platform () utilising a specialised control architecture that uses these optimal conditions. The experiment demonstrated that devices could be guided using an MRI scanner and were viable with only a software update. The magnetic device navigated complex routes at approximately 4 millimetres per second, consistent with clinical MRI scanners. MRI scanners could be utilised with one scanner for simultaneous actuation, localisation, and imaging systems.
Distributed electromagnets
Distributed electromagnets have been created to increase energy efficiency and reduce layout constraints. Electromagnet-based distributed systems contain columnar coils that move around and point to the workspace. A soft-iron core can be inserted as it is readily magnetised and demagnetised. Many magnetic manipulation technologies have been created to control components in vast work areas. The number of electromagnets, the arrangement, and the limits of these systems all vary. Systematic analysis of issues in various situations was presented, and the necessary number of stationary electromagnets was rigorously quantified with the given magnetic manipulation requirement [Citation38]. Maxwell’s equations have been analysed in terms of their influence. It is indicated that 3-DOF force control requires four magnetic sources, whereas 5-DOF and heading control require eight magnetic sources. OctoMag () is a 5-DOF wireless manipulation device that utilises a distributed array arrangement [Citation16]. It contains two sets of eight identical electromagnets with no limitations on the ability to move across an expansive workspace or in its degrees of rotation flexibility. Instead of using uniform fields and linear superposition, which make modelling and control more accessible, OctoMag uses complicated non-uniform magnetic fields, giving it a unique set of capabilities. With computer vision, the system provides precise positioning. Using the 5-DOF range of motion enabled by this approach, controlled magnetic forces and torques can also be delivered. On the other hand, there is their inability to deliver great forces and their incapacity to carry massive weights. This control system may not be the best solution for performing surgical operations requiring much force. After that, OctoMag was modified as MiniMag () to be as small as possible [Citation17]. The two sets’ optimum deflections are lower than OctoMag’s. There are systems where the upper and lower sets are so perfectly aligned that the misalignment is of zero magnitudes.
Figure 5. Magnetic actuation systems rely on a distributed electromagnet. (a) OctoMag’s working prototype. The system employs electromagnets with lengths and diameters of 210 mm apiece. The distance [Citation38]. (b) Views from the top and bottom of the MiniMag and electromagnetic core configuration [Citation16]. (c) A prototype of magnetic catheter tip positioning and electromagnet system [Citation17]. (d) BigMag is six mobile coils in a spherical workspace. It also includes an automated inserter that regulates stereo vision. The coils are placed on two fixtures that spin [Citation18]. (e) The ARMM system features a mobile electromagnet s’with 6-DOF and can offer magnetic actuation in a sphere of a workspace [Citation19]. (f) Conceütual system illustration concerning the patient and an overall magnetic actuation system construction. It utilises a parallel mechanism to power three electromagnets [Citation39]. (g) Overview of the experimental validation setup [Citation21].
![Figure 5. Magnetic actuation systems rely on a distributed electromagnet. (a) OctoMag’s working prototype. The system employs electromagnets with lengths and diameters of 210 mm apiece. The distance [Citation38]. (b) Views from the top and bottom of the MiniMag and electromagnetic core configuration [Citation16]. (c) A prototype of magnetic catheter tip positioning and electromagnet system [Citation17]. (d) BigMag is six mobile coils in a spherical workspace. It also includes an automated inserter that regulates stereo vision. The coils are placed on two fixtures that spin [Citation18]. (e) The ARMM system features a mobile electromagnet s’with 6-DOF and can offer magnetic actuation in a sphere of a workspace [Citation19]. (f) Conceütual system illustration concerning the patient and an overall magnetic actuation system construction. It utilises a parallel mechanism to power three electromagnets [Citation39]. (g) Overview of the experimental validation setup [Citation21].](/cms/asset/83bc5073-b21e-44bb-8a4a-110052cd0301/imit_a_2198004_f0005_c.jpg)
Moreover, an automated approach using eight electromagnets arranged in three dimensions () concerning one for inserting and directing an intravascular three-magnet tip catheter in the human heart that is user-friendly has been designed and tested [Citation18]. When using the presented electromagnet system, the catheter can be bent between −35.41° and 17.30° from the initial to the final. BigMag (), an adjustable magnetic actuation system comprised of six electromagnets placed in two fixtures, was developed to address the challenges of catheter actuation, tracking, and closed-loop control alternative to catheter systems that utilise stationary electromagnets [Citation19]. Several experiments were carried out to determine how effectively the framework performs. In the magnetic field, the inverse map shows a 2.20% error.
