Abstract
Context
Kuhuang (KH) injection is a widely used anticholestatic drug in the clinic and the mechanisms are still unclear.
Objective
This study uses a new 3D tissue-engineered (TE) liver platform to study the ability of kuhuang to ameliorate liver injury induced by chlorpromazine (CPZ) and the possible mechanisms involved.
Materials and methods
The TE livers (n = 25) were divided into 5 groups (n = 5 livers/group) as 3D, 3D + CPZ, 3D + CPZ + KH, 3D + CPZ + GW9662 (a PPARγ inhibitor) and 3D + CPZ + KH + GW9662. The treatments with kuhuang (1 mg/mL) and GW9662 (10 μmol/L) were given to the desired groups on the 7th day of the experimental process. 20 μmol/L CPZ was added on the 8th day.
Results
According to the 2D experimental results, the minimum effective concentration of kuhuang is 10 μg/mL and the optimal effective concentration is 1 mg/mL. Kuhuang ameliorated tissue damage in the TE livers both in terms of tissue structure and culture supernatant. Kuhuang significantly reduced TBA accumulation (38%) and downregulated CYP7A1 (38%) and CYP8B1 (79%). It reduced hepatic levels of ROS (14%), MDA (27%) but increased the levels of GSH (41%), SOD (12%), BSEP (4.4-fold), and MRP2 (74%). Moreover, kuhuang downregulated DR5 (99%) but increased the mRNA expression of PPARγ (4-fold). Molecular docking analyses determined the bioactivity of the active compounds of kuhuang through their specific bindings to PPARγ.
Conclusions
Kuhuang could alleviate CPZ-induced cholestatic liver injury by activating PPARγ to reduce oxidative stress. Applying kuhuang for the treatment of CPZ-induced liver injury could be suggested.
Introduction
Drug-induced liver injury (DILI) is a common clinical adverse drug reaction and an important factor in drug development failure and withdrawal (Kullak-Ublick et al. Citation2017). Cholestatic injury and mixed hepatocytic/cholestatic injury constitute the two main subtypes of DILI and may account for 50% of all DILI cases (Shen et al. Citation2019). Drug-induced cholestasis (DRIC) may manifest clinically as pruritus, malaise, darkened urine, and jaundice and is often asymptomatic in the early stages, eventually manifesting as elevated serum alkaline phosphatase (ALP) and γ-glutamyl transpeptidase (GGT) levels and possibly progressing to hyperbilirubinemia, which can lead to liver failure or even death in severe cases (Padda et al. Citation2011). At present, effective drugs for the clinical treatment of cholestasis are lacking. Ursodeoxycholic acid (UDCA) and obeticholic acid (OCA) are the clinically approved drugs for cholestasis and are recognized to have certain curative effects, but their therapeutic effect is limited, as there are large individual differences in their effects (Santiago et al. Citation2018). Thus, the treatment strategies for DRIC are not ideal. Therefore, it is necessary to develop new drugs for the treatment of cholestatic liver injury. Furthermore, traditional Chinese medicine has received increasing attention in the treatment of DRIC (Li et al. Citation2019; Wang et al. Citation2020; Hua et al. Citation2021).
Kuhuang injection is a traditional Chinese medicine injection made from traditional Chinese medicinal materials such including Radix Bupleuri, Sophora Flavescens, Rheum Officinale, Herba Artemisiae, and Folium Isatidis (shown in ), which are extracted and refined through modern technologies (Wu et al. Citation2005a, Citation2005b). It has the effects of clearing heat, removing dampness, soothing the liver, and relieving jaundice. It is commonly used clinically to relieve jaundice and protect the liver. Clinical reports have revealed that it protects the liver and ameliorates intrahepatic cholestasis, but the specific mechanism by which it alleviates jaundice is still unclear (Xu et al. Citation2009).
Table 1. Raw material information of kuhuang injection.
The mechanisms of DRIC are diverse, and some of the related factors are unknown (Sundaram and Björnsson Citation2017; Chatterjee and Annaert Citation2018). Changes in the expression of liver bile transport proteins (BSEP and MRP2) in addition to other mechanisms, such as damage to the cytoskeleton and oxidative stress, contribute to the pathological process of cholestasis (Anthérieu et al. Citation2013; Hendriks et al. Citation2016; Burban et al. Citation2018). CPZ is a neuroleptic phenothiazine. It is widely used in the clinical treatment of schizophrenia and has been associated with several cases of hepatic injury, mainly intrahepatic cholestasis (Todorović Vukotić et al. Citation2021). However, its initial toxic effects are still largely ignored, possibly because the current models used for safety assessment in drug development cannot accurately predict human cholestasis. Therefore, it is necessary to construct a new preclinical cellular model of DRIC. Because the source of fresh human hepatocytes is limited, we used differentiated human HepG2 cells expressing phase I and phase II drug-metabolizing enzymes and transporters (Hewitt and Hewitt Citation2004; Ramaiahgari et al. Citation2014) and established a novel three-dimensional (3D) TE liver platform in vitro by recellularization of a naturally obtained rat decellularized scaffold (Wu et al. Citation2018; Han et al. Citation2019; Zhang et al. Citation2021) in this study. By using this platform, we constructed a DRIC model by adding CPZ (20 μmol/L) and bile acid mixtures (shown in ) to analyse the characteristics of intrahepatic cholestasis induced by CPZ treatment and to explore the protective effect of kuhuang injection against CPZ-induced cholestasis.
