Abstract
Context
Sanziguben (SZGB) is an empirical prescription used in traditional Chinese medicine to treat diabetic nephropathy (DN). As an abundant and primarily effective component of SZGB, Sanziguben polysaccharides (SZP) can be digested by flora to generate biological activity.
Objective
Our study aimed to clarify the potential mechanism of SZP in improving chronic DN.
Materials and methods
Male db/db mice were randomized into DN, SZP (500 mg/kg) and metformin (MET, 300 mg/kg) groups. Wild-type littermates served as the normal control (NC) group. The drug was orally administered for 8 weeks. Enzyme-linked immunosorbent assay was used to detect the inflammatory factors. Proteins related to inflammation were evaluated using western blotting and immunohistochemical examination. Gut microbiota was analysed using 16S rRNA sequencing.
Results
SZP significantly reduced 24 h urine albumin (p < 0.05) of DN mice. Compared to DN group, SZP significantly decreased the homeostasis model assessment of insulin resistance index, serum creatinine and blood urea nitrogen levels (20.27 ± 3.50 vs. 33.64 ± 4.85, 19.22 ± 3.77 vs. 32.52 ± 3.05 μmol/L, 13.23 ± 1.42 vs. 16.27 ± 0.77 mmol/L, respectively), and mitigated renal damage. SZP also regulated gut microbiota and decreased the abundance of Gram-negative bacteria (Proteobacteria, Klebsiella and Escherichia-Shigella). Subsequently, SZP reduced lipopolysaccharides levels (1.06- to 1.93-fold) of DN mice. Furthermore, SZP inhibited the expression levels of TLR4, phospho-NF-κB p65, NLRP3 proteins and interleukin (IL)-18 and IL-1β.
Conclusions
These results demonstrated that SZP improved intestinal flora disorder and inhibited the TLR4/NF-κB/NLRP3 pathway to alleviate DN.
Introduction
Diabetes mellitus (DM) is a metabolic disease characterized by glucose metabolism disorder caused by environmental and genetic factors, and it can lead to various complications, such as diabetic nephropathy (DN), diabetic cardiomyopathy and diabetic retinopathy (Guthrie and Guthrie Citation2004; Schmidt Citation2018). Diabetic nephropathy is a severe and common microvascular complication of diabetes (Xiong and Zhou Citation2019) and the major cause of end-stage renal disease, which increases the mortality risk of diabetes (Wada and Makino Citation2013). Therefore, DN is an important cause of death in diabetic patients (Zhang et al. Citation2020). The pathological manifestations of DN include glomerular hypertrophy, mesangial matrix hyperplasia, glomerular basement membrane thickening, more serious glomerular glomerulosclerosis, renal angiosclerosis and tubulointerstitial fibrosis (Tervaert et al. Citation2010). Early DN is characterized by the appearance of urinary microalbumin excretion and associated symptoms, such as hypertension, oedema and even renal failure (Xiong and Zhou Citation2019).
Intestinal flora can participate in the metabolic processes of nutrients and energy and maintain intestinal epithelial homeostasis and immune defence (Ma et al. Citation2019). Recent studies have shown that disorders in intestinal flora are related to the occurrence and development of DN (Chi et al. Citation2021). Dysbiosis of the gut microbiota destroys the integrity of the intestinal epithelium, resulting in the excessive production of endotoxins (lipopolysaccharide, LPS) produced by Gram-negative bacteria, which further damages the mechanical barrier formed by intestinal epithelial cells and the tight junction between them (Stephens and von der Weid Citation2020; Hua et al. Citation2022). Some studies have demonstrated that LPS can enter the blood through the damaged intestinal mucosa, initiating diabetic renal inflammation (Fritsche Citation2015). In addition, a study showed that renal monocyte infiltration in kidney tissue affects the expression of the NLRP3 inflammasome, and NLRP3 inflammasome induces the secretion of the pro-inflammatory cytokines interleukin-1β (IL-1β) and interleukin-18 (IL-18) by activating caspase-1, which promotes cell death and induces automatic defence and inflammatory responses (Andrade-Oliveira et al. Citation2019). The NLRP3 inflammasome can be induced and activated by the pro-inflammatory mediator LPS, and the persistent inflammatory condition caused by its activation is involved in the occurrence and progression of DN (Yang et al. Citation2021).
At present, the drugs used to treat DN are mainly synthetic hypoglycaemic agents (Samsu Citation2021). However, prolonged or overdose of these drugs triggers several side effects, such as gastrointestinal disturbances and allergies (Ayodele et al. Citation2004). Therefore, bioactive components of natural plants with no or low toxicity effects have gained wide interest in recent years. Polysaccharides are widely distributed in nature and exhibit a broad range of biological activities, including antioxidant, antimicrobial, antidiabetic, antitumor and immune regulatory activities (Dedhia et al. Citation2022; Mukherjee et al. Citation2022). These biological activities allow for their wide application in multiple diseases (Zeng et al. Citation2019). However, as macromolecular compounds, polysaccharides cannot be directly absorbed into the blood but exert biological activities via intestinal microflora. The gut microbiota can participate in the metabolism of polysaccharides, and its composition and function are affected by the active ingredients of polysaccharides to produce beneficial effects on diseases (Ho Do et al. Citation2021).
Sanziguben (SZGB) consists of Rosae laevigatae Michx. (Rosaceae), Phyllanthus emblica L. (Phyllanthaceae), Schisandra chinensis (Turcz.) Baill. (Schisandraceae) and Gynostemma pentaphyllum (Thunb.) Makino (Cucurbitaceae), an empirical prescription for the clinical treatment of DN and protection against kidney injury. Our previous research found that Sanziguben polysaccharides (SZP) have beneficial effects on renal functions in DN mice induced by a high-fat diet along with a low dose of streptozocin through an anti-inflammatory effect (Zhou et al. Citation2021). Therefore, this study further investigated the protective effects of SZP against DN in db/db mice and its potential mechanism of action.