The ARMM (advanced robotics for magnetic manipulation) system was introduced [Citation20]. This system offers ROI for specified mass and heat dissipation limitations (). The ARMM system can create magnetic fields and gradients precisely required while allowing for non-linearities induced by core saturation, owing to the approach. However, there are particular challenges in the length of catheterisations, the accuracy when the catheter is inserted, and the dependability with which these catheters are imaged using non-hazardous methods. Because of that, an ARMM system is being implemented to address these concerns, which combines different subsystems to improve clinical practicability [Citation39]. A clinically suitable workstation and a gelatinous phantom depicting a life-sized human torso are utilised in the experiments. An ultrasound image guides catheter insertion utilising a magnet with a median operation time to the target of 32.6 s.
The ARMM system offers the advantage of broad workspaces that produce lower heat, switch on and off the magnetic field, and provide adequate area for imaging equipment. Aside from that, positioning errors caused by angular displacements in the pulsating fluid flow should be minimised. Moreover, in response to long duration and undesired trauma limits, a workflow for magnetic control of a steerable guidewire and catheter under ultrasound (US) guidance () has been developed [Citation21]. The presented model accurately predicts tip deformation and the average operating speed is 3.08 mm/s, with an average modelling error of 0.89 mm and a 1.79 mm average error between monitored tip locations and target points
There are benefits and drawbacks to both mobile and permanent. Mobile permanent magnetic systems offer the benefit of adjusting workspace size, while stationary electromagnets have poor environmental adaptability, high energy consumption, and are challenging to scale. Despite this, there are certain disadvantages to being aware. Stationary electromagnets need a simple current control strategy and complete field generation capability, while mobile permanent magnetic systems offer limited field generation dexterity and complex motion control.
The therapeutic use of these magnetic devices depends on the efficiency of predicting and simulating their behaviour in complicated anatomical structures. A new simulation framework that accounts for magnetic actuation and interaction forces with meshed collision models was introduced [Citation22,Citation40]. Using characterisation and autonomous and providing training simulators were also demonstrated using this system. Its physical equivalent, the real-world m-CR, exhibited similar behaviour to the experiment. The average errors positioning and angle vary from 5.1 to 6.8 mm and 3.8 to 7.2 degrees, respectively.
Challenges and future works
Magnetic propulsion, control and tracking
Despite the many benefits of mobile electromagnetic systems, significant difficulties remain. Electromagnets have a considerably great volume and weight, and in certain situations, a multi-coil configuration with strong interaction forces is needed. Consequently, load-carrying, extremely durable, and structurally compact drive systems are required. Numerous solutions and substantial non-linearities could arise due to the whole DOF of both driven mechanisms and coil currents; thus, further study into developing field-generating algorithms with high robustness is highly required.
As imaging remains a concern in many applications, imaging instruments should be included at the system level. Magnetic localisation is ideally suited to millimetre-sized end effectors, while micrometre end effectors would struggle. Individuals with cardiac pacemakers or metallic foreign bodies can use MRI for simultaneous actuation, localisation, or imaging. Due to the apparent limited area, radiation is an inevitable consequence of fluoroscopy equipment. Probe rotation and translation are required for ultrasound imaging, which must be handled when magnetic actuation devices are used.
Motion capture sensors are extensively used in the monitoring of catheters [Citation23,Citation41]. However, their clinical use is restricted due to catheter integration issues and interference from ferromagnetic materials in operating rooms. The limited visibility and quality of the images and the breathing movements restrict image-based catheter tracking. It is essential to monitor the whole catheter to prevent physical harm to the tissues, not just the tip. Furthermore, the future development of robotic catheterisation platforms could offer possibilities to automate difficult-to-perform procedures, lowering the cognitive load on the operators.
Manoeuvrability and performance
Catheters’ ability to do complex tasks is determined by several factors, including their output force, movement accuracy, DOF, and working space. The catheter can do complex tasks if the output force is strong and precise, and there are enough DOFs and workspace available. However, there is a time delay between catheter movement and magnetic field vector identification when permanent magnets generate a magnetic navigation system that cannot be extended beyond a certain point. Furthermore, due to magnetic fields interfering with one another, the magnetic navigation catheter system does not enable using more than one catheter at a time.
Safety concerns
Modern robotic systems utilise a joystick or haptic device as the master, altering experienced operators’ natural behaviour and motion patterns. Consequently, researchers are trying to create robotic systems with master interfaces that imitate the motions of a clinician at the bedside. Future research should create ergonomically sound, hands-on robotic platforms that preserve the operator’s inherent competency, significantly affecting the widespread adoption of robotic catheterisation devices. Using force feedback to improve patient safety and catheter navigation will decrease radiation exposure while enhancing catheter navigation.