Table 2. Composition of the bile acid mixture and the concentrations.
Materials and methods
DMEM was purchased from Thermo Fisher Biochemicals Co., Ltd. (USA). Foetal bovine serum, PLA2 enzyme, and penicillin were purchased from Gibco (USA). An AMV one-step RT-PCR kit was obtained from TaKaRa (China). Kuhuang injection extract powder was provided by Changshu Leiyunshang Pharmaceutical Co., Ltd. (China). A peristaltic pump was purchased from Master-Flex (German), and a 0.22 μm filter (Millex-GV) (German) was used. Sodium Deoxycholate (SDC) was obtained from VETEC (USA), and disposable indwelling intravenous needles (BD type Y 22 G) (USA) were used. A cell counting kit-8 (CCK-8) kit was purchased from Dojindo (USA), and reactive oxygen species (ROS) detection kits were purchased from Shanghai Biyuntian (China). Malondialdehyde (MDA) assay kits, superoxide dismutase (SOD) assay kits, glutathione (GSH) assay kits, and TBA assay kits were purchased from Nanjing Jiancheng Institute of Bioengineering (China). Aspartate transaminase (AST) test kits, alanine transaminase (ALT) test kits, lactate dehydrogenase (LDH) test kits, and alkaline phosphatase (ALP) test kits were all obtained from Nanjing Jiancheng Institute of Biological Engineering (China). CPZ was purchased from MCE (MedChemExpress) (USA), and GW9662 was obtained from Selleck (USA).
Animals
Male Sprague-Dawley (SD) rats (body weight: 180–220 g) were obtained from the Weitong Lihua Experimental Animal Centre. The animals were bred normally before the experiment. The Capital Medical University Animal Experiments and Experimental Animals Management Committee (AEEI-2020-076) approved all protocols for feeding, anaesthesia, blood and tissue sampling, and euthanasia of animals.
Preparation of decellularized liver scaffolds
Rats were fasted for 24 h and deprived of water for 4 h before the procedure. The rats were anaesthetized with 10% chloral hydrate. After disinfection, the abdominal cavity was opened to expose the liver and portal vein, and an indwelling intravenous needle was inserted through the portal vein. After fixation, a peristaltic pump was turned on at a flow rate of 15–20 mL/min and used to flush the portal vein for approximately 20 min. Then 2% PLA2 enzyme was applied for 10 min followed by precooled HBSS for 20 min, as shown in . Then, the entire liver was removed, ensuring the integrity of the liver. Subsequently, rat liver scaffolds were ligated and trimmed, and the middle liver lobe was retained. The scaffolds were placed in a special-shaped sterile bottle prefilled with DMEM, connected to a peristaltic pump device, and circulated overnight in an incubator at 37 °C and 5% CO2.
Figure 1. Constructing in vitro model of chlorpromazine-induced cholestatic liver injury by tissue engineering. (A) Schematic diagram of the model establishment process. (B) The process of obtaining decellularized scaffolds. (C) Appearance of normal rat liver (C1) and decellularized rat liver scaffold (C2). D. HE staining of normal rat liver (D1) and decellularized rat liver scaffold (D2). E. Bioreactor system for growing TE livers. (F) Appearance of the established TE liver (F1) and liver injury model (F2).
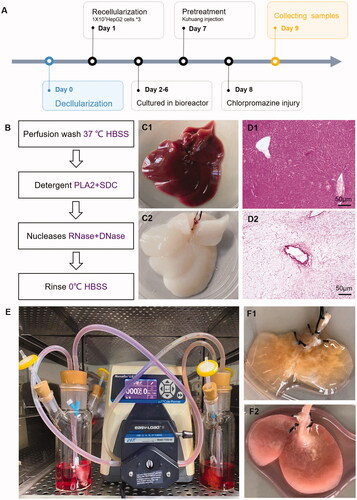
Establishment of CPZ-induced cholestatic TE liver injury
Highly differentiated HepG2 cells were cultured in DMEM supplemented with 10% foetal bovine serum, 100 U/mL penicillin, and 0.1 mg/mL streptomycin. Decellularized scaffolds were recellularized 3 times with 1 × 107 HepG2 cells each time at a rate of 9 mL/min. The perfusion rate was selected based on the hepatic blood flow rate of adult rats during the resting period (85 mL/min/kg). After completion of recellularization, the medium with bile acid mixtures (shown in ) was changed every day for 5 consecutive days. The livers grew after 5 days of culture, and then kuhuang (1 mg/mL) was added on the 7th day of the experimental procedure. Twenty-four h later, on the 8th day, CPZ was added to establish injury, as shown in . The livers were collected 24 h after CPZ was added, and supernatant samples were collected before and after CPZ administration and examined.
