Abstract
Context
Hyperoside (Hyp), one of the active flavones from Rhododendron (Ericaceae), has beneficial effects against cerebrovascular disease. However, the effect of Hyp on vasodilatation has not been elucidated.
Objective
To explore the effect of Hyp on vasodilatation in the cerebral basilar artery (CBA) of Sprague-Dawley (SD) rats suffering with ischaemic–reperfusion (IR) injury.
Materials and methods
Sprague-Dawley rats were randomly divided into sham, model, Hyp, Hyp + channel blocker and channel blocker groups. Hyp (50 mg/kg, IC50 = 18.3 μg/mL) and channel blocker were administered via tail vein injection 30 min before ischaemic, followed by 20 min of ischaemic and 2 h of reperfusion. The vasodilation, hyperpolarization, ELISA assay, haematoxylin–eosin (HE), Nissl staining and channel-associated proteins and qPCR were analysed. Rat CBA smooth muscle cells were isolated to detect the Ca2+ concentration and endothelial cells were isolated to detect apoptosis rate.
Results
Hyp treatment significantly ameliorated the brain damage induced by IR and evoked endothelium-dependent vasodilation rate (47.93 ± 3.09% vs. 2.99 ± 1.53%) and hyperpolarization (–8.15 ± 1.87 mV vs. −0.55 ± 0.42 mV) by increasing the expression of IP3R, PKC, transient receptor potential vanilloid channel 4 (TRPV4), IKCa and SKCa in the CBA. Moreover, Hyp administration significantly reduced the concentration of Ca2+ (49.08 ± 7.74% vs. 83.52 ± 6.93%) and apoptosis rate (11.27 ± 1.89% vs. 23.44 ± 2.19%) in CBA. Furthermore, these beneficial effects of Hyp were blocked by channel blocker.
Discussion and conclusions
Although Hyp showed protective effect in ischaemic stroke, more clinical trial certification is needed due to the difference between animals and humans.
Introduction
Ischaemic stroke is a leading cause of morbidity and mortality worldwide; it is characterized by cerebral ischaemic and hypoxia resulting from an impairment of the cerebral blood supply, which leads to infarction (Lv et al. Citation2021; Yang et al. Citation2021). The etiology of cerebral ischaemic stroke and underlying mechanisms responsible for this condition are complex. A variety of risk factors, such as inflammation, calcium overload and oxidative stress, are considered to contribute to the pathogenesis of cerebral ischaemic stroke (Radu et al. Citation2017). At present, the only drug approved by the FDA for the treatment of ischaemic stroke is a recombinant tissue plasminogen activator (rt-PA) called alteplase. However, its benefits to patients are limited due to its short therapeutic window because the optimal time for drug administration is within 4.5 h after onset (Pan et al. Citation2020). Therefore, exploring novel and effective drugs for treating ischaemic stroke is an essential task.
Vascular tone dysfunction is associated with the progression of ischaemic cerebrovascular disease (Hao et al. Citation2019). The transient receptor potential vanilloid channel 4 (TRPV4), an osmo-mechanosensitive channel, can protect against ischaemic brain injury by reducing cell death, decreasing calcium overload and regulating angiogenesis and neurogenesis (Rakers et al. Citation2017; Chen et al. Citation2018). Previous studies have demonstrated that TRPV4 is vital for influencing cerebral vasodilation through endothelium-derived hyperpolarizing factor (EDHF) production by evoking small conductance calcium-activated potassium channels (SKCa) and medium conductance calcium-activated potassium channels (IKCa), which participate in regulating the arteriole basal tone (Sonkusare et al. Citation2012; Goto et al. Citation2018). However, whether the regulation of TRPV4-mediated vasodilation is beneficial in alleviating ischaemic–reperfusion (IR) injury remains unknown.
Hyperoside (Hyp) is an active ingredient of the total flavones from Rhododendron (Ericaceae), and it has protective properties against oxidative stress, inflammation and Ca2+ influx (Seki et al. Citation2017; Xiao et al. Citation2017). Previous studies have shown that Hyp protects against cerebral ischaemic injury by reducing brain oedema, decreasing infarction size, relaxing cerebrovascular vessels and increasing cerebral blood flow (Bagher and Garland Citation2014; Wang et al. Citation2020). Thus, Hyp is presumed to be a potential therapeutic agent for ameliorating the self-repair process following ischaemic stroke. However, the mechanism of Hyp and its effects on cerebral vasodilation following ischaemic have not yet been fully elucidated.
In the present study, we identify the role and potential molecular mechanisms of Hyp in vasodilatation in the cerebral basilar artery (CBA) of rats with IR injury and schematic of the experimental protocol is shown in . Our results demonstrated that Hyp exerts a neuroprotective effect by upregulating IP3R and PKC expression and subsequently activating TRPV4 channels to stimulate endothelium-dependent vasodilation and ameliorate ischaemic brain injury, suggesting that Hyp could be developed into an effective drug for the treatment of ischaemic stroke.
Figure 1. The schematic of experimental protocols: after seven days of adaptive feeding in SD rats, ischaemic pretreatment was performed, ischaemic was performed for 30 min after 24 h, and then 2 h after reperfusion, relevant factors were detected in serum, HE and Nissl staining were performed in brain tissue, CBA was used to detect vasodilatation and hyperpolarization, endothelial cells in CBA were subjected to WB and flow cytometry, and smooth muscle cells were used to detect Ca2+ concentration.