Materials and methods
Plant materials used and preparation of the polysaccharides
Dried ripe fruits of R. laevigatae were purchased from Bozhou Shenglin Pharmaceutical Industry Co., Ltd. (Bozhou, China), dried ripe fruits of P. emblica and S. chinensis were obtained from Zhongshan Zhongzhi Pharmaceutical Group Co., Ltd. (Zhongshan, China), and dried whole grass of G. pentaphyllum was purchased from Hunan Bestcome Traditional Medicine Co., Ltd. (Hunan, China). All herbs were identified and authenticated by Dr. Guangtian Peng, a medicinal botanist in the Guangzhou University of Chinese Medicine. The voucher specimens (JYZ-21-WF, YGZ-21-WF, WWZ-21-WF and JGL-21-WF, respectively) were deposited at the authors’ laboratory in Guangzhou University of Chinese Medicine.
The above Chinese herbs were mixed in a ratio of 6:5:3:6. After 24 h of desiccation, the SZGB was crushed and screened through a 50-mesh sieve and degreased three-times with petroleum ether (1:3) for 24 h at room temperature under reflux. After filtration, the residue was extracted twice with distilled water (1:12) for 5 h at 80 °C. The extracts were concentrated by rotary vacuum evaporation. The concentrate was precipitated using absolute ethanol. The precipitate was then collected and dissolved in distilled water. The step of deproteinization with the Sevage method was added based on a previous SZP extraction technique (Zhou et al. Citation2021). Finally, the deproteinized solution was lyophilized to obtain crude polysaccharides (SZP). Finally, the phenol–sulphuric acid method, carbazole–sulphuric acid method, barium chloride–gelatin method and the bicinchoninic acid (BCA)-based assays were applied to detect the neutral sugar, uronic acid, sulphate groups and protein content, respectively.
Chemicals
Metformin (MET) was obtained from Sino-American Shanghai Squibb Pharmaceuticals, Ltd. (Shanghai, China). All assay kits were purchased from Nanjing Jiancheng Bioengineering Institute (Nanjing, China). Mouse insulin (CSB-E05071m), LPS (CSB-E13066m), IL-18 (CSB-EO4609) and IL-1β (CSB-E08054) ELISA kits were provided by HuaMei Co. (Shanghai, China). Tissue lysates were prepared using radioimmunoprecipitation assay (RIPA) lysis buffer (CWBIO) containing a protease/phosphatase inhibitor cocktail. The following primary antibodies were used: TLR4 (Affinity, San Francisco, CA, AF7017), NF-κB p65 (CST, Boston, MA, #8242), phospho-NF-κB p65 (CST, Boston, MA, #3033), NLRP3 (Abcam, Cambridge, UK, ab263899), ASC (Affinity, San Francisco, CA, AF6304), caspase-1 (Affinity, San Francisco, CA, AF5418), ZO-1 (CST, Boston, MA, #8193), occludin (Abcam, Cambridge, UK, ab216327) and β-actin (CWBIO, Beijing, China, CW0096). The secondary antibodies used were goat anti-rabbit IgG (CWBIO, Beijing, China) and goat anti-mouse IgG (CWBIO, Beijing, China).
Animals
Six-week-old male db/db and wild-type (WT) littermate mice were purchased from GemPharmatech Co., Ltd. (animal production license number: SCXK2020-0054) (Nanjing, China). The animals were maintained at the Guangzhou University of Chinese Medicine (animal use license number: SYXK2019-0202). A total of 10 WT mice and 30 db/db mice were reared on alternating 12 h light/dark cycle conditions at a relatively constant temperature of 22 ± 2 °C in the study. All animal experiments were approved by the Laboratory Animal Ethics Committee of Guangzhou University of Chinese Medicine (approved identification: ZYD-2021-166) and performed in accordance with ethical standards.
Experimental design
The db/db mice were divided randomly into three groups of 10 mice each: DN control group (DN), DN + 300 mg/kg MET group (MET) and DN + 500 mg/kg SZP group (SZP), WT mice were assigned to the normal control (NC) group and gavaged with an equal volume of distilled water. Fasting blood glucose (FBG) levels of 8-week-old mice were measured using handheld glucometers (Accu-Chek, Roche, Munich, Germany). FBG levels of db/db mice higher than 16.7 mmol/L were considered as diabetic mice. Urine was collected from db/db mice using metabolic cages on the 8th weekend. The urine of db/db mice with >150% WT was considered as DN mice. Drug treatment was initiated 9 weeks after the successful establishment of the DN model and was administered for eight consecutive weeks. The body weights of the mice were monitored weekly, and the food intake and volume of water intake were measured once a week. In the concurrent phase, the FBG levels of mice were measured once every 2 weeks, and urine was monitored once every 4 weeks. Serum, cecal contents and kidneys were harvested after 8 weeks.
Detection of serum biochemical indexes
Serum samples were obtained after centrifugation of blood samples at 3000 rpm for 10 min. The serum levels of triglyceride (TG), total cholesterol (T-CHO), serum creatinine (SCr) and blood urea nitrogen (BUN) were measured according to the manufacturer’s instructions.
Renal oxidative stress and inflammatory factors assay
Renal tissue was homogenized in cold 0.9% saline to obtain a 10% homogenate. The supernatant was collected via centrifugation. As per manufacturer’s instruction, the levels of superoxide dismutase (SOD), catalase (CAT) and malondialdehyde (MDA) in renal tissue homogenate were determined with corresponding assay kits. Kidney endotoxin, IL-18 and IL-1β levels were quantified using ELISA kits.