Analysis of cytotoxicity by the CCK-8 assay
HepG2 cells were suspended at a density of 40–60 cells/µL, and 100 µL of the cell suspension was added to each well of a 96-well plate. The cells were incubated for 24 h, and then kuhuang was added to the culture plate at different concentrations. After 24 h, 10 µL of CCK-8 solution was added to each well according to the manufacturer’s instructions, the culture was incubated for 20 min, the absorbance (optical density [OD]) was measured at 450 nm with a microplate reader, and cell viability was calculated.
Analysis of ROS, MDA, GSH, and SOD levels
Cells from TE livers were ground, collected in centrifuge tubes and washed 1–2 times with PBS. The precipitated cells were collected by low-speed centrifugation, and then the cells were fully lysed using lysis solution. The supernatants were collected, 500 µL of PBS and 0.5 µL of the fluorescent probe DCFH-DA was added, the supernatants were incubated in a water bath at 37 °C for 40 min and washed twice with PBS, 200 µL PBS was added, the samples were mixed, and a fluorescence microplate reader was used to measure the fluorescence intensity at 480 nm excitation and 525 nm emission. ROS levels in the cells were calculated.
The lysis supernatants were placed in a 96-well plate and reagents and samples were added according to the instructions of the MDA kit. Then, the absorbance of each well was measured at 532 nm using an enzyme marker. Finally, the level of MDA in the cells was calculated.
The supernatants were processed according to the instructions of the GSH kit. The samples were added to a 96-well plate, mixed well, and allowed to stand for 5 min. Then, the absorbance of each well was measured with a microplate reader at 405 nm to calculate the GSH content in the cells.
The samples were added to a 96-well plate according to the instructions of the SOD kit. After mixing and incubating at 37 °C for 20 min, the absorbance was measured with a microplate reader at a wavelength of 450 nm. The concentrations of SOD in the cells were calculated.
Analysis of AST, ALT, LDH, and ALP levels
Supernatants were collected in a special-shaped bottle after the livers were removed from the machine. The samples were added to a 96-well plate and processed according to the instructions of the corresponding kit. The optical density (OD) of each sample was measured using a microplate reader at the appropriate wavelength, and the enzyme levels in the samples were calculated.
TBA assay
The TE livers were removed, and C4 collagenase was used to isolate HepG2 cells from the livers. The cells were placed in a 15 mL centrifuge tube, centrifuged at 1000 rpm, and washed 2–3 times with PBS, and the pelleted cells were collected. Then, 150 µL of RIPA lysis buffer was added, and the cells were ultrasonicated to achieve full lysis. According to the instructions of the kit, the samples were placed on 96-well plates. After incubation for 5 min in a 37 °C incubator, the OD was measured with a microplate reader at a wavelength of 405 nm, the plates were incubated in a 37 °C incubator for 2 min, and the OD was measured again with a microplate reader. Finally, according to the appropriate formula and dilution factor, the concentration of TBA in each group of samples was calculated.
SEM and TEM
The obtained TE livers were fixed for SEM and TEM using 2.5% glutaraldehyde. The samples were then sent to the Electron Microscopy Centre of Peking University Medical School for sample processing. After processing, the samples were observed with a JSM-5600LV scanning electron microscope (JEOL) and a JEM1230PLUS transmission electron microscope (JEOL) at 80 kV. Digital images were acquired using a Gatan Orius SC1000 CCD digital camera with Digital MicroImager 3.11.0 software (Gatan).
Immunofluorescence analysis of tissue sections
Paraffin sections were dewaxed and hydrated. Antigen repair solution was boiled, and then the sections were placed in the solution, boiled for 10 min, removed, cooled naturally to room temperature, and washed three times with ddH2O. Primary antibodies were diluted with the serum of the secondary antibody host species, and the sections were incubated with primary antibody overnight at 4 °C and washed three times with TBS. The secondary antibody was diluted with the serum of the secondary antibody host species, and the sections were incubated with secondary antibody for 1 h at room temperature and washed three times with TBS. The nuclei were stained with DAPI at room temperature for 10 min. Finally, the slides were sealed, and images were collected using a laser confocal microscope.
RNA extraction and RT-qPCR
Total RNA was extracted with TRIzol and analysed with a UV spectrophotometer. The RNA concentration was calculated, and then the RNA was treated with DNase. After reverse transcription using a kit from Takara, qPCR was performed on an ABI StepOnePlus machine. The primers used are shown in .
Table 3. Primers used in this study.
Western blot analysis
Samples were collected, total protein was extracted, and the protein concentration was determined by the BCA method. A total of 34 g protein per sample was subjected to 30% acrylamide-methylene bisacrylamide gel electrophoresis and transferred via the wet transfer method. After transfer, Lichun red was used to stain the membrane, and protein transfer was assessed. The membranes were incubated with monoclonal antibodies diluted in 5% BSA-TBST overnight at 4 °C. The next day, the membranes were washed, incubated with secondary antibody, shaken for 40 min at room temperature, and washed 3 times with TBST, developed with a luminescent solution, and imaged.