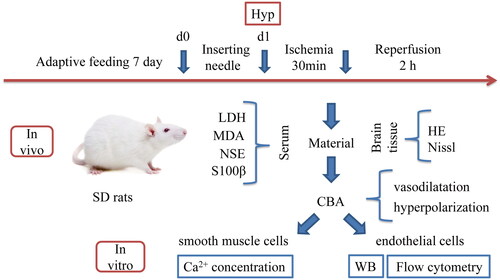
Materials and methods
Animal model and drug treatment
One hundred and twenty male Sprague-Dawley (SD) rats weighing 230–280 g were purchased from the Nanjing Qinglongshan Experimental Animal Company (certificate no. Scxk 2014-0001, Nanjing, China). All rats were maintained in accordance with the US National Institute of Health guidelines (NIH publication no. 85-23, revised 1996) and received approval from the Institutional Animal Care and Use Committee of Wannan Medical College (LLSC-2020-139). The four-vessel occlusion (4-VO) method was employed to establish a global cerebral IR rat model through bilateral carotid artery occlusion, as previously reported (Han et al. Citation2013, Citation2014). Briefly, bilateral vertebral arteries were blocked by inserting an electrocoagulation needle (0.5 mm) into the pterygoid foramen. After 24 h, two non-traumatic arteriole clips were used to simultaneously clamp the bilateral carotid arteries. The body temperature was maintained at 37.0 ± 0.5 °C using a heat lamp. The blood vessels were maintained in ischaemic for 20 min, and then the rats were subjected to 2 h of reperfusion (Zhang et al. Citation2012). The sham-operated animals underwent all surgical procedures except electrocoagulation and occlusion of the carotid arteries. All rats were randomly divided into different groups: sham, IR, IR + Hyp (50 mg/kg), IR + Hyp + HC-067047 (10 mg/kg, IC50 = 133 nM), TRAM-34 (0.5 mg/kg, IC50 = 20 nM), apamin (0.3 mg/kg, IC50 = 1 nM), BisI (2.5 mg/kg IC50 = 20 nM), 2APB (2 mg/kg, IC50 = 42 µM) or BisI + 2APB (2.5 mg/kg + 2 mg/kg) and IR + HC-067047 (10 mg/kg), TRAM-34 (0.5 mg/kg), apamin (0.3 mg/kg), BisI (2.5 mg/kg), 2APB (2 mg/kg) or BisI (2.5 mg/kg) + 2APB (2 mg/kg), with eight rats in each group. Except Sham group, other groups were injected with the above drugs through tail vein 30 min before bilateral common carotid artery occlusion, followed by 20 min of ischaemic and 2 h of reperfusion.
HE and Nissl staining
All rats in each group were sacrificed by CO2 asphyxiation, and the brains were carefully separated from the skull, fixed in 4% formalin, and embedded in paraffin. Formalin-fixed paraffin-embedded coronal sections were processed into 5 μm thick tissue slices for haematoxylin–eosin (HE) and Nissl staining. The hippocampal CA1 region images were captured using an optical microscope.
MDA, NSE, S100β and LDH ELISA assays
All rats were anaesthetized as described above, and then blood samples were collected from the abdominal aorta and centrifuged in a 4 °C precooled centrifuge for 15 min at 3500 rpm. The serum was removed, and the levels of MDA, NSE and S100β and activity of LDH in the serum were measured using ELISA kits according to the manufacturer’s instructions. Samples and standard dilutions were prepared according to the manufacture protocol of the ELISA kits (Calvin Biotechnologies, CK-E30266R, CK-E30447R, CK-E30417R, CK-E30024R,China).
Determination of vasodilation function in isolated cerebral vessels
The CBA segments from different groups were subjected to perfusion with physiological salt solution (PSS) at 150 μL/min and 11.305 kPa by inserting a glass tube through the lumen at the two ends and fixing the microtubules with a surgical line (10-0). l-NAME (30 μmol/L), INDO (10 μmol/L), HC-067047 (10 μmol/L), TRAM-34 (1 μmol/L) and apamin (0.5 μmol/L) were co-incubated with the PSS solution. The average values of the minimum and maximum diameters of the CBA were recorded during vasomotion. The percentage of dilatation was calculated as dilation (%) = [(Dx – Dmin)/(Dmax – Dmin)] × 100%.
Membrane potential of isolated cerebral vessels
First, the rats were sacrificed by CO2 asphyxiation. Then, cerebral vessels were carefully excised from rats under an inverted microscope, fixed in a 10 mL silica gel slot with an average pressure of 85 mmHg, bubbled with PSS containing 95% O2 and 5% CO2, and incubated at a temperature of 37 °C for 1 h. Microelectrodes for the detection of intracellular membrane potential (Em) were applied to vascular endothelial smooth muscle cells (VSMCs) of the CBA. A glass microelectrode (40–80 MΩ, filled with 3 mol/L KCl) was inserted into each VSMC to measure the trends in the intracellular membrane potential (Em) value. The potential difference and interference (50 Hz) were recorded using a conventional high-impedance amplifier and selectively moved aside. The values of Em were monitored and analysed using a MacLab system connected to Chart 5 software. Hyperpolarization of VSMC membranes was recorded as soon as the negative resting membrane potential increased evidently (Petersen Citation2017).
Western blot assay
First, the rats were sacrificed by CO2 asphyxiation. Then, the CBAs of rats selected from the same group were collected to extract endothelial cells. Rat basilar artery endothelial cells were isolated using the MACS method, as described in detail previously (Ohtsuki et al. Citation2007; Han et al. Citation2014). CBA vessels obtained from animals in each group were lysed with RIPA lysis buffer (containing 1% PMSF) in an ice bath for 2 h to extract CBA proteins. A bicinchoninic acid (BCA) kit (Beyotime Institute of Biotechnology, Shanghai, China) was used to measure the total protein concentrations according to the manufacturer’s instructions. Proteins were separated by 10% SDS-PAGE and then transferred to polyvinylidene fluoride membranes. After blocking with 5% nonfat milk for 1 h at room temperature, the membranes were incubated with the following primary antibodies at 4 °C overnight: TRPV4 (cat. no. SAB2104243; 1:1000; Sigma, St. Louis, MO), IP3R (cat. no. BM5235; 1:500; Boster, Pleasanton, CA), PKC (cat. no. BM0401; 1:500; Boster, Pleasanton, CA), KCNN3 (cat. no. 192515; 1:1000; Abcam, Cambridge, UK), KCNN4 (cat. no. PA5-41015; 1:500; Thermo, Waltham, MA) and GAPDH (cat. no. BA2913; 1:500; Boster, Pleasanton, CA).
Reverse transcription-quantitative polymerase chain reaction
A TRIzol kit (HyClone, Shanghai, China) was used to separate the total RNA. cDNA synthesis was performed using a Prime Script Reverse Transcription Kit (Thermo Fisher Scientific, Karlsruhe, Germany) according to the manufacturer’s instructions. After cDNA synthesis, the samples were amplified and quantified using a Power SYBR Green PCR Master Mix kit (Roche Ltd., Basel, Switzerland). The sequences of all primers are shown in . The relative expression of target genes was evaluated by the 2–ΔΔCt method using GAPDH as an internal control.