Histology analysis
To evaluate the degree of renal histopathological damage, we stained renal sections with haematoxylin and eosin (H&E), Masson, periodic acid-Schiff (PAS) and Sirius red. Briefly, fresh renal tissues were fixed in 4% paraformaldehyde for 24 h and embedded in paraffin. Thereafter, 4 µm sections of the tissue samples were obtained and staining was performed as described above. Morphology, fibrosis, glycogen deposition and collagen deposition in tissues were observed by H&E, Masson, PAS and Sirius red staining.
Intestinal microbiota composition analysis
According to the manufacturer’s instructions, total microbial community genomic DNA was extracted from the cecal contents of mice using the E.Z.N.A.® soil DNA Kit (Omega Bio-tek, Norcross, GA). The mentioned bacterial DNA extraction was employed as the template using slightly modified versions of upstream primer 338F (5′-ACTCCTACGGGGGCAGCAG-3TC sequence and downstream primer 806R (5′-GGACTACVGGGTWTCTAAT-3′) to amplify gene V3–V4 hypervariable region of the 16S rRNA by ABI GeneAmp® 9700 PCR thermocycler (ABI, Foster City, CA). Purified PCR products were built using the NEXTFLEX Rapid DNA-Seq Kit, and sequencing was performed using the Illumina MiSeq PE300 platform/NovaSeq PE250 platform (Illumina, San Diego, CA) and analysed according to the manufacturer’s instructions (Majorbio Technology Co., Ltd., Shanghai, China).
Immunohistochemical staining
Renal tissue sections were incubated with EDTA for antigen retrieval. Thereafter, the slides were incubated with TLR4, NLRP3, ZO-1 and occludin antibodies (1:500 dilution) for 40 min at 37 °C. The slides were washed three times with phosphate-buffered saline for 5 min and incubated with the secondary antibody for 20 min at 37 °C. After washing, the slides were observed under a light microscope.
Western blot analysis
Kidney tissues were homogenized in RIPA lysis buffer containing 1 mM phenylmethanesulfonyl fluoride and protease inhibitors. The supernatant was obtained after centrifugation, and the protein concentration was determined using BCA assay, after which 5× loading buffer was added and boiled for 10 min. Protein samples were transferred onto polyvinylidene fluoride membranes after electrophoresis. Anti-NLRP3, anti-ASC, anti-caspase1, anti-TLR4, anti-NF-κB p65 and anti-NF-κB p-p65 antibodies were incubated overnight at 4 °C on a shaker. The membranes were washed five times with Tris-buffered saline with Tween 20 (TBST) for 6 min and incubated with the secondary antibodies for 1 h at room temperature. After incubation, the membranes were washed five times with TBST for 6 min. Finally, the membranes were monitored with an enhanced chemiluminescence reagent and quantified using Image J software (Bethesda, MD).
Statistical analysis
The results are expressed as mean ± SD. All data were analysed using SPSS (SPSS Inc., Chicago, IL) and GraphPad Prism 8.0.2 (GraphPad Software, La Jolla, CA). Statistically significant differences were determined by one-way analysis of variance and reported as p < 0.05.
Results
SZP content
The SZP extracted from SZBG was a brownish-yellow powder. The yield and content of the total polysaccharides obtained from SZP were 6.92% and 88.18%, respectively. In addition, 53.37 ± 4.78% neutral sugar, 27.39 ± 2.83% uronic acid and 7.42±0.64% sulphate groups of SZP were obtained, and the content of protein was reduced to 7.73 ± 0.68% after optimized the process of SZP extraction and purification ().
Table 1. Composition of SZP.
SZP alleviated DN in db/db mice
The effects of SZP were evaluated in a db/db DN mouse model. A flow diagram of the study is shown in . Weekly body weights were monitored during drug administration. It was found that there was no significant change in the body weight () of DN mice between the different treatment groups. Based on the weekly water and food intake results, DN mice showed a significant decrease in water intake () but only a slight effect in food intake () after SZP and MET treatment. As shown in , FBG, serum insulin, and the homeostasis model assessment of insulin resistance (HOMA-IR) index were significantly decreased after SZP and MET treatment compared to those in the DN group. These findings illustrate that SZP and MET played a role in improving the symptoms of polydipsia, lowering blood glucose and improving insulin resistance.
Figure 1. Sanziguben polysaccharides (SZP) ameliorated renal injury in diabetic nephropathy (DN) mice after 8 weeks of administration. (A) Study flow diagram, (B) body weight, (C) water intake, (D) food intake, (E) fasting blood glucose (FBG), (F) serum insulin, (G) the homeostasis model assessment of insulin resistance (HOMA-IR) index, (H) triglyceride (TG), (I) total cholesterol (T-CHO), (J) urine albumin, (K) renal weight, (L) renal index, (M) serum creatinine (SCr), (N) blood urea nitrogen (BUN), (O) catalase (CAT), (P) superoxide dismutase (SOD), (Q) malondialdehyde (MDA), (R) histology (haematoxylin and eosin (H&E), periodic acid-Schiff (PAS), Masson and Sirius red staining). All data were expressed as the mean ± SD (n = 6). ##p< 0.01, ###p< 0.001 vs. NC group; *p< 0.05, **p< 0.01, ***p< 0.001 vs. DN group.