Identification and analysis of active compounds for docking validation
According to the kuhuang drug certificate of analysis report provided by Lei Yunshang Company and the existing literature report (Wu et al. Citation2005a), we selected these four monomers (matrine, emodin, sophocarpine and sophoridine) with specific contents as active compounds. The two-dimensional (2D) structures of the compounds were downloaded from the PubChem database as SDF files, optimized with Chem3D software, and saved in × mol2 format. The 3D structures of the target proteins were downloaded from the PDB database (https://www.rcsb.org/), dehydrated and hydrogenated using PyMOL software, and then extracted with AutoDock Vina 1.1.2 software. The 3D structure of the target protein was downloaded from the PDB database, dehydrated and hydrogenated using PyMOL software, hydrogenated with AutoDock Vina 1.1.2 software, charged and combined with nonpolar hydrogen, and saved in × pdbqt format. The active site of the target was determined from the literature, grid box coordinates were set, and the box size was adjusted to 0.1 nm for each small box. Small molecules were docked to the proteins using AutoDock Vina 1.1.2 software.
Statistical methods
Statistical analysis was performed using GraphPad Prism 5.0 (GraphPad Software Inc., San Diego, CA, USA). The measurement data from the experiments are expressed as the mean ± SEM. SPSS 22 statistical software was used, and one-way ANOVA was used for comparisons between groups and LSD t-test was further used for statistical analyses between two independent groups. p* < 0.05, p** < 0.01, and p*** < 0.001 indicate significant differences.
Results
Establishment of a DRIC model
After recellularization, TE livers were continuously perfused with a peristaltic pump in an incubator (). Normal control TE livers were cultured in a complete medium. Livers in the 20 μmol/L CPZ-treated group and the 3D group after 7 days in culture are shown in and . After CPZ treatment, intracellular TBA levels in the CPZ group were 18.90 ± 0.65 μmol/L, which was a striking increase compared with those of control group (5.67 ± 1.13 μmol/L) (p < 0.01, ).
Figure 2. Effect of kuhuang on total bile acids (TBA) and metabolism rate-limiting enzymes in chlorpromazine-induced cholestatic TE liver. (A) The TBA concentrations of 3D, CPZ, and KH group. (B) RNA expression of CYP7A1. C. RNA expression of CYP8B1. D. WB analysis of 3D, CPZ, and KH group. *p < 0.05, **p < 0.01, ***p < 0.001, compared to the 3D CPZ group.
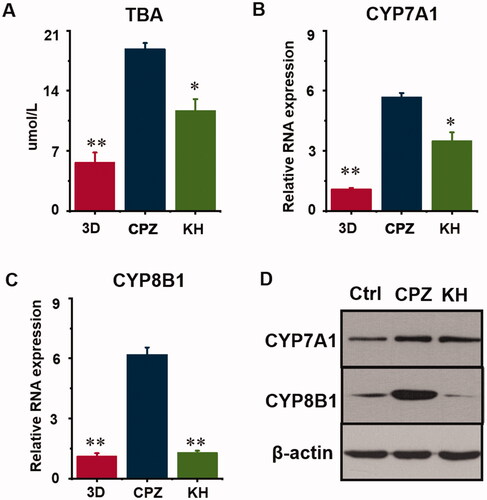
Kuhuang injection was essentially non-toxic and protected hepatocytes from CPZ-induced injury in 2D culture
The cytotoxicity and protective effects of kuhuang were tested in a 96-well plate. Kuhuang had no obvious cytotoxicity at different concentrations (from 1 ng/mL to 1 mg/mL), as shown in . The protective effect of different concentrations of kuhuang against CPZ-induced injury was further observed, and it was found that kuhuang had a dose-dependent protective effect starting at a dose of 10 µg/mL. Additionally, there was a positive correlation between cell viability with the kuhuang concentration, indicating that kuhuang has a good protective effect on cells, and 1 mg/mL was selected as the experimental drug concentration ().
Figure 3. Toxicity test and protective effect of kuhuang injection extract on chlorpromazine-induced HepG2 cell injury. (A) Different concentrations of kuhuang injection extract (1 ng/mL,10 ng/mL, 100 ng/mL, 1 μg/mL, 10 μg/mL, 100 μg/mL, and 1 mg/mL) on cell viability. (B) Protective effect of kuhuang injection extract on chlorpromazine-induced HepG2 cell injury. *p < 0.05, **p < 0.01, ***p < 0.001, compared to the 2D CPZ group.
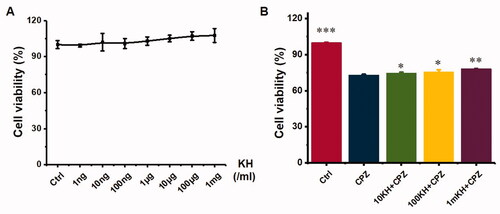
The protective effect of kuhuang on TE livers injured by CPZ
Administering CPZ to the TE livers caused significant (p < 0.05) liver damage and necrosis of cells as evidenced by the elevated serum hepatic enzymes ALT, AST, ALP, and LDH compared with the 3D group (). Effects of pre-treatment with kuhuang exhibited a significant (p < 0.05) decrease in serum activities of ALT, AST, ALP, and LDH (). HE staining showed that CPZ resulted in more intense staining of the nucleus and reduced staining of the cytoplasm. This indicates that the damage to the cells was significantly alleviated. Additionally, the number of cells was significantly increased in the KH group relative to the CPZ group (). SEM revealed that the morphology of the cells was normal, the connection between the cells was tight, the microvilli of the cells were developed, and swelling and rounding of the cells were improved in the KH group compared with the CPZ-treated group (). TEM showed that the capillary bile ducts between the cells were absent in the CPZ group but present after treatment with kuhuang. In addition, the injury group showed a significant accumulation of bile salt particles in liver cells (). The above data prove that kuhuang exerts a protective effect against drug-induced cholestatic liver injury in this model.