Low cost
The costs of medical devices are a significant concern for both clinicians and patients. The magnetic navigation catheter and magnetic actuation system is the most expensive device, and the pricing includes both the robotic system and the expenses of the operational environment. In order to avoid magnetic fields from interfering with other devices, installing a system will need the installation of steel plates and specialised equipment, which are both costly and time-consuming.
Low learning curve
A low learning curve benefits clinicians since it reduces training time and implementation expenses. Reduced surgery times benefit patients while freeing up clinicians’ time and saving money. Involving clinicians in developing design criteria and requirements for both function and operation will ensure that the final product meets the needs of both settings.
Conclusion
A remote actuation tool with a controlled magnetic field fulfils the locomotion needs of magnetic robots in restricted spaces. Because of this, the magnetic actuation system’s performance directly impacts clinical applications. A permanent magnet is used to create regional variations in the magnetic field, and various electromagnetic currents
This review describes actuation and navigation catheter systems, covering the fundamental theory, systematic setup, and potential challenges. The preliminary results have shown that magnetic actuation methods still require additional research and validation. Magnetic actuation systems will enable significant improvements when combined with other cutting-edge biomedical innovation technologies such as advanced control methods.
Disclosure statement
No potential conflict of interest was reported by the author(s).
Additional information
Funding
References
- Midthun DE. Early detection of lung cancer. F1000Res. 2016;5:739.
- Sutedja G. New techniques for early detection of lung cancer. Eur Respir J Suppl. 2003;39(39 suppl):57s–66s.
- Wobith M, Wehle L, Haberzettl D, et al. Needle catheter jejunostomy in patients undergoing surgery for upper gastrointestinal and pancreato-biliary cancer–impact on nutritional and clinical outcome in the early and late postoperative period. Nutrients. 2020;12(9):2564.
- Naranjo J, Portner ER, Jakub JW, et al. Ipsilateral intravenous catheter placement in breast cancer surgery patients. Anesth Analg. 1213;2021:10.
- Assadsangabi B, Tee MH, Wu S, et al. Catheter-based microrotary motor enabled by ferrofluid for microendoscope applications. J Microelectromech Syst. 2016;25(3):542–548.
- Xie F, Zheng X, Xiao B, et al. Navigation bronchoscopy-guided radiofrequency ablation for nonsurgical peripheral pulmonary tumors. Respiration. 2017;94(3):293–298.
- Heidt T, Reiss S, Krafft AJ, et al. Real-time magnetic resonance imaging–guided coronary intervention in a porcine model. Sci Rep. 2019;9(1):10.
- Liu T, Poirot NL, Franson D, et al. Modeling and validation of the three-dimensional deflection of an MRI-compatible magnetically actuated steerable catheter. IEEE Trans Biomed Eng. 2015;63:2142–2154.
- da Veiga T, Chandler JH, Lloyd P, et al. Challenges of continuum robots in clinical context: a review. Prog Biomed Eng. 2020;2(3):032003.
- Rahmer J, Stehning C, Gleich B. Remote magnetic actuation using a clinical scale system. PLoS One. 2018;13(3):e0193546.
- Leong F, Garbin N, Di Natali C, et al. Magnetic surgical instruments for robotic abdominal surgery. IEEE Rev Biomed Eng. 2016;9:66–78.
- Simi M, Silvestri M, Cavallotti C, et al. Magnetically activated stereoscopic vision system for laparoendoscopic single-site surgery. IEEE/ASME Trans Mechatron. 2013;18(3):1140–1151.
- Ryan P, Diller E. Magnetic actuation for full dexterity microrobotic control using rotating permanent magnets. IEEE Trans Robot. 2017;33(6):1398–1409.
- Zhang W, Meng Y, Huang P. A novel method of arraying permanent magnets circumferentially to generate a rotation magnetic field. IEEE Trans Magn. 2008;44:2367–2372.
- Erin O, Boyvat M, Tiryaki ME, et al. Magnetic resonance imaging system–driven medical robotics. Adv Intell Syst. 2020;2(2):1900110.
- Kummer MP, Abbott JJ, Kratochvil BE, et al. OctoMag: an electromagnetic system for 5-DOF wireless micromanipulation. IEEE Trans Robot. 2010;26(6):1006–1017.
- Kratochvil BE, Kummer MP, Erni S, et al. editors. MiniMag: a hemispherical electromagnetic system for 5-DOF wireless micromanipulation. Experimental robotics; 2014. Springer.
- Le VN, Nguyen NH, Alameh K, et al. Accurate modeling and positioning of a magnetically controlled catheter tip. Med Phys. 2016;43(2):650–663.