Table 1. Sequence of primers.
Ca2+ concentration detection
Rat CBA smooth muscle cells were isolated using the immunomagnetic bead method as described above. The cells were treated with 2 mL cell culture medium and 5 μg Fluo-3/AM fluorescent probe. After 40 min of incubation, 200 mL of the culture medium was added to the confocal laser dish. A small dish was placed in the dark for 20 min to completely hydrolyse the cell probe. A laser-scanning confocal microscope was used to record fluorescence intensity at an emission wavelength of 526 nm and an excitation wavelength of 488 nm. The mean fluorescence intensity of the smooth muscle cells was detected using the Image-Pro Plus image analysis software.
Flow cytometry
The same grouping and administration were used for the laser-scanning confocal experiments in the rats. All rats were subjected to 20 min of ischaemic and 2 h reperfusion and subsequently sacrificed. Endothelial cells from rat CBA were separated using the immunomagnetic bead method, as described above. The ratio of deionized water to diluted combined buffer was 1:3. The concentration of CBA endothelial cells was regulated to 1 × 106/mL, and 100 μL of cell suspension was placed in a 5 mL flow tube. Annexin V/FITC (5 μL) was then added, mixed and incubated at room temperature for 5 min. For flow detection, 20 μg/mL (10 μL) propidium iodide (PI) solution and 400 μL of PBS were added.
Statistical analysis
Statistical analyses were performed using SPSS 20.0 (SPSS Inc., Chicago, IL). Quantitative data are presented as the mean ± standard deviation (SD). The non-paired t-test was used to compare the two groups. Single-factor analysis of variance (ANOVA) was used to compare multiple groups. Homogeneity of variance was tested using the SNK-q test, and irregularity was assessed using Tamhane’s T2 test.
Results
Hyp attenuates brain injury associated with TRPV4 channel activation in IR rats
First, we examined the effects of Hyp on brain injury under IR conditions. Compared with the sham group, the brains of IR rats showed an increase in the number of necrotic neurons and the breakage and disintegration of nerve fibres. Importantly, this damage was markedly improved by Hyp administration (). Previous studies have reported that the TRPV4 channel exerts neuroprotective effects against ischaemic brain injury. To explore whether hyp-attenuation of IR-induced brain injury is associated with TRPV4 channel activation, the TRPV4-specific antagonist (HC-067047) was administered to IR rats. Interestingly, the beneficial effects of Hyp were partially blocked by the HC-067047 treatment (). Moreover, the results showed that the anti-ischaemic effect of Hyp was partly abrogated by inhibitors of IKCa (TRAM-34) or SKCa (apamin), which are downstream of TRPV4 (). As shown in , Nissl staining showed that the number of neurons was increased in the Hyp-administered rats compared with the IR rats, and this change was reversed by the HC-067047, TRAM-34 or apamin treatment (). These results suggest that Hyp exerts neuroprotective effects against brain damage in IR rats, which is partly associated with the TRPV4-SKCa/IKCa pathway.
Hyp induces endothelium-dependent vasodilatation in the CBA of IR rats
TRPV4 regulates cerebral vascular tone by evoking SKCa and IKCa activation (Jackson Citation2022). Therefore, we aimed to identify whether Hyp is associated with TRPV4-mediated vasodilatation. As shown in , the Hyp treatment significantly evoked vasodilatation (47.93 ± 3.09% vs. 2.99 ± 1.53%, p < 0.01) and hyperpolarization (–8.15 ± 1.87 mV vs. −0.55 ± 0.42 mV, p < 0.01) in the CBA, although this effect was eliminated by a TRPV4-specific antagonist. Importantly, we observed that the effects of Hyp on vascular tone were relieved by removing the endothelium from the CBA, suggesting that endothelial TRPV4 activation participates in Hyp-induced vasodilatation. Moreover, we found that endothelium-dependent vasodilation was not influenced by co-treatment with an eNOS inhibitor (l-NAME) or prostacyclin (PGI2) inhibitor (Indo), indicating that Hyp-mediated vasodilation is likely associated with EDHF production.
Figure 4. Effect of Hyp on dilatation and hyperpolarization in the CBA of rats after cerebral ischaemic–reperfusion. Hyp (mol/L) induced endothelial-dependent dilatation (A) and hyperpolarization (B) in the CBA. The dilatation (C) and hyperpolarization (D) induced by Hyp occurred in an NO- and PGI2-independent manner (n = 3). **p < 0.01 vs. vehicle (–Endo); ##p < 0.01 vs. IR (+Endo); ΔΔp < 0.01 vs. Hyp; ▲▲p < 0.01 vs. Hyp + l-NAME + INDO. +Endo: endothelium-intact;–Endo: endothelium-denudated; vehicle: DMSO solution.
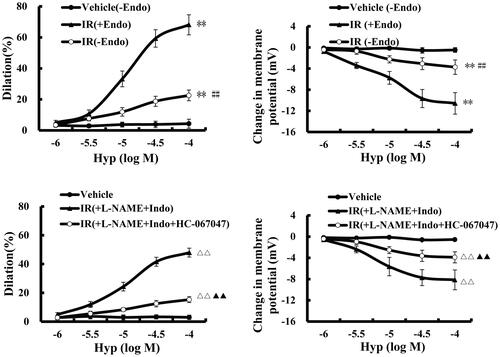
Hyp regulates SKCa and IKCa channels by activating TRPV4 in the CBA of IR rats
Based on the above data, we aimed to identify how Hyp influences TRPV4-mediated vasodilation in the CBA. As shown in , the Hyp treatment significantly increased both the protein and mRNA levels of TRPV4 in CBAs under IR conditions. However, the HC-067047 treatment produced the opposite effect. Moreover, we found that the increased levels of IKCa induced by the Hyp treatment were blocked by the TRAM-34 treatment in the CBA of IR rats (). Moreover, as illustrated in , Hyp administration upregulated both the protein and mRNA levels of SKCa, and these changes were reversed by the apamin treatment. Importantly, we found that HC-067047 abolished the effect of Hyp on the expression of IKCa and SKCa in the CBA of IR rats (). These results indicated that Hyp regulated IKCa and SKCa expression by activating TRPV4 in the CBA of IR rats.