Figure 4. Culture supernatant levels of hepatocyte injury marker and effect of chlorpromazine and kuhuang on histological changes in TE liver tissue. (A) ALT; (B) AST; (C) LDH; (D) ALP; (E) H–E staining, SEM and TEM observation of the 3D, CPZ, and KH (Kuhuang + CPZ) group. Yellow arrow indicates capillary bile duct. Red arrow indicates aggregated bile acid salts.
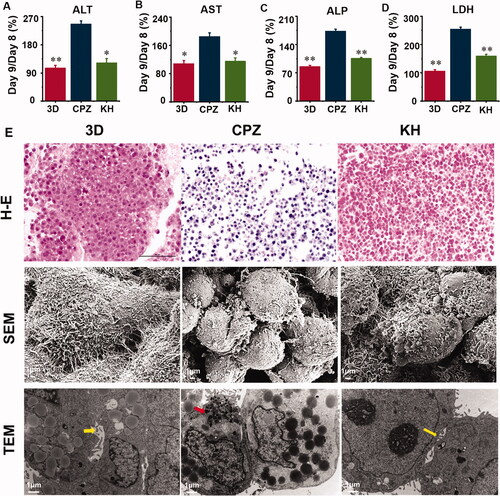
The effect of kuhuang on the metabolism of bile acids
At the end of culture, the TBA level in the CPZ group was 18.90 ± 0.65 μmol/L, and Kuhuang reduced this level to 11.72 ± 1.31 μmol/L (p < 0.05, ). The classic pathway of bile acid synthesis involves the action of CYP7A1, CYP8B1, and other enzymes. To clarify the molecular mechanisms by which Kuhuang regulates hepatic bile acids accumulation under CPZ treatment, CYP7A1 and CYP8B1 were measured by PCR and WB analysis (). The results showed that the expression of CYP7A1 and CYP8B1 increased significantly (p < 0.01) when CPZ was administered and that Kuhuang reduced the accumulation of bile acids in liver cells by inhibiting the expression of these two rate-limiting enzymes (CYP7A1, p < 0.05; CYP8B1, p < 0.01) compared to the CPZ group.
The effect of kuhuang on oxidative stress caused by CPZ
Significantly increased concentrations of ROS and MDA resulted from the addition of CPZ, indicating that CPZ induced oxidative stress in TE livers (p < 0.01, ). Moreover, CPZ decreased the SOD and GSH activity level by 22% and 29%, respectively (p < 0.05, ). In the KH group, CPZ-induced oxidative stress was significantly reduced (). Analysis of ROS and MDA levels showed that kuhuang effectively reduced the production of ROS and MDA in cells upon CPZ-induced damage, thereby protecting cells (p < 0.05, ). The GSH and SOD assay results showed that when cells were injured by CPZ, kuhuang effectively increased the content of GSH and SOD in the TE livers (p < 0.05, ), thereby increasing the antioxidant capacity of the cells. These results, which are shown in , indicate that kuhuang has a protective effect against the oxidative stress response.
The effect of kuhuang on bile acid export pumps
Under normal physiological conditions, bile acids produced in liver cells are mainly exported by BSEP and MRP2. F-actin is also expressed and helps hepatocytes export bile acids (). CPZ induces oxidative stress, which disrupts the integrity of the F-actin cytoskeleton, and structural damage to the F-actin cytoskeleton induces the expression of both BSEP and MRP2. The internalization and endocytosis of bile salt export pump further aggravate the accumulation of bile acids. It was found that the expression of the bile acid export pumps BSEP and MRP2 and F-actin was significantly higher in the kuhuang-treated group than in the CPZ-induced injury group (). This finding once again confirms that kuhuang exerts protective effects.
Kuhuang treatment inhibited CPZ-induced oxidative stress via PPAR-γ
According to the kuhuang drug certificate of analysis report and related literature (Wu et al. Citation2005a), 3 ingredients of Sophorae Flavescentis Radix (matrine, sophocarpine, and sophoridine) and 1 ingredient of Rhei Radix Et Rhizoma (emodin) were identified. Molecular docking between the 4 main components of kuhuang injection and the target PPARG (encodes the protein PPAR-γ) was then performed. The molecular docking binding energies of the 4 components to PPAR-γ are shown in . It is generally accepted that docking energy values less than −4.25 kcal/mol indicate some binding activity between the two molecules, docking energy values less than −5.0 kcal/mol indicate good binding activity, and docking energy values less than −7.0 kcal/mol indicate strong binding activity. The results showed that all 4 major components were able to bind to the target site of PPARG, and the binding sites are shown in .