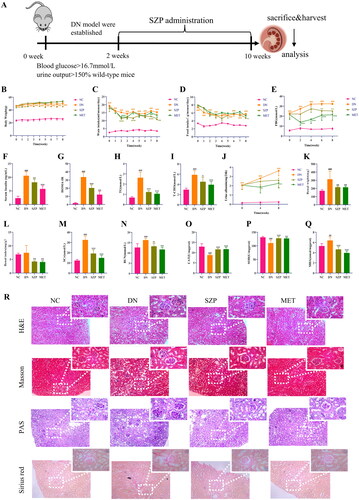
As shown in , serum TG and T-CHO levels were considerably reduced after SZP and MET administration compared to those in the DN group. As estimated by the KW/BW ratio, SZP and MET treatment markedly reversed the changes in renal weight and renal index compared to those in the DN group (). The results showed that SZP and MET treatment attenuated kidney enlargement in mice with DN. Further observations on 24 h proteinuria and serum levels of BUN and SCr were performed to evaluate the effects of SZP and MET treatment in DN mice (). We observed that the levels of the aforementioned indicators were significantly higher in DN mice, indicating renal metabolic dysfunction, compared to the NC group. These three indicators were markedly reduced after 8 consecutive weeks of SZP and MET treatment. These findings revealed that SZP and MET treatment improved DN-induced metabolic abnormalities in renal and blood lipids.
The levels of CAT and SOD in the kidney tissue were significantly decreased, whereas the level of MDA was significantly higher in DN mice than that in the NC group (), showing that the response to oxidative stress was enhanced in kidneys of mice with DN. After SZP and MET treatment, the levels of CAT and SOD were significantly increased, whereas the level of MDA was considerably reduced. These results suggested that SZP and MET treatment improved renal oxidative stress and renal function in DN mice.
SZP ameliorated kidney injury and fibrosis in DN mice
The results of the pathological section analysis are summarized in , the HE-stained pathological section showed glomerular enlargement and mesangial hyperplasia, inflammatory cell infiltration, and fibrosis of renal interstitium in DN mice. The improvement of renal injury in the SZP and MET groups was significant compared to that in the DN group, which is specifically shown in glomeruli, inflammatory cell infiltration, and fibrosis of the renal interstitium. PAS staining revealed glomerular hypertrophy, glomerular basement mesangial hyperplasia and glycogen deposition in the DN mice. Compared to the DN group, these pathological changes were ameliorated in the SZP and MET groups. Masson and Sirius red staining revealed glomerular basement mesangial hyperplasia and heavy collagen deposition in the DN mice. The improvement in the collagen deposition area in the SZP and MET groups was significant compared to that in the DN group. In summary, these results indicate that SZP and MET treatment effectively protected against renal injury and fibrosis.
SZP improved the dysbiosis of gut microbiota
Faecal microbiota diversity and composition were analysed to evaluate the effects of SZP on the intestinal flora. The Shannon index at the operational taxonomic unit level indicated that the current sequencing quantity was sufficiently large to reflect the vast majority of microorganisms species (). The Chao, ACE, Shannon and Simpson indices were used to reflect the diversity and richness of the gut microbiome. The results showed that the diversity and richness of faecal microbiota were considerably reduced in DN mice compared to those in NC mice (). After SZP treatment, the Chao and ACE indices were significantly elevated, indicating that faecal microbiota richness increased. However, there were no visible effects on faecal microbiota diversity. We next investigated the β-diversity of the faecal microbiota and demonstrated that the gut microbiome was remodelled by SZP treatment ().
Figure 2. Sanziguben polysaccharides (SZP) improved gut microbiota in diabetic nephropathy (DN) mice. (A) Shannon index curve. The alpha diversity of the gut microbiome: (B) ACE index, (C) Chao index, (D) Shannon index and (E) Simpson index. (F) Unweighted UniFrac PCoA (principal coordinates analysis). (G) Relative abundance of intestinal microbiota at the phylum level, (H) Proteobacteria and (I) Verrucomicrobiota. (J)Abundance changes in representative bacteria at the genus level are shown in a heatmap. Genera that were statistically different with SZP treatment: the relative abundance of (K) Akkermansia, (L) Klebsiella, (M) Marvinbryantia, (N) Blautia, (O) Escherichia-Shigella and (P) Eubacterium_xylanophilum_group. All data were expressed as the mean ± SD (n = 6). #p< 0.05, ##p< 0.01, ###p< 0.001 vs. NC group; *p< 0.05, **p< 0.01 vs. DN group.
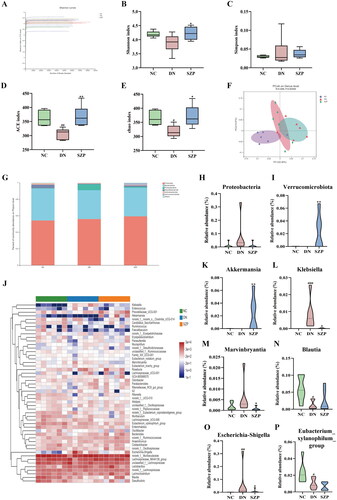
To further evaluate the impact of SZP administration on the relative abundance of intestinal microbes, we analysed the differential bacterial composition at the phylum and genus levels. As shown in , the microbial composition at the phylum level in the three groups was significantly different. Compared with the DN group, the abundance of the phylum Verrucomicrobia was visibly elevated, whereas the abundance of Proteobacteria generally decreased after SZP treatment (). At the genus level, the abundance of Akkermansia was markedly increased, while the abundance of Klebsiella, Escherichia-Shigella and Marvinbryantia were clearly reduced in SZP group as compared to the DN group (). These findings reveal that dysbiosis of the gut microbiota was improved by SZP treatment.