- Sikorski J, Denasi A, Bucchi G, et al. Vision-based 3-D control of magnetically actuated catheter using BigMag – an array of mobile electromagnetic coils. IEEE/ASME Trans Mechatron. 2019;24(2):505–516.
- Sikorski J, Heunis CM, Franco F, et al. The ARMM system: an optimized mobile electromagnetic coil for non-linear actuation of flexible surgical instruments. IEEE Trans Magn. 2019;55(9):1–9.
- Yang Z, Yang L, Zhang M, et al. Magnetic control of a steerable guidewire under ultrasound guidance using mobile electromagnets. IEEE Robot Autom Lett. 2021;6(2):1280–1287.
- Yang Z, Zhang L. Magnetic actuation systems for miniature robots: a review. Adv Intell Syst. 2020;2(9):2000082.
- Tercero C, Ikeda S, Uchiyama T. eds. Catheter insertion mechanism and feedback control using magnetic motion capture sensor. 2006 SICE-ICASE International Joint Conference; 2006. IEEE.
- Armacost MP, Adair J, Munger T, et al. Accurate and reproducible target navigation with the stereotaxis niobe® magnetic navigation system. J Cardiovasc Electrophysiol. 2007;18: s26–S31.
- Filgueiras-Rama D, Estrada A, Shachar J, et al. Remote magnetic navigation for accurate, real-time catheter positioning and ablation in cardiac electrophysiology procedures. J Vis Exp. 2013;74:3658.
- Carpi F, Pappone C. Stereotaxis niobe® magnetic navigation system for endocardial catheter ablation and gastrointestinal capsule endoscopy. Expert Rev Med Devices. 2009;6(5):487–498.
- Tognarelli S, Castelli V, Ciuti G, et al. Magnetic propulsion and ultrasound tracking of endovascular devices. J Robot Surg. 2012;6(1):5–12.
- Zhang S, Yin M, Lai Z, et al. Design and characteristics of 3D magnetically steerable guidewire system for minimally invasive surgery. IEEE Robot Autom Lett. 2022;7(2):4040–4046.
- Steck D, Qu J, Kordmahale SB, et al. Mechanical responses of ecoflex silicone rubber: compressible and incompressible behaviors. J Appl Polym Sci. 2019;136(5):47025.
- Pittiglio G, Barducci L, Martin JW, et al. Magnetic levitation for soft-tethered capsule colonoscopy actuated with a single permanent magnet: a dynamic control approach. IEEE Robot Autom Lett. 2019;4(2):1224–1231.
- Mahoney AW, Abbott JJ. Managing magnetic force applied to a magnetic device by a rotating dipole field. Appl Phys Lett. 2011;99(13):134103.
- Slawinski PR, Simaan N, Taddese AZ, et al. Sensitivity ellipsoids for force control of magnetic robots with localization uncertainty. IEEE Trans Robot. 2019;35(5):1123–1135.
- Mahoney AW, Abbott JJ. Generating rotating magnetic fields with a single permanent magnet for propulsion of untethered magnetic devices in a lumen. IEEE Trans Robot. 2014;30(2):411–420.
- Di Natali C, Buzzi J, Garbin N, et al. Closed-loop control of local magnetic actuation for robotic surgical instruments. IEEE Trans Robot. 2015;31(1):143–156.
- Scaglioni B, Fornarelli N, Garbin N, et al. Independent control of multiple degrees of freedom local magnetic actuators with magnetic cross-coupling compensation. IEEE Robot Autom Lett. 2018;3(4):3622–3629.
- Leclerc J, Isichei B, Becker AT. A magnetic manipulator cooled with liquid nitrogen. IEEE Robot Autom Lett. 2018;3(4):4367–4374.
- Folio D, Ferreira A. Two-dimensional robust magnetic resonance navigation of a ferromagnetic microrobot using pareto optimality. IEEE Trans Robot. 2017;33(3):583–593.
- Petruska AJ, Nelson BJ. Minimum bounds on the number of electromagnets required for remote magnetic manipulation. IEEE Trans Robot. 2015;31(3):714–722.
- Heunis CM, Wotte YP, Sikorski J, et al. The armm system-autonomous steering of magnetically-actuated catheters: towards endovascular applications. IEEE Robot Autom Lett. 2020;5(2):705–712.
- Dreyfus R, Boehler Q, Nelson BJ. A simulation framework for magnetic continuum robots. IEEE Robot Autom Lett. 2022;7(3):8370–8376.
- Tercero C, Ikeda S, Uchiyama T, et al. Autonomous catheter insertion system using magnetic motion capture sensor for endovascular surgery. Int J Med Robot. 2007;3:52–58.