Figure 5. Effect of Hyp on the expression of TRPV4, IKCa and SKCa. The protein and mRNA expression of TRPV4 (A, B), IKCa (C, D) and SKCa (E, F) were detected by western blot and qPCR assays in the CBA of each rat group (n = 3). **p < 0.01 vs. sham; #p < 0.05, ##p < 0.01 vs. IR; &p < 0.05 vs. Hyp; $$p < 0.01 vs. HC-067047, △△p < 0.01 vs. TRAM-34; ▲▲p < 0.01 vs. apamin.
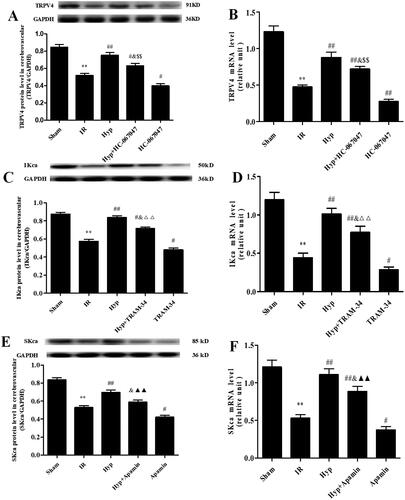
Figure 6. Effect of the TRVP4 antagonist on the expression of IKCa and SKCa. The protein and mRNA expression levels of IKCa (A, B) and SKCa (C, D) in the CBA of each rat group were detected by western blot and qPCR assays (n = 3). **p < 0.01 vs. Sham; #p < 0.05, ##p < 0.01 vs. IR; &p < 0.05, &&p < 0.01 vs. Hyp; $p < 0.05, $$p < 0.01 vs. HC-067047.
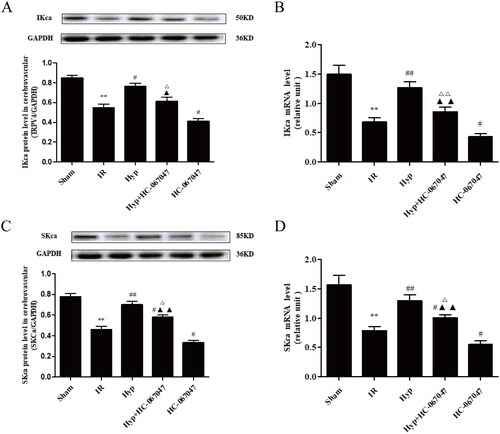
Hyp upregulates TRPV4 expression associated with activating IP3- and PKC-mediated signalling pathways
Previous studies demonstrated that IP3-associated and PKC-mediated signalling pathways contribute to PGI2- and NO-independent vasodilation under different conditions (Yuan et al. Citation2016; Leo et al. Citation2018). Therefore, we examined the effect of Hyp on IP3 and PKC in the brains of IR rats. As shown in , Hyp significantly reduced LDH activity in the serum of IR rats (5053.68 ± 321.51 U/L vs. 8160.04 ± 345.25 U/L, p < 0.01), although this change was reversed by treatment with an IP3R inhibitor (2APB) or an inhibitor of PKC (BisI). Meanwhile, the serum levels of MDA (5.05 ± 0.47 nmol/mL vs. 8.83 ± 0.64 nmol/mL, p < 0.01), NSE (7.22 ± 0.57 ng/mL vs. 11.47 ± 0.92 ng/mL, p < 0.01) and S100β (69.11 ± 5.23 pg/mL vs. 112.24 ± 7.49 pg/mL, p < 0.01) were decreased by Hyp, and these changes were abrogated by co-treatment with 2APB or BisI (). As illustrated in , compared to the IR group, the Hyp treatment markedly increased IP3R and PKC expression in CBA endothelial cells. As anticipated, the IP3R and PKC levels were downregulated by the 2APB or BisI treatment in IR rats under Hyp administration conditions. Importantly, the effect of Hyp on TRPV4 expression was considerably suppressed by the 2APB and BisI treatments (). These results indicate that Hyp upregulates TRPV4 expression by activating the IP3 and PKC signalling pathways.
Figure 7. Effect of the IP3/PKC-Hyp axis on brain injury in serum. ELISA assay was used to detect the effects of Hyp on LDH activity (A), MDA content (B), NSE content (C) and S100β content (D) in the different groups (n = 6). **p < 0.01 vs. sham; #p < 0.05, ##p < 0.01 vs. IR; Δp < 0.05, ΔΔp < 0.01 vs. Hyp; ▲p < 0.05 vs. Hyp + BisI + 2APB.
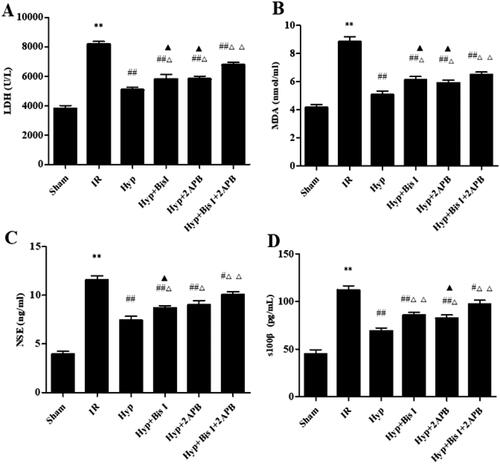
Figure 8. Hyp upregulates TRPV4 expression associated with activating IP3- and PKC-mediated signalling pathways. (A) Increased effect of Hyp on the expression of the PKC protein was obviously attenuated by the PKC specific blocker BisI. (B) Upregulated effect of Hyp on the protein level of IP3R was significantly inhibited by the IP3 receptor blocker 2APB. (C) Pharmacologic inhibition of IP3 and/or PKC partially ablated the upregulation of TRPV4 expression elicited by Hyp in endothelial cells from CBAs (n = 3). **p < 0.01 vs. sham; #p < 0.05, ##p < 0.01 vs. IR; Δp < 0.05 vs. Hyp; ▲p < 0.05, ▲▲p < 0.01 vs. BisI + 2APB.
