Abstract
Context
Diabetic nephropathy (DN) is the main cause of end-stage renal disease. Modified Shen-Yan-Fang-Shuai formula (M-SYFSF) has excellent clinical efficacy in treating diabetic kidney disease. However, the potential mechanism of M-SYFSF remains unknown.
Objective
To investigate the mechanism of M-SYFSF against DN by network pharmacological analysis and biological experiments.
Materials and methods
Utilizing a web-based pharmacology database, the potential mechanisms of M-SYFSF against DN were identified. In vivo experiments, male SD rats were injected with streptozotocin (50 mg/kg) and got uninephrectomy to construct a model of DN. M-SYFSF (11.34 g/kg/d) was gavaged once per day for 12 weeks after model establishment. In vitro experiments, human proximal tubular cells (HK-2) were performed with advanced glycation end-products (AGEs) (100 μg/mL), then intervened with M-SYFSF freeze-dried powder. Pathological staining, WB, IHC, ELISA were conducted to explore the mechanism of M-SYFSF against DN.
Results
Network pharmacological analysis showed that MAPK pathway was the potential pathway. Results showed that compared with the Model group, M-SYFSF significantly reduced 24h urine albumin, UACR, and serum creatinine levels (54.90 ± 26.67 vs. 111.78 ± 4.28, 8.87 ± 1.69 vs. 53.94 ± 16.01, 11.56 ± 1.70 vs. 118.70 ± 49.57, respectively), and improved renal pathological changes. Furthermore, the intervention of M-SYFSF reduced the expression of pro-inflammatory cytokines and inhibited the activation of MAPK pathway in AGEs-treated HK-2 cells.
Discussion and conclusion
M-SYFSF is likely to reduce inflammation in DN by inhibiting the MAPK pathway. It provides a theoretical basis for the clinical application of M-SYFSF in the treatment of DN.
Introduction
Diabetic nephropathy (DN), a microvascular complication causing damage to the kidneys (Forbes and Cooper Citation2013), develops in 20–40% of individuals diagnosed with diabetes mellitus (Roy et al. Citation2020). The risk of diabetic kidney disease is increasing as the number of diabetic patients globally exceeds 537 million by 2021 (International Diabetes Federation [IDF] Citation2021), and the incidence of diabetes-related end-stage renal disease (ESRD) in China rises (Cheng et al. Citation2021; Lin et al. Citation2021). DN is a major cause of ESRD worldwide (Doshi and Friedman Citation2017). Therefore, modern science and future medicine face challenges in identifying new therapeutic options to prevent and treat DN. The etiology of DN is complex, involving several factors, including genetic susceptibility, hyperglycemia, hyperlipidemia, hypertension, altered renal hemodynamics and vasoactive substance metabolism (Warren et al. Citation2019; Wang et al. Citation2021). In the past decade, traditional Chinese medicine (TCM) for the treatment of DN has been recognized for its multi-channel and multi-target mechanisms of action (Lu et al. Citation2019; Guo et al. Citation2021).
In China, M-SYFSF is a modified version of SYFSF, which has been used as a traditional medicine formula historically, to treat individuals with diabetic kidney disease (Yao et al. Citation2019). When it is used with medicines that decrease blood glucose, M-SYFSF may make benefit to diabetic kidney disease patients with proteinuria reduction and renal function protection. M-SYFSF consists of seven herbs: Astragalus mongholicus Bunge (Fabaceae) [Astragali radix] (Huang Qi), Angelica sinensis (Oliv.) Diels (Apiaceae) [Angelicae sinensis radix] (Dang Gui), Sargassum pallidum (Turn.) C.Ag. (Sargassaceae) [Sargassum] (Hai Zao), Trionyx sinensis Wiegmann (Trionychidae) [Trionycis carapax] (Bie Jia), Ostrea gigas Thunberg (Ostreidae) [Ostreae concha] (Mu Li), Panax notoginseng (Burk.) F. H. Chen (Araliaceae) [Notoginseng radix et rhizoma] (San Qi), and Rehmannia glutinosa Libosch. (Scrophulariaceae) [Rehmanniae radix praeparata] (Shu Dihuang) in a ratio of 30:10:10:10:15:3:30. After pattern differentiation and treatment, SYFSF can delay the time to the first dialysis for non-dialysis patients with chronic kidney disease stage 5 and reduce the incidence of dialysis events in chronic kidney disease stage 5 patients (Ma et al. Citation2020).