SZP suppressed excessive inflammation in DN and its effects were mediated via inhibiting the TLR4/NF-κB/NLRP3 pathway
The cell–cell junctions of the kidney were disrupted and the intestinal barrier was damaged in DN, which led to decreased levels of ZO-1 and occludin. Thus, the kidney receives LPS leaking from the intestine into the blood, which leads to the infiltration of inflammatory cells in the renal tissue. Inflammation is a typical feature of DN, and LPS can exacerbate inflammatory responses (Yang et al. Citation2020). Our results indicated that SZP treatment significantly reduced inflammatory infiltration of the kidney, as demonstrated by the reduced levels of LPS, IL-18 and IL-1β (). The protein expression levels of ZO-1 and occludin in colonic tissues and their immunohistochemical expression in renal tissues were significantly increased by SZP treatment ( and ), demonstrating that SZP treatment repaired the endothelial barrier integrity of kidney and colonic tissues in DN.
Figure 3. Sanziguben polysaccharides (SZP) inhibited the inflammatory factors, altered the protein levels of the TLR4/NF-κB/NLRP3 pathway and kidney barrier in kidney tissues. (A) Lipopolysaccharide (LPS), (B) interleukin (IL)-18 and (C) IL-1β levels in kidney were determined by enzyme-linked immunosorbent assay (ELISA) (n = 6, mean ± SD). Expression of (D) TLR4, (E) the ratio of NF-κB p-p65 to NF-κB p65, (F) NLRP3, (G) ASC, (H) caspase-1, evaluated by western blot analysis (n = 3, mean ± SD). Representative immunohistochemistry (IHC) result for (I) TLR4, (G) NLRP3, (K) ZO-1, (L) occludin (n = 3, mean ± SD). ##p< 0.01, ###p< 0.001 vs. NC group; *p< 0.05, **p< 0.01, ***p< 0.001 vs. diabetic nephropathy (DN) group.
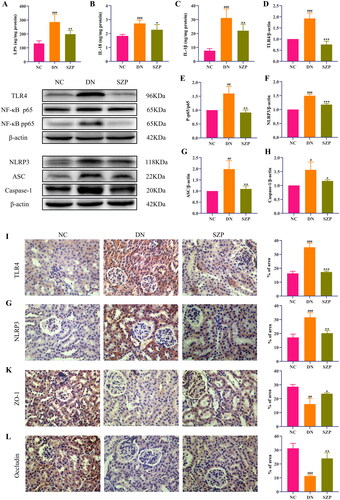
Figure 4. Sanziguben polysaccharides (SZP) improved tight junction proteins of gut barrier. Western blot analysis of (A) ZO-1 and (B) occludin proteins in colon tissue (n = 3, mean ± SD). ##p< 0.01, ###p< 0.001 vs. NC group; *p< 0.05, **p< 0.01 vs. diabetic nephropathy (DN) group.
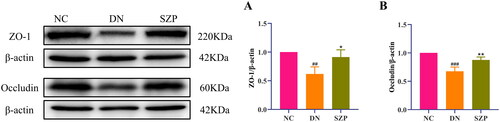
The NLRP3 inflammasome comprises various protein complexes that regulate IL-1β and IL-18 secretion. It has been reported that the regulation of TLR4 and the downstream signal NF-κB/NLRP3 have therapeutic implications in DN (Lee et al. Citation2019; Ram et al. Citation2020). To further explore the underlying mechanism of the role of SZP in DN, the protein expression levels of TLR4, NF-κBp65, NF-κB p-p65, NLRP3, ASC and caspase-1 were examined in renal tissues. The western blotting results revealed that the protein levels of TLR4, NF-κB p-p65, NLRP3, ASC and caspase-1 were significantly increased in DN mice, whereas these effects were reversed by SZP treatment (). In addition, immunohistochemistry results indicated that TLR4 and NLRP3 protein expression levels were decreased in the SZP treatment group (). These results imply that SZP could mitigate the inflammatory response in the kidney by inhibiting the TLR4/NF-κB/NLRP3 pathway.
Discussion
In recent years, intestinal flora has received wide attention in the pathogenesis of DN (Yang et al. Citation2021). Growing evidence has revealed that Chinese medicinal polysaccharides influence intestinal microflora and exert pharmacological effects (Zhang et al. Citation2018; Ge et al. Citation2021). In the present study, we found that the intestinal microbiota regulated by SZP might be a potential therapeutic strategy to treat DN. Through 16S rRNA analysis, we found that SZP regulated the dysregulated intestinal flora and reduced the abundance of Gram-negative bacteria to reduce the release of LPS, thereby improving the colon epithelial barrier and further diminishing the damage of inflammation to the kidney.
db/db mice are considered a good animal model for DN (Nørgaard et al. Citation2019; Guo et al. Citation2020). In the DN group, body weight, water and food intake, and excretion were increased compared to those in the NC group. DN mice showed a significant decrease in water intake but only a slight effect in food intake after SZP and MET treatment. This might be related to the fact that DN mice showed no significant weight loss after SZP and MET treatment. These results indicated that SZP might not improve the weight of DN mice by controlling food intake. Insulin resistance is a key link in the occurrence and development of DN (Svensson and Eriksson Citation2006), and HOMA-IR is a reliable marker of insulin resistance (Ekblad et al. Citation2015; Cho et al. Citation2018). In this study, SZP-treated DN mice showed significantly decreased FBG, serum insulin and HOMA-IR index. These results suggest that SZP may improve insulin resistance. DN is characterized by proteinuria and renal injury (Wada and Makino Citation2013), whereas SCr and BUN are generally used to evaluate the degree of renal injury (Tang et al. Citation2021). In this study, we found that after supplementation with SZP, the levels of 24 h urine albumin, renal index, SCr and BUN were significantly decreased in DN mice, indicating that SZP could improve renal enlargement and renal metabolic dysfunction in DN mice. Owing to the close correlation between glucose and lipid metabolism, the development of DN is associated with abnormal lipid metabolism (Herman-Edelstein et al. Citation2014). TG and T-CHO, which are indicators of blood lipid metabolism, were also reversed in DN mice after SZP treatment, indicating that SZP could effectively ameliorate DN-induced dyslipidaemia. Diabetes is also accompanied by a disorder in glucose metabolism in the renal tissue (Warren et al. Citation2019). Abnormal glucose metabolism caused by hyperglycemia catalyses the production of large amounts of reactive oxygen species, which enhances oxidative stress. SOD and CAT are important biological enzymes that scavenge free radicals in the body, and MDA is a lipid peroxidation biomarker that reflects the extent of membrane damage (Ding et al. Citation2018). These results were consistent with our results, SZP treatment effectively improved renal oxidative stress, as evidenced by significantly increased levels of antioxidant enzymes SOD and CAT, and significantly decreased MDA levels. In addition, we observed the effect of SZP treatment on the renal structure of DN mice by the pathological staining of the renal tissue. H&E and PAS staining mainly reflected changes in the renal structure and glycogen accumulation in DN mice. Masson and Sirius red staining can be used to stain collagen fibres. Histological staining showed that SZP treatment alleviated renal damage in DN mice, reduced the deposition of glycogen and collagen fibres, and significantly reduced the levels of inflammatory factors.