Figure 7. Molecular docking model and visual map of binding site between main components of kuhuang injection and PPARG target. The main components of kuhuang injection are matrine, emodin, sophocarpine, and sophoridine.
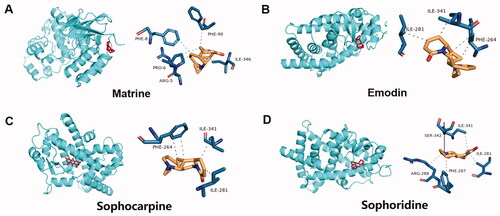
Table 4. The binding energy (BE) of the main components of kuhuang injection and the target molecule of PPARγ (Kcal/mol).
After CPZ-induced injury, oxidative stress resulted in the downregulation of PPAR-γ expression (p < 0.05, ) compared to the 3D group, and after kuhuang administration, the expression of PPAR-γ increased significantly (p < 0.01, ) compared to the CPZ group, indicating that kuhuang exerted a protective effect by inhibiting oxidative stress via an increase in PPAR-γ expression, ultimately leading to a decrease in the gene and protein expression of the death receptor DR5. The above results indicate that kuhuang exerts an inhibitory effect by increasing PPAR-γ expression and reducing DR5 expression (p < 0.01, ) compared to the CPZ group. Then, GW9662 (a PPAR-γ inhibitor) was used to confirm that kuhuang-induced inhibition of oxidative stress is dependent on PPAR-γ. Our results showed that kuhuang injection extract treatment did not further influence ROS overproduction following treatment with GW9662 in the presence of CPZ ().
Figure 8. Kuhuang could have an antioxidant effect and protect cell death via activating PPARγ. (A) RNA expression of PPARγ in different groups (3D, CPZ, KH). (B) WB analysis of PPARγ in different groups (3D, CPZ, KH). (C) RNA expression of DR5 in different groups (3D, CPZ, KH). (D) WB analysis of DR5 in different groups (3D, CPZ, KH). (E) WB analysis of PPARγ in different groups (3D, CPZ, KH, CPZ + GW9662, and KH + GW9662). GW9662 is the inhibitor of PPARγ. (F) ROS concentration in different groups (3D, CPZ, KH, CPZ + GW9662, and KH + GW9662).
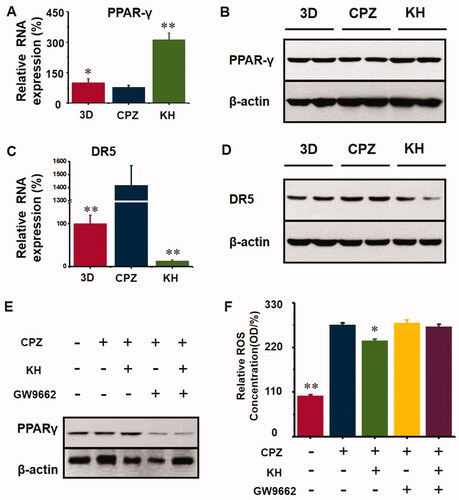
Discussion
DRIC is an important clinical problem that can lead to drug withdrawal and discontinuation, resulting in significant economic losses (Sundaram and Björnsson Citation2017). Due to differences in bile acid metabolism among species, animal models cannot simulate DRIC well (Petrov et al. Citation2018; McGill and Jaeschke Citation2019). However, the strategies for preclinically predicting a drug’s capacity to induce DRIC are currently limited to measuring the compound's potential to inhibit BSEP (Cheng et al. Citation2016; Gijbels et al. Citation2019). However, the manifestation of DRIC is often complex and multifactorial. Importantly, 3D interactions between cells and structures with noncellular components occur in the human body. However, classic 2D monolayer cell culture models cannot mimic these interactions between cells and components or matrices (Kuna et al. Citation2018). In the past decade, 3D cell culture systems, such as organoids and spheroids, have been developed to mimic the hepatic structure and cellular interactions in vitro (Sato et al. Citation2021). These 3D cell culture models cannot fully simulate the liver microstructure and circulatory perfusion system, which are very important for maintaining hepatocyte polarity and function. Therefore, new in vitro models that can be used to comprehensively assess the cholestatic risk of compounds in a physiologically relevant liver model are needed. We constructed a 3D TE liver model in the early stage, which can be used to better simulate drug metabolism by the liver and pathology (Wu et al. Citation2018; Han et al. Citation2019; Zhang et al. Citation2021). Because the model utilizes human hepatocytes, species variability is not an issue, and the model can be used to simulate DILI.