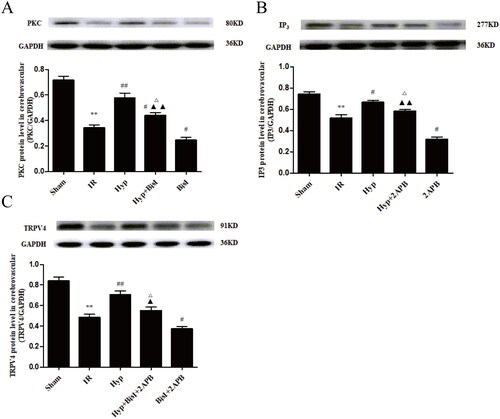
Hyp reduces Ca2+ concentrations and cell apoptosis by activating IP3- and PKC-mediated signalling pathways
Based on these results, we further examined the effect of Hyp on the Ca2+ concentration and endothelial cell apoptosis and elucidated the underlying regulatory mechanisms. As shown in , the increased Ca2+ concentration in IR rats was reduced by the Hyp treatment (49.08 ± 7.74% vs. 83.52 ± 6.93%, p < 0.01). However, this change was blocked by the co-treatment with 2APB and BisI. Meanwhile, we found that IR increased endothelial cell apoptosis in the CBA compared with that of the sham group, which was significantly reversed by Hyp administration (11.27 ± 1.89% vs. 23.44 ± 2.19%, p < 0.01). Interestingly, the 2APB or BisI treatment increased the apoptotic rate of CBA endothelial cells (). These results demonstrated that Hyp may suppress the Ca2+ concentration in smooth muscle cells and inhibit endothelial cell apoptosis by activating IP3- and PKC-mediated signalling pathways in IR rats.
Figure 9. Hyp decreased the Ca2+ concentration in the basilar artery smooth muscle cells of IR rats. Immunofluorescence imaging indicated that the decreased intensity of Ca2+ by Hyp could be reversed by the IP3 receptor blocker 2APB or PKC blocker BisI. (A) Sham, (B) IR, (C) Hyp, (D) HYP + BisI, (E) Hyp + 2APB and (F) Hyp + BisI + 2APB (n = 3). **p < 0.01 vs. sham; #p < 0.05, ##p < 0.01 vs. IR; ΔΔp < 0.01 vs. Hyp; ▲p < 0.05 vs. Hyp + BisI + 2APB. Scale bar = 20 μM.
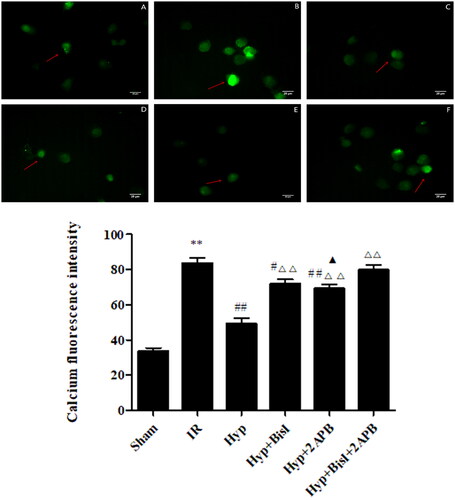
Figure 10. Effects of Hyp on the apoptosis of endothelial cells from the CBA. Apoptosis was analysed by flow cytometry following Annexin V-FITC/PI (Annexin V conjugated to green-fluorescent FITC dye and propidium iodide) double-staining. (A) Sham, (B) IR, (C) Hyp, (D) HYP + BisI, (E) Hyp + 2APB and (F) Hyp + BisI + 2APB. (G) Percentage of apoptotic cells is indicated (n = 6). **p < 0.01 vs. sham; ##p < 0.01 vs. IR; Δp < 0.05, ΔΔp < 0.01 vs. Hyp; ▲p < 0.05, ▲▲p < 0.01 vs. Hyp + BisI + 2APB.
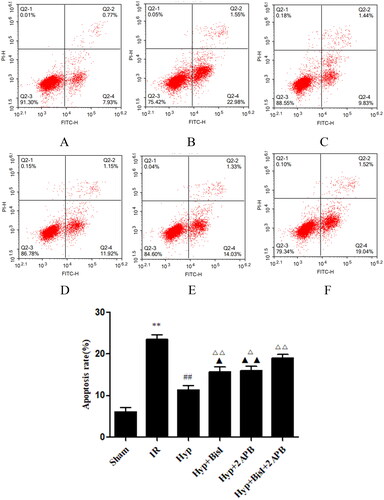
Discussion
In the present study, we observed that Hyp protects against brain injury induced by cerebral IR. We also showed that Hyp exerted a neuroprotective effect by inducing endothelium-dependent vasodilation and hyperpolarization at the level of cell biology. Meanwhile, we found that Hyp-mediated vasodilation and hyperpolarization are likely associated with activation of the TRPV4 channel through the upregulation of IP3R and PKC expression at the molecular level.
Ischaemic stroke accounts for approximately 70% of all strokes worldwide (GBD 2016 Lifetime Risk of Stroke Collaborators Citation2018). In 2019, the incidence of stroke was 100 million cases globally (GBD 2019 Stroke Collaborators Citation2021). Unfortunately, approximately seven million deaths occurred due to ischaemic stroke (Jeon et al. Citation2020). Ischaemic stroke results from a disruption of blood flow to the brain due to vessel occlusion or stenosis. Thus, the improvement of cerebral blood flow is critical and beneficial for the treatment of ischaemic stroke (Fan et al. Citation2022). Previous studies have demonstrated that vasodilation responses contribute to increased brain blood flow, promote cerebral collateral recruitment, and reduce infarct size during ischaemic stroke (Gregori-Pla et al. Citation2019; Nizari et al. Citation2021). Vasodilation of blood vessels is mainly associated with endothelial function through the release of vasoactive factors, such as EDHF, NO and PGI2 (Bohlen et al. Citation2009; Godo et al. Citation2021). The TRPV4 channel is an osmomechanosensitive channel that is widely expressed in most tissues, including the brain, heart, liver and vasculature (Boudaka and Tominaga Citation2022). Previous studies have demonstrated that TRPV4 is a new target for the treatment of cerebrovascular disease via distinct pathways (Baratchi et al. Citation2016). For example, TRPV4 is involved in a wide range of physiological functions, such as cell proliferation, survival, differentiation, migration and adhesion (Zaninetti et al. Citation2011; Hatano et al. Citation2013). TRPV4 activation ameliorates cerebral ischaemic by reducing neuronal injury and regulating angiogenesis and neurogenesis (Chen et al. Citation2018). Therefore, exploring effective drugs that influence TRPV4-mediated vasodilation is necessary to protect against ischaemic stroke.