Our study is consistent with previous studies reporting decreased intestinal microbiota diversity in DN mice and a higher abundance of LPS-producing protein bacteria (Sabatino et al. Citation2017; Cai et al. Citation2020). We found that Proteobacteria, Klebsiella and Escherichia-Shigella were abundantly enriched in DN mice. As a Gram-negative bacterium, Proteobacteria produces a large amount of LPS, which increases the oxygen level in the lumen and leads to an imbalanced structure in the intestine, which is closely related to the severity of DN (Hu et al. Citation2020). Klebsiella is a Gram-negative bacterium that produces various pathogenic factors, including multiple adhesins, capsular polysaccharides and LPS (Linh et al. Citation2022). Besides, Escherichia-Shigella, which is a Gram-negative bacteria, belongs to Escherichia coli enriched in DN patients (Thingholm et al. Citation2019; Hu et al. Citation2020). After SZP treatment, the abundance of Gram-negative bacteria, such as Proteobacteria, Klebsiella and Escherichia-Shigella, was remarkedly reduced. Previous studies have shown that the abundance of Marvinbryantia is significantly increased in DN and positively correlated with serum MDA levels and inflammatory factors in the intestinal mucosa (Haenen et al. Citation2013; Zhang et al. Citation2020), which is in line with the results of our study. Compared with DN mice, the abundance of Marvinbryantia was reversed after SZP treatment. Gut microbes are essential for maintaining the integrity of the intestinal epithelium and for protecting the intestinal barrier. Previously, it was demonstrated that Verrucomicrobia is responsible for the regulation of intestinal mucosal health (Barlow et al. Citation2015). Akkermansia was confirmed to be positively associated with MET treatment for diabetes and with the expression of tight junction proteins and the integrity of the intestinal barrier (Gryaznova et al. Citation2022). Interestingly, Verrucomicrobia and Akkermansia were individually enriched in the SZP group, suggesting that SZP treatment enhanced intestinal barrier function. This was clearly supported by the balance between the composition of the microbiota and the increased expression levels of tight junction proteins. These results confirmed that SZP treatment modulated gut microbiota, reduced the abundance of harmful intestinal bacteria and increased the abundance of beneficial intestinal bacteria.
Based on the 16S rRNA gene sequencing results of the gut microflora, we found that the abundance of Gram-negative bacteria was remarkably higher in DN mice. LPS, an essential component of the cell wall of Gram-negative bacteria that induces metabolic inflammatory responses, is released following bacterial cell death cleavage (Fritsche Citation2015). Under physiological conditions, low concentrations of non-pathogenic LPS can be detected in the blood circulation. However, with an increase in the abundance of Gram-negative bacteria, large amounts of LPS are released in the intestine, which damages the intestinal mucosal barrier and increases intestinal permeability. Due to the gut–kidney axis, the kidneys receive large amounts of LPS that accumulate in the intestine and leak into blood circulation (Hua et al. Citation2022). A previous study showed that the NLRP3 inflammasome is composed of ASC, and caspase-1 participates in the inflammatory response in DN and is related to the disorder of intestinal flora (Ding et al. Citation2018). LPS induces NF-κB by activating TLR4 pattern recognition receptor signal transduction and further activates NLRP3 (Niu et al. Citation2022). The activated NLRP3 inflammasome converts the precursor pro-inflammatory cytokines IL-18 and IL-1β into their bioactive forms, further aggravating the inflammatory reaction and renal damage (Yang et al. Citation2021). Our results also indicated that the levels of LPS and pro-inflammatory cytokines and the expression levels of TLR4/NF-κB/NLRP3 pathway-related proteins were significantly increased. These data indicate that the inflammasome in the kidney of DN mice was highly activated and aggravated inflammation. The intestinal mucosal barrier and renal LPS content were improved by SZP treatment, which inhibited inflammation by blocking the activation of the TLR4/NF-κB/NLRP3 signalling pathway.
Conclusions
The results of our study indicate that SZP played a protective role in DN. The mechanisms may involve stabilizing the gut microbiota, upregulating tight junction protein expression levels, reducing the levels of LPS, and suppressing the activation of the TLR4/NF-κB/NLRP3 signalling pathway to improve inflammation, and eventually alleviate renal injuries in DN mice. Therefore, SZP as a natural supplement could be considered potentially effective agent for the treatment of DN.
Author contributions
Fan Wang: conceptualization, methodology, investigation, writing – original draft, writing – review and editing, data curation. Chang Liu: writing – review and editing. LingZhi Ren: writing – original draft. YanYang Li: writing – review and editing. HongMei Yang: writing – original draft. Yang Yu: resources, writing – review and editing, supervision. WeiPing Xu: supervision, writing – review and editing, project administration, funding acquisition.