Chronic inflammation and fibrosis are crucial factors affecting the development of diabetic microvascular complications (Barutta et al. Citation2015; Sharma et al. Citation2017, Citation2018, Citation2019). In response to stimulation by persistent hyperglycemia, advanced glycation end-products (AGEs) or multiple inflammatory mediators, proteinuria presents a continuous upregulation (Navarro et al. Citation2006), which accelerates the process of tubular fibrosis and aggravating renal injury in DN (Hu et al. Citation2015; Ranganathan et al. Citation2013). Our previous study indicated that SYFSF reduced the inflammatory response by acting on the tumour necrosis factor-alpha/nuclear factor kappa B (TNF-α/NF-κB) pathway (Lv et al. Citation2017), thereby it reduced kidney damage caused by diabetes. However, the mechanism by which M-SYFSF acts on reducing inflammation and delaying the progression of DN, especially on renal tubules, remains unclear and needs to be further improved.
Network pharmacological analysis is a newly proposed strategy in TCM research (Wang et al. Citation2021). It is an effective approach for linking the active compounds of TCM, its corresponding targets and disease target genes using complex networks, which allows an in-depth analysis of its underlying mechanism (Ouyang et al. Citation2021; Wei et al. Citation2021). To elucidate the underlying inflammatory mechanism by which M-SYFSF acts on DN, our research team employed a network pharmacological analysis method combined with in vivo and in vitro experimental validation.
Materials and methods
Network pharmacological analysis
Bioactive compounds and prediction of corresponding targets of M-SYFSF
The traditional Chinese medicine systems pharmacology database and analysis platform (TCMSP (Ru et al. Citation2014; Wang et al. Citation2022), https://tcmspw.com/tcmsp.php/) and SymMap (http://www.symmap.org/) (Wu et al. Citation2019) was employed to verify the main chemical compounds of these herbs in M-SYFSF, as well as the protein targets associated with these compounds. We selected the major M-SYFSF chemical compounds with established criteria consisting of oral bioavailability (OB) ≥ 30% and drug-like (DL) ≥ 0.18. After obtaining the simplified molecular input line entry system (SMILES) structures of the active compounds of Bie Jia and Mu Li, the gene targets on which the active ingredients act in vivo were obtained on the similarity ensemble approach (SEA, http://sea.bkslab.org) platform. Further, we used UniProt (http://www.uniprot.org) to normalize the protein targets, including gene identity and name, and to remove genes of non-human origin.
Prediction of the targets of kidney disease
We employed the recently updated GeneCards (https://www.genecards.org/) to generate disease genes using the keywords “Diabetic Nephropathy” and “Diabetic Kidney Disease”.
Construction and analysis of the interaction network
We built a protein-protein interaction (PPI) network of disease-drug intersection targets using the highly cited human PPI database (STRING) (https://string-db.org). The protein species were set to “Homo sapiens”. The confidence level was set to “medium confidence (0.400)”. To determine the compounds and core targets of M-SYFSF in the treatment of DN, we imported the data into Cytoscape (version 3.8.2). We then performed a topological analysis of the network and employed a node degree value that exceeded the average value to identify core targets and compounds.
Network construction and enrichment analysis
To predict the biological functions and pathways of M-SYFSF targeting proteins in the treatment of DN, we utilized the OmicShare platform (http://omicshare.com/tools/) and the database for annotation, visualization and integrated discovery (DAVID, https://david.ncifcrf.gov/) to conduct GO and KEGG pathway enrichment analysis. The significantly enriched biological processes (BP), molecular functions (MF), cellular compounds (CC), and pathways were visualized as bubble maps.