The total flavones of Rhododendron (TFR) represent the effective parts separated from the flowers of Rhododendron simsii Planch (Wu et al. Citation2019). Hyp is an active ingredient of TFR that significantly improves recovery from cerebral IR injury (Shi et al. Citation2019). Our results showed that Hyp treatment ameliorated IR-induced brain injury, as evidenced by a decrease in the number of damaged neurons and an increase in the level of TRPV4 compared with IR rats. Importantly, the beneficial effect of Hyp was suppressed by HC-067047 administration, which is a selective inhibitor of the TRPV4 channel. The activation of TRPV4 is important for regulating endothelium-dependent vasodilation by enhancing Ca2+ influx through SKCa and IKCa channel activation (Poole et al. Citation2013). Interestingly, we also found that Hyp evokes vasodilatation and hyperpolarization in the CBA of IR rats and that its action was blocked by HC-067047 treatment. As expected, we also found that Hyp regulated SKCa and IKCa channels by activating TRPV4. Moreover, we observed that the effect of Hyp on neuronal injury and the upregulation of IKCa and SKCa were abrogated by inhibitors of IKCa (TRAM-34) or SKCa (apamin) in the CBA of IR. These results demonstrated that Hyp exerts a neuroprotective effect by activating TRPV4-mediated vasodilation.
Accumulating evidence has shown that TRPV4 activation influences vascular dilation by inducing the production of EDHF, NO or PGI2 (Liu et al. Citation2021). Similar to previous studies, we also found that Hyp-induced vasodilatation is dependent on EDHF production in an NO- and PGI2-independent manner in endothelial cells from the CBA of IR rats. To further investigate the mechanism by which Hyp affects TRPV4 expression, we focused on IP3 and PKC. IP3-associated and PKC-mediated signalling pathways play a critical role in inducing PGI2- and NO-independent vasodilation. IP3 is an important second messenger that binds to inositol triphosphate receptors on the sarcoplasmic reticulum to cause Ca2+ release and an increase in intracellular Ca2+ concentration (Ivanova et al. Citation2017). Studies have shown that IP3 activation promotes the opening of TRPV4 channels (Heathcote et al. Citation2019). PKC induces vasodilation through the EDHF mechanism by activating TRPV4, which plays an important role in regulating vasomotor function (Sonkusare et al. Citation2014). The results herein showed that the expression of IP3R and PKC was markedly increased by the Hyp treatment, and this effect was reduced by treatment with an IP3R inhibitor (2APB) or an inhibitor of PKC (BisI). Importantly, the effect of Hyp on TRPV4 expression was considerably suppressed by the 2APB and BisI treatment, suggesting that Hyp upregulates TRPV4 expression through the activation of the IP3 and PKC signalling pathways.
Taken together, our results demonstrated that Hyp protects against IR-induced brain injury by inducing TRPV4-mediated vasodilation through the upregulation of IP3R and PKC expression, suggesting that Hyp may be used to develop effective drugs for the treatment of ischaemic stroke.
Conclusions
Hyp can induce both endothelium-dependent and -independent vasodilatation and hyperpolarization in the CBA of IR animals. The protective effect of Hyp against cerebral ischaemic injury may be associated with CBA vasodilatation and hyperpolarization triggered by the upregulated expression of IP3R, PKC, IKCa, SKCa and TRPV4 in the CBA vascular endothelium (). In addition, the therapeutic role of Hyp was related to the activation of the IP3/PKC signalling pathway, thus leading to TRPV4 channel opening and Ca2+ influx blockage in the vascular smooth muscle cells of the CBA, which then leads to a decreased apoptotic rate of the CBA endothelial cells during cerebral IR injury. Therefore, the current findings establish the therapeutic potential of the traditional Chinese medicine agent Hyp in the treatment of brain damage. Although our Hyp has shown some protective effect in the treatment of ischaemic stroke in animal experiments, more clinical trial certification is needed due to the difference between animals and humans. In addition, based on the deficiency of rt-PA, Hyp is also expected to become an auxiliary drug for rt-PA.
Figure 11. Schematic diagram of the protective mechanism of hypericin on ischaemic brain injury. Hyperin treatment can increase the expression of IP3/PKC, thereby promoting the opening of the TRPV4 channel in endothelial cells, which stimulates endothelium-dependent vasodilation and ameliorates ischaemic brain injury by evoking IKCa/SKCa.
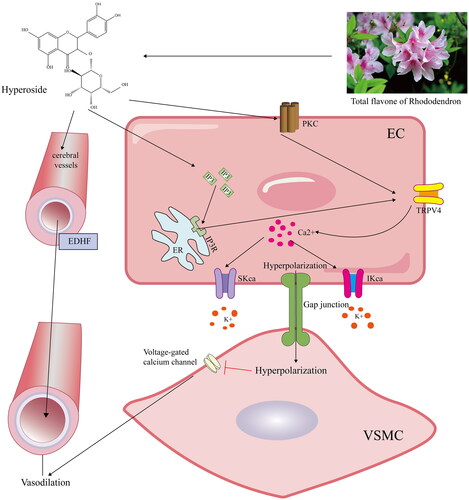
Author contributions
Jun Han designed the study and drafted the manuscript. Lei Shi and Chenchen Jiang coordinated the experiments. Yuxiang He, Hanghang Xu, Xuebin Shen and Jiajun Lu conducted the animal studies. Xiuyun Yin participated in the data analyses. Zhuo Chen, Di Cao and Xuefeng Hou were involved in the discussion of the experiments and drafts of the manuscript. All authors have read and approved the final manuscript. All data were generated in-house and no paper mill was used. All authors agree to be accountable for all aspects of the work, thus ensuring its integrity and accuracy.
Disclosure statement
No potential conflict of interest was reported by the author(s).
Data availability statement
All data generated or analysed during this study are included in the manuscript.
Additional information
Funding
References
- Bagher P, Garland CJ. 2014. Scaffolding builds to reduce blood pressure. Sci Signal. 7(333):pe16. doi: 10.1126/scisignal.2005527.
- Baratchi S, Almazi JG, Darby W, Tovar-Lopez FJ, Mitchell A, McIntyre P. 2016. Shear stress mediates exocytosis of functional TRPV4 channels in endothelial cells. Cell Mol Life Sci. 73(3):649–666. doi: 10.1007/s00018-015-2018-8.