CPZ is a well-known cause of acute cholestatic liver injury (Todorović Vukotić et al. Citation2021). Numerous instances of apparent CPZ-induced acute liver injury, which is estimated to occur in 1 in 500 individuals exposed to CPZ, have been reported in the literature. Therefore, we used CPZ to injure TE livers and establish DRIC in this study. Increasing evidence shows that oxidative stress plays an important role in liver injury in rodents and humans with cholestatic liver disease (Anthérieu et al. Citation2013; Hendriks et al. Citation2016; Burban et al. Citation2018). In CPZ-induced cholestasis, oxidative stress can impede the secretion of bile acids by disrupting the cytoskeletal protein F-actin in hepatocytes and inducing internalization of BSEP and MRP2, causing intracellular accumulation of toxic bile acids, which induces DR5 expression and thus leads to activation of the death receptor signalling pathway. PPAR-γ is a ligand-inducible transcription factor of the nuclear hormone receptor superfamily (Ahmadian et al. Citation2013). It is expressed in hepatocytes and exerts antioxidant effects by inducing the expression of antioxidant enzymes through binding to elements in the promoters of antioxidant genes (Sheng et al. Citation2020; Xiang et al. Citation2021). Furthermore, docking software predicted that 14 main compounds of kuhuang injection had binding domains for PPAR-γ. In this study, we found that kuhuang prevented oxidative stress at least partially via the PPAR-γ signalling pathway. The PPAR-γ antagonist GW9662 was administered in combination with kuhuang injection extract, and the results showed that inhibition of PPAR-γ markedly alleviated the ability of Kuhuang injection to alleviate oxidative stress induced by CPZ, indicating that PPAR-γ is required for the effect of kuhuang and that PPAR-γ activation is a potential therapeutic strategy for cholestasis. A previous study revealed that thiazolidinediones, agonists of PPAR-γ, increase the expression of BSEP. Xiang et al found that tectorigenin, a partial agonist of PPAR-γ, alleviates cholestasis in an experimental mouse model (Xiang et al. Citation2021). These data indicate that activating PPAR-γ may be an effective target for the treatment of cholestatic liver disease. Additionally, we propose that Kuhuang can activate PPAR-γ, thus alleviating oxidative stress induced by CPZ, which can lead to disruption of F-actin and thus cause endocytosis of BSEP and MRP2, reducing the accumulation of toxic bile acids in cells, downregulating the expression of the apoptotic protein DR5 and protecting hepatocytes. This may also be the molecular mechanism by which kuhuang exerts its antioxidant and anti-injury effects in the cholestatic drug liver injury model.
Conclusions
This study shows that 1 mg/mL kuhuang injection extract exerts a significant anticholestatic effect, possibly by activating PPAR-γ signalling and reducing the internalization of MRP2 and BSEP caused by oxidative stress-induced disruption of F-actin, thereby alleviating CPZ-induced intrahepatic cholestasis and reducing the expression of DR5 and cell death (). These findings are expected to provide the basis for the clinical use of kuhuang injection in the treatment of drug-induced cholestatic liver injury.
Disclosure statement
No potential conflict of interest was reported by the author(s).
Additional information
Funding
References
- Ahmadian M, Suh JM, Hah N, Liddle C, Atkins A, Downes M, Evans R. 2013. PPARγ signaling and metabolism: the good, the bad and the future. Nat Med. 19(5):557–566.
- Anthérieu S, Bachour-El Azzi P, Dumont J, Abdel-Razzak Z, Guguen-Guillouzo C, Fromenty B, Robin MA, Guillouzo A. 2013. Oxidative stress plays a major role in chlorpromazine-induced cholestasis in human HepaRG cells. Hepatology. 57(4):1518–1529.
- Burban A, Sharanek A, Guguen-Guillouzo C, Guillouzo A. 2018. Endoplasmic reticulum stress precedes oxidative stress in antibiotic-induced cholestasis and cytotoxicity in human hepatocytes. Free Radic Biol Med. 115:166–178.
- Chatterjee S, Annaert P. 2018. Drug-induced cholestasis: mechanisms, models, and markers. Curr Drug Metab. 19(10):808–818.
- Cheng Y, Woolf TF, Gan J, He K. 2016. In vitro model systems to investigate bile salt export pump (BSEP) activity and drug interactions: a review. Chem Biol Interact. 255:23–30.
- Gijbels E, Vilas-Boas V, Deferm N, Devisscher L, Jaeschke H, Annaert P, Vinken M. 2019. Mechanisms and in vitro models of drug-induced cholestasis. Arch Toxicol. 93(5):1169–1186.
- Han W, Ding M, Liu S, Chen Y, Duan Z. 2019. Evaluation of 3D re-cellularized tissue engineering: a drug-induced hepatotoxicity model for hepatoprotectant research. Toxicol Mech Methods. 29(9):654–664.
- Hendriks DF, Fredriksson Puigvert L, Messner S, Mortiz W, Ingelman-Sundberg M. 2016. Hepatic 3D spheroid models for the detection and study of compounds with cholestatic liability. Sci Rep. 6:35434.
- Hewitt NJ, Hewitt P. 2004. Phase I and II enzyme characterization of two sources of HepG2 cell lines. Xenobiotica. 34(3):243–256.
- Hua W, Zhang S, Lu Q, Sun Y, Tan S, Chen F, Tang L. 2021. Protective effects of n-butanol extract and iridoid glycosides of Veronica ciliata Fisch. against ANIT-induced cholestatic liver injury in mice. J Ethnopharmacol. 266:113432.