M-SYFSF drug composition and preparation
Preparation of M-SYFSF
A mixture of Huang Qi (drying), Dang Gui, Hai Zao, Bie Jia (processing with vinegar), Mu Li (drying), San Qi, and Shu Dihuang (doses: 30, 10, 10, 10, 15, 3, 30 g) was purchased from the Dongzhimen Hospital, Beijing University of Chinese Medicine (Beijing, China).
Before decocting the herbs, each of the seven herbs in M-SYFSF was separately soaked in 10 times the volume amount of water for 30 min. Firstly, Bie Jia and Mu Li were decocted for 30 min, after that, other herbs were added and these mixtures were decocted together for 30 min before being filtered to yield extract A. The mixtures were then reconstituted with water, decocted for 30 min, and filtered to obtain extract B. The mixture of liquids A and B was concentrated to 0.8 g/mL herbs concentrate C and frozen for 24 h at −80 °C. Then, the herbs concentrate C was dried and dehydrated to powder form in a freeze dryer at −80 °C, and the powder was packed and kept in a refrigerator at −80 °C.
UHPLC-MS/MS analysis
Preparing of sample solution 10 mg of accurately weighted freeze-dried M-SYFSF powder was immersed into 5 mL of 50% methanol aqueous solution before being ultrasonicated for 25 min. Then the extracted solution was filtered through a 0.22 μm filtration membrane for further UHPLC-MS/MS analysis.
UHPLC-MS/MS analysis conditions
Chemical ingredients analysis was performed in a Vanquish UHPLC system (Thermo Fisher Scientific Inc.) with an electrospray ionization (ESI) interface. An ACQUITY UPLC® HSS (Waters, USA) T3 column (2.1 mm × 100 mm, 1.8 μm) was applied for chromatographic analysis at 40 °C column temperature with a flow rate of 0.2 mL/min. The mobile phase was composed of water containing 0.1% formic acid (v/v, A) and acetonitrile (B), with a gradient elution as follows: 10% B at 0-2 min, 10%-95% B at 2-11 min, 95% B at 11-14 min, 95%-10% B at 14-14.1 min and 10% B at 14.1-17 min. The injection volume was 5 μL.
Mass spectrometric analysis was operated in negative and positive ionization modes and the data collecting as well as analysis were carried out using Xcalibur 4.1 software (Thermo Fisher). The parameters in the source were set as follows: sheath gas (N2) flow rate at 30 Arb, auxiliary gas (N2) flow rate at 10 Arb, probe heater temperature at 350 °C, capillary temperature at 320 °C, the full MS scan range at 100 to 1500 m/z with a resolution of 12,000 and the MS/dd-MS2 with a resolution of 30,000.
Molecular docking analysis
The two-dimensional (2D) structures of the basic compounds were downloaded from the PubChem database (https://pubchem.ncbi.nlm.nih.gov/). Then, Chem3D was employed to process and convert these 2D structures of the identified major compounds into a 3D structure and saved as ligands using the PDBQT format. The 3D structures of the desired compounds were then downloaded from the PDB database (https://www.rcsb.org/). CB-Dock (http://clab.labshare.cn/cb-dock/php/) was employed to dock ligands with target molecules.
In vitro and in vivo experiments
Establishment and treatment of a DN-induced rat model
The experimental animals were 60 male Sprague-Dawley (SD) rats (6-8 weeks old, weight: 180-200 g) obtained from the Beijing Vital River Laboratory Animal Technology Co., Ltd. (SCXK [Beijing] 2012-0001). All the animals were handled in compliance with the criteria established by the Animal Research Committee of Beijing University of Chinese Medicine. The Animal Welfare and Ethics Review Committee of Dongzhimen Hospital, affiliated with Beijing University of Chinese Medicine, approved this experiment ethically (ethics number: 18-19). The experiments were performed at the Barrier Animal Experimental Facility of the Beijing University of Chinese Medicine and were a compound of the