- Bohlen HG, Zhou X, Unthank JL, Miller SJ, Bills R. 2009. Transfer of nitric oxide by blood from upstream to downstream resistance vessels causes microvascular dilation. Am J Physiol Heart Circ Physiol. 297(4):H1337–H1346. doi: 10.1152/ajpheart.00171.2009.
- Boudaka A, Tominaga M. 2022. Physiological and pathological significance of esophageal TRP channels: special focus on TRPV4 in esophageal epithelial cells. Int J Mol Sci. 23(9):4550. doi: 10.3390/ijms23094550.
- Chen CK, Hsu PY, Wang TM, Miao ZF, Lin RT, Juo SH. 2018. TRPV4 activation contributes functional recovery from ischemic stroke via angiogenesis and neurogenesis. Mol Neurobiol. 55(5):4127–4135. doi: 10.1007/s12035-017-0625-0.
- Fan JL, Brassard P, Rickards CA, Nogueira RC, Nasr N, McBryde FD, Fisher JP, Tzeng YC. 2022. Integrative cerebral blood flow regulation in ischemic stroke. J Cereb Blood Flow Metab. 42(3):387–403. doi: 10.1177/0271678X211032029.
- GBD 2016 Lifetime Risk of Stroke Collaborators. 2018. Global, regional, and country-specific lifetime risks of stroke, 1990 and 2016. N Engl J Med. 379:2429–2437. doi: 10.1056/NEJMoa1804492.
- GBD 2019 Stroke Collaborators. 2021. Global, regional, and national burden of stroke and its risk factors, 1990–2019: a systematic analysis for the Global Burden of Disease Study 2019. Lancet Neurol. 20(10):795–820. doi: 10.1016/S1474-4422(21)00252-0.
- Godo S, Takahashi J, Yasuda S, Shimokawa H. 2021. Endothelium in coronary macrovascular and microvascular diseases. J Cardiovasc Pharmacol. 78(Suppl. 6):S19–S29. doi: 10.1097/FJC.0000000000001089.
- Goto K, Ohtsubo T, Kitazono T. 2018. Endothelium-dependent hyperpolarization (EDH) in hypertension: the role of endothelial ion channels. Int J Mol Sci. 19(1):315. doi: 10.3390/ijms19010315.
- Gregori-Pla C, Blanco I, Camps-Renom P, Zirak P, Serra I, Cotta G, Maruccia F, Prats-Sanchez L, Martinez-Domeno A, Busch DR, et al. 2019. Early microvascular cerebral blood flow response to head-of-bed elevation is related to outcome in acute ischemic stroke. J Neurol. 266(4):990–997. doi: 10.1007/s00415-019-09226-y.
- Han J, Chen ZW, He GW. 2013. Acetylcholine and sodium hydrosulfide-induced endothelium-dependent relaxation and hyperpolarization in cerebral vessels of global cerebral ischemic–reperfusion rat. J Pharmacol Sci. 121(4):318–326. doi: 10.1254/jphs.12277fp.
- Han J, He GW, Chen ZW. 2014. Protective effect and mechanism of total flavones from Rhododendron simsii Planch on endothelium-dependent dilatation and hyperpolarization in cerebral ischemic–reperfusion and correlation to hydrogen sulphide release in rats. Evid Based Complement Alternat Med. 2014:904019. doi: 10.1155/2014/904019.
- Hao Y, Qi Z, Ding Y, Yu X, Pang L, Zhao T. 2019. Effect of interventional therapy on IL-1β, IL-6, and neutrophil–lymphocyte ratio (NLR) levels and outcomes in patients with ischemic cerebrovascular disease. Med Sci Monit. 25:610–617. doi: 10.12659/MSM.912064.
- Hatano N, Suzuki H, Itoh Y, Muraki K. 2013. TRPV4 partially participates in proliferation of human brain capillary endothelial cells. Life Sci. 92(4–5):317–324. doi: 10.1016/j.lfs.2013.01.002.
- Heathcote HR, Lee MD, Zhang X, Saunter CD, Wilson C, McCarron JG. 2019. Endothelial TRPV4 channels modulate vascular tone by Ca2+-induced Ca2+ release at inositol 1,4,5-trisphosphate receptors. Br J Pharmacol. 176(17):3297–3317. doi: 10.1111/bph.14762.
- Ivanova H, Luyten T, Decrock E, Vervliet T, Leybaert L, Parys JB, Bultynck G. 2017. The BH4 domain of Bcl-2 orthologues from different classes of vertebrates can act as an evolutionary conserved inhibitor of IP(3) receptor channels. Cell Calcium. 62:41–46. doi: 10.1016/j.ceca.2017.01.010.
- Jackson WF. 2022. Endothelial ion channels and cell–cell communication in the microcirculation. Front Physiol. 13:805149. doi: 10.3389/fphys.2022.805149.
- Jeon J, Lourenco J, Kaiser EE, Waters ES, Scheulin KM, Fang X, Kinder HA, Platt SR, Rothrock MJ Jr, Callaway TR, et al. 2020. Dynamic changes in the gut microbiome at the acute stage of ischemic stroke in a pig model. Front Neurosci. 14:587986. doi: 10.3389/fnins.2020.587986.
- Leo MD, Zhai X, Yin W, Jaggar JH. 2018. Impaired trafficking of β1 subunits inhibits BK channels in cerebral arteries of hypertensive rats. Hypertension. 72(3):765–775. doi: 10.1161/HYPERTENSIONAHA.118.11147.
- Liu L, Guo M, Lv X, Wang Z, Yang J, Li Y, Yu F, Wen X, Feng L, Zhou T. 2021. Role of transient receptor potential vanilloid 4 in vascular function. Front Mol Biosci. 8:677661. doi: 10.3389/fmolb.2021.677661.
- Lv L, Xi HP, Huang JC, Zhou XY. 2021. LncRNA SNHG1 alleviated apoptosis and inflammation during ischemic stroke by targeting miR-376a and modulating CBS/H2S pathway. Int J Neurosci. 131(12):1162–1172. doi: 10.1080/00207454.2020.1782904.