Acknowledgements
We thank Dr. Yang Yu for assistance with experimental design, and also thank Shanghai Majorbio Technology Co., Ltd. for sequence production and sample management.
Disclosure statement
The authors declare that the research has no competing interests.
Additional information
Funding
References
- Andrade-Oliveira V, Foresto-Neto O, Watanabe I, Zatz R, Câmara N. 2019. Inflammation in renal diseases: new and old players. Front Pharmacol. 10:1192.
- Ayodele OE, Alebiosu CO, Salako BL. 2004. Diabetic nephropathy – a review of the natural history, burden, risk factors and treatment. J Natl Med Assoc. 96(11):1445–1454.
- Barlow GM, Yu A, Mathur R. 2015. Role of the gut microbiome in obesity and diabetes mellitus. Nutr Clin Pract. 30(6):787–797.
- Cai TT, Ye XL, Li RR, Chen H, Wang YY, Yong HJ, Pan ML, Lu W, Tang Y, Miao H, et al. 2020. Resveratrol modulates the gut microbiota and inflammation to protect against diabetic nephropathy in mice. Front Pharmacol. 11:1249.
- Chi M, Ma K, Wang J, Ding Z, Li Y, Zhu S, Liang X, Zhang Q, Song L, Liu C. 2021. The immunomodulatory effect of the gut microbiota in kidney disease. J Immunol Res. 2021:5516035.
- Cho JH, Lee SS, Han KD, Joo YH. 2018. Insulin resistance is associated with chronic laryngitis in Korean women. J Nutr Health Aging. 22(4):471–475.
- Dedhia N, Marathe SJ, Singhal RS. 2022. Food polysaccharides: a review on emerging microbial sources, bioactivities, nanoformulations and safety considerations. Carbohydr Polym. 287:119355.
- Ding T, Wang S, Zhang X, Zai W, Fan J, Chen W, Bian Q, Luan J, Shen Y, Zhang Y, et al. 2018. Kidney protection effects of dihydroquercetin on diabetic nephropathy through suppressing ROS and NLRP3 inflammasome. Phytomedicine. 41:45–53.
- Ekblad LL, Rinne JO, Puukka PJ, Laine HK, Ahtiluoto SE, Sulkava RO, Viitanen MH, Jula AM. 2015. Insulin resistance is associated with poorer verbal fluency performance in women. Diabetologia. 58(11):2545–2553.
- Fritsche KL. 2015. The science of fatty acids and inflammation. Adv Nutr. 6(3):293S–301S.
- Ge Y, Ahmed S, Yao W, You L, Zheng J, Hileuskaya K. 2021. Regulation effects of indigestible dietary polysaccharides on intestinal microflora: an overview. J Food Biochem. 45:13564.
- Gryaznova M, Dvoretskaya Y, Burakova I, Syromyatnikov M, Popov E, Kokina A, Mikhaylov E, Popov V. 2022. Dynamics of changes in the gut microbiota of healthy mice fed with lactic acid bacteria and Bifidobacteria. Microorganisms. 10:1020.
- Guo Y, Ran Z, Zhang Y, Song Z, Wang L, Yao L, Zhang M, Xin J, Mao X. 2020. Marein ameliorates diabetic nephropathy by inhibiting renal sodium glucose transporter 2 and activating the AMPK signaling pathway in db/db mice and high glucose-treated HK-2 cells. Biomed Pharmacother. 131:110684.
- Guthrie RA, Guthrie DW. 2004. Pathophysiology of diabetes mellitus. Crit Care Nurs Q. 27(2):113–125.
- Haenen D, Zhang J, Souza da Silva C, Bosch G, van der Meer IM, van Arkel J, van den Borne JJ, Pérez Gutiérrez O, Smidt H, Kemp B, et al. 2013. A diet high in resistant starch modulates microbiota composition, SCFA concentrations, and gene expression in pig intestine. J Nutr. 143(3):274–283.
- Herman-Edelstein M, Scherzer P, Tobar A, Levi M, Gafter U. 2014. Altered renal lipid metabolism and renal lipid accumulation in human diabetic nephropathy. J Lipid Res. 55(3):561–572.
- Ho Do M, Seo YS, Park HY. 2021. Polysaccharides: bowel health and gut microbiota. Crit Rev Food Sci Nutr. 61(7):1212–1224.
- Hu X, Ouyang S, Xie Y, Gong Z, Du J. 2020. Characterizing the gut microbiota in patients with chronic kidney disease. Postgrad Med. 132(6):495–505.
- Hua Q, Han Y, Zhao H, Zhang H, Yan B, Pei S, He X, Li Y, Meng X, Chen L, et al. 2022. Punicalagin alleviates renal injury via the gut–kidney axis in high-fat diet-induced diabetic mice. Food Funct. 13(2):867–879.
- Lee JH, Sarker MK, Choi H, Shin D, Kim D, Jun HS. 2019. Lysophosphatidic acid receptor 1 inhibitor, AM095, attenuates diabetic nephropathy in mice by downregulation of TLR4/NF-κB signaling and NADPH oxidase. Biochim Biophys Acta Mol Basis Dis. 1865(6):1332–1340.
- Linh HT, Iwata Y, Senda Y, Sakai-Takemori Y, Nakade Y, Oshima M, Nakagawa-Yoneda S, Ogura H, Sato K, Minami T, et al. 2022. Intestinal bacterial translocation contributes to diabetic kidney disease. J Am Soc Nephrol. 33(6):1105–1119.