- Kullak-Ublick GA, Andrade RJ, Merz M, End P, Benesic A, Gerbes AL, Aithal GP. 2017. Drug-induced liver injury: recent advances in diagnosis and risk assessment. Gut. 66(6):1154–1164.
- Kuna L, Bozic I, Kizivat T, Bojanic K, Mrso M, Kralj E, Smolic R, Wu GY, Smolic M. 2018. Models of drug induced liver injury (DILI) – current issues and future perspectives. Curr Drug Metab. 19(10):830–838.
- Li W, Wang G, Wang T, Li Y, Li Y, Lu X, Wang Y, Zhang H, Liu P, Wu J, et al. 2019. Protective effect of herbal medicine Huangqi decoction against chronic cholestatic liver injury by inhibiting bile acid-stimulated inflammation in DDC-induced mice. Phytomedicine. 62:152948.
- McGill MR, Jaeschke H. 2019. Animal models of drug-induced liver injury. Biochim Biophys Acta Mol Basis Dis. 1865(5):1031–1039.
- Padda MS, Sanchez M, Akhtar AJ, Boyer JL. 2011. Drug-induced cholestasis. Hepatology. 53(4):1377–1387.
- Petrov PD, Fernández-Murga ML, López-Riera M, Goméz-Lechón MJ, Castell JV, Jover R. 2018. Predicting drug-induced cholestasis: preclinical models. Expert Opin Drug Metab Toxicol. 14(7):721–738.
- Ramaiahgari SC, den Braver MW, Herpers B, Terpstra V, Commandeur JN, van de Water B, Price LS. 2014. A 3D in vitro model of differentiated HepG2 cell spheroids with improved liver-like properties for repeated dose high-throughput toxicity studies. Arch Toxicol. 88(5):1083–1095.
- Santiago P, Scheinberg AR, Levy C. 2018. Cholestatic liver diseases: new targets, new therapies. Therap Adv Gastroenterol. 11:1756284818787400.
- Sato K, Zhang W, Safarikia S, Isidan A, Chen A, Li P, Francis H, Kennedy L, Baiocchi L, Alvaro D, et al. 2021. Organoids and spheroids as models for studying cholestatic liver injury and cholangiocarcinoma. Hepatology. 74(1):491–502.
- Shen T, Liu Y, Shang J, Xie Q, Li J, Yan M, Xu J, Niu J, Liu J, Watkins PB, et al. 2019. Incidence and etiology of drug-induced liver injury in mainland China. Gastroenterology. 156(8):2230–2241.
- Sheng P, Li P, Liu P, Yan H, Wang J, Lu W, Liu C, Zhou Y. 2020. Cistanches alleviates sevoflurane-induced cognitive dysfunction by regulating PPAR-γ-dependent antioxidant and anti-inflammatory in rats. J Cell Mol Med. 24(2):1345–1359.
- Sundaram V, Björnsson ES. 2017. Drug-induced cholestasis. Hepatol Commun. 1(8):726–735.
- Todorović Vukotić N, Đorđević J, Pejić S, Đorđević N, Pajović SB. 2021. Antidepressants- and antipsychotics-induced hepatotoxicity. Arch Toxicol. 95(3):767–789.
- Wang G, Li Y, Shi R, Wang T, Li Y, Li W, Zheng M, Fan F, Zou J, Zan B, et al. 2020. Yinchenzhufu decoction protects against alpha-naphthylisothiocyanate-induced acute cholestatic liver injury in mice by ameliorating disordered bile acid homeostasis and inhibiting inflammatory responses. J Ethnopharmacol. 254:112672.
- Wu Q, Liu J, Liu L, Chen Y, Wang J, Leng L, Yu Q, Duan Z, Wang Y. 2018. Establishment of an ex vivo model of nonalcoholic fatty liver disease using a tissue-engineered liver. ACS Biomater Sci Eng. 4(8):3016–3026.
- Wu Y, Chen J, Cheng Y. 2005a. Determination of sophocarpine, matrine, and sophoridine in Kuhuang injection by GC-MS. J Anal Chem. 60(10):967–973.
- Wu Y, Chen J, Cheng Y. 2005b. A sensitive and specific HPLC-MS method for the determination of sophoridine, sophocarpine and matrine in rabbit plasma. Anal Bioanal Chem. 382(7):1595–1600.
- Xiang J, Yang G, Ma C, Wei L, Wu H, Zhang W, Tao X, Jiang L, Liang Z, Kang L, et al. 2021. Tectorigenin alleviates intrahepatic cholestasis by inhibiting hepatic inflammation and bile accumulation via activation of PPARγ. Br J Pharmacol. 178(12):2443–2460.
- Xu X, Ling Q, Gao F, He Z, Xie H, Zheng S. 2009. Hepatoprotective effects of marine and kuhuang in liver transplant recipients. Am J Chin Med. 37(01):27–34.
- Zhang X, Zhang Y, Gao W, Guo Z, Wang K, Liu S, Duan Z, Chen Y. 2021. Naringin improves lipid metabolism in a tissue-engineered liver model of NAFLD and the underlying mechanisms. Life Sci. 277:119487.