- Nizari S, Basalay M, Chapman P, Korte N, Korsak A, Christie IN, Theparambil SM, Davidson SM, Reimann F, Trapp S, et al. 2021. Glucagon-like peptide-1 (GLP-1) receptor activation dilates cerebral arterioles, increases cerebral blood flow, and mediates remote (pre)conditioning neuroprotection against ischaemic stroke. Basic Res Cardiol. 116(1):32–44. doi: 10.1007/s00395-021-00873-9.
- Ohtsuki S, Yamaguchi H, Asashima T, Terasaki T. 2007. Establishing a method to isolate rat brain capillary endothelial cells by magnetic cell sorting and dominant mRNA expression of multidrug resistance-associated protein 1 and 4 in highly purified rat brain capillary endothelial cells. Pharm Res. 24(4):688–694. doi: 10.1007/s11095-006-9188-x.
- Pan XW, Wang MJ, Gong SS, Sun MH, Wang Y, Zhang YY, Li F, Yu BY, Kou JP. 2020. YiQiFuMai lyophilized injection ameliorates tPA-induced hemorrhagic transformation by inhibiting cytoskeletal rearrangement associated with ROCK1 and NF-κB signaling pathways. J Ethnopharmacol. 262:113161. doi: 10.1016/j.jep.2020.113161.
- Petersen CCH. 2017. Whole-cell recording of neuronal membrane potential during behavior. Neuron. 95(6):1266–1281. doi: 10.1016/j.neuron.2017.06.049.
- Poole DP, Amadesi S, Veldhuis NA, Abogadie FC, Lieu T, Darby W, Liedtke W, Lew MJ, McIntyre P, Bunnett NW. 2013. Protease-activated receptor 2 (PAR2) protein and transient receptor potential vanilloid 4 (TRPV4) protein coupling is required for sustained inflammatory signaling. J Biol Chem. 288(8):5790–5802. doi: 10.1074/jbc.M112.438184.
- Radu RA, Terecoasă EO, Băjenaru OA, Tiu C. 2017. Etiologic classification of ischemic stroke: where do we stand? Clin Neurol Neurosurg. 159:93–106. doi: 10.1016/j.clineuro.2017.05.019.
- Rakers C, Schmid M, Petzold GC. 2017. TRPV4 channels contribute to calcium transients in astrocytes and neurons during peri-infarct depolarizations in a stroke model. Glia. 65(9):1550–1561. doi: 10.1002/glia.23183.
- Seki T, Goto K, Kiyohara K, Kansui Y, Murakami N, Haga Y, Ohtsubo T, Matsumura K, Kitazono T. 2017. Downregulation of endothelial transient receptor potential vanilloid type 4 channel and small-conductance of Ca2+-activated K+ channels underpins impaired endothelium-dependent hyperpolarization in hypertension. Hypertension. 69(1):143–153. doi: 10.1161/HYPERTENSIONAHA.116.07110.
- Shi Y, Qiu X, Dai M, Zhang X, Jin G. 2019. Hyperoside attenuates hepatic ischemic–reperfusion injury by suppressing oxidative stress and inhibiting apoptosis in rats. Transplant Proc. 51(6):2051–2059. doi: 10.1016/j.transproceed.2019.04.066.
- Sonkusare SK, Bonev AD, Ledoux J, Liedtke W, Kotlikoff MI, Heppner TJ, Hill-Eubanks DC, Nelson MT. 2012. Elementary Ca2+ signals through endothelial TRPV4 channels regulate vascular function. Science. 336(6081):597–601. doi: 10.1126/science.1216283.
- Sonkusare SK, Dalsgaard T, Bonev AD, Hill-Eubanks DC, Kotlikoff MI, Scott JD, Santana LF, Nelson MT. 2014. AKAP150-dependent cooperative TRPV4 channel gating is central to endothelium-dependent vasodilation and is disrupted in hypertension. Sci Signal. 7(333):ra66. doi: 10.1126/scisignal.2005052.
- Wang Y, Liu S, Wang R, Shi L, Liu Z, Liu Z. 2020. Study on the therapeutic material basis and effect of Acanthopanax senticosus (Rupr. et Maxim.) harms leaves in the treatment of ischemic stroke by PK-PD analysis based on online microdialysis-LC–MS/MS method. Food Funct. 11(3):2005–2016. doi: 10.1039/c9fo02475a.
- Wu L, Li Q, Liu S, An X, Huang Z, Zhang B, Yuan Y, Xing C. 2019. Protective effect of hyperoside against renal ischemic–reperfusion injury via modulating mitochondrial fission, oxidative stress, and apoptosis. Free Radic Res. 53(7):727–736. doi: 10.1080/10715762.2019.1623883.
- Xiao R, Xiang AL, Pang HB, Liu KQ. 2017. Hyperoside protects against hypoxia/reoxygenation induced injury in cardiomyocytes by suppressing the Bnip3 expression. Gene. 629:86–91. doi: 10.1016/j.gene.2017.07.063.
- Yang Y, Gao H, Liu W, Liu X, Jiang X, Li X, Wu Q, Xu Z, Zhao Q. 2021. Arctium lappa L. roots ameliorates cerebral ischemic through inhibiting neuronal apoptosis and suppressing AMPK/mTOR-mediated autophagy. Phytomedicine. 85:153526. doi: 10.1016/j.phymed.2021.153526.
- Yuan Q, Yang J, Santulli G, Reiken SR, Wronska A, Kim MM, Osborne BW, Lacampagne A, Yin Y, Marks AR. 2016. Maintenance of normal blood pressure is dependent on IP3R1-mediated regulation of eNOS. Proc Natl Acad Sci U S A. 113(30):8532–8537. doi: 10.1073/pnas.1608859113.
- Zaninetti R, Fornarelli A, Ciarletta M, Lim D, Caldarelli A, Pirali T, Cariboni A, Owsianik G, Nilius B, Canonico PL, et al. 2011. Activation of TRPV4 channels reduces migration of immortalized neuroendocrine cells. J Neurochem. 116(4):606–615. doi: 10.1111/j.1471-4159.2010.07144.x.
- Zhang L, Dong L-Y, Li Y-J, Hong Z, Wei W-S. 2012. The microRNA miR-181c controls microglia-mediated neuronal apoptosis by suppressing tumor necrosis factor. J Neuroinflammation. 9:211. doi: 10.1186/1742-2094-9-211.