- Ma Q, Li Y, Li P, Wang M, Wang J, Tang Z, Wang T, Luo L, Wang C, Wang T, et al. 2019. Research progress in the relationship between type 2 diabetes mellitus and intestinal flora. Biomed Pharmacother. 117:109138.
- Mukherjee S, Jana S, Khawas S, Kicuntod J, Marschall M, Ray B, Ray S. 2022. Synthesis, molecular features and biological activities of modified plant polysaccharides. Carbohydr Polym. 289:119299.
- Niu X, Song H, Xiao X, Yu J, Yu J, Yang Y, Huang Q, Zang L, Han T, Zhang D, et al. 2022. Tectoridin alleviates lipopolysaccharide-induced inflammation via inhibiting TLR4-NF-κB/NLRP3 signaling in vivo and in vitro. Immunopharmacol Immunotoxicol. 44(5):641–655.
- Nørgaard SA, Briand F, Sand FW, Galsgaard ED, Søndergaard H, Sørensen DB, Sulpice T. 2019. Nephropathy in diabetic db/db mice is accelerated by high protein diet and improved by the SGLT2 inhibitor dapagliflozin. Eur J Pharmacol. 860:172537.
- Ram C, Jha AK, Ghosh A, Gairola S, Syed AM, Murty US, Naidu V, Sahu BD. 2020. Targeting NLRP3 inflammasome as a promising approach for treatment of diabetic nephropathy: preclinical evidences with therapeutic approaches. Eur J Pharmacol. 885:173503.
- Sabatino A, Regolisti G, Cosola C, Gesualdo L, Fiaccadori E. 2017. Intestinal microbiota in type 2 diabetes and chronic kidney disease. Curr Diab Rep. 17(3):16.
- Samsu N. 2021. Diabetic nephropathy: challenges in pathogenesis, diagnosis, and treatment. Biomed Res Int. 2021:1497449.
- Schmidt AM. 2018. Highlighting diabetes mellitus: the epidemic continues. Arterioscler Thromb Vasc Biol. 38(1):e1–e8.
- Stephens M, von der Weid PY. 2020. Lipopolysaccharides modulate intestinal epithelial permeability and inflammation in a species-specific manner. Gut Microbes. 11(3):421–432.
- Svensson M, Eriksson JW. 2006. Insulin resistance in diabetic nephropathy – cause or consequence. Diabetes Metab Res Rev. 22(5):401–410.
- Tang G, Li S, Zhang C, Chen H, Wang N, Feng Y. 2021. Clinical efficacies, underlying mechanisms and molecular targets of Chinese medicines for diabetic nephropathy treatment and management. Acta Pharm Sin B. 11(9):2749–2767.
- Tervaert TW, Mooyaart AL, Amann K, Cohen AH, Cook HT, Drachenberg CB, Ferrario F, Fogo AB, Haas M, de Heer E, et al. 2010. Pathologic classification of diabetic nephropathy. J Am Soc Nephrol. 21(4):556–563.
- Thingholm LB, Rühlemann MC, Koch M, Fuqua B, Laucke G, Boehm R, Bang C, Franzosa EA, Hübenthal M, Rahnavard A, et al. 2019. Obese individuals with and without type 2 diabetes show different gut microbial functional capacity and composition. Cell Host Microbe. 26(2):252–264.e10.
- Wada J, Makino H. 2013. Inflammation and the pathogenesis of diabetic nephropathy. Clin Sci. 124(3):139–152.
- Warren AM, Knudsen ST, Cooper ME. 2019. Diabetic nephropathy: an insight into molecular mechanisms and emerging therapies. Expert Opin Ther Targets. 23(7):579–591.
- Xiong Y, Zhou L. 2019. The signaling of cellular senescence in diabetic nephropathy. Oxid Med Cell Longev. 2019:7495629.
- Yang G, Wei J, Liu P, Zhang Q, Tian Y, Hou G, Meng L, Xin Y, Jiang X. 2021. Role of the gut microbiota in type 2 diabetes and related diseases. Metabolism. 117:154712.
- Yang M, Wang X, Han Y, Li C, Wei L, Yang J, Chen W, Zhu X, Sun L. 2021. Targeting the NLRP3 inflammasome in diabetic nephropathy. Curr Med Chem. 28(42):8810–8824.
- Yang R, Li Y, Mehmood S, Yan C, Huang Y, Cai J, Ji J, Pan W, Zhang W, Chen Y. 2020. Polysaccharides from Armillariella tabescens mycelia ameliorate renal damage in type 2 diabetic mice. Int J Biol Macromol. 162:1682–1691.
- Zeng P, Li J, Chen Y, Zhang L. 2019. The structures and biological functions of polysaccharides from traditional Chinese herbs. Prog Mol Biol Transl Sci. 163:423–444.
- Zhang T, Yang Y, Liang Y, Jiao X, Zhao C. 2018. Beneficial effect of intestinal fermentation of natural polysaccharides. Nutrients. 10:1055.
- Zhang XX, Kong J, Yun K. 2020. Prevalence of diabetic nephropathy among patients with type 2 diabetes mellitus in China: a meta-analysis of observational studies. J Diabetes Res. 2020:2315607.
- Zhang Y, Chen L, Hu M, Kim JJ, Lin R, Xu J, Fan L, Qi Y, Wang L, Liu W, et al. 2020. Dietary type 2 resistant starch improves systemic inflammation and intestinal permeability by modulating microbiota and metabolites in aged mice on high-fat diet. Aging. 12(10):9173–9187.
- Zhou K, Zhang J, Liu C, Ou L, Wang F, Yu Y, Wang Y, Bai S. 2021. Sanziguben polysaccharides inhibit diabetic nephropathy through NF-κB-mediated anti-inflammation. Nutr Metab. 18(1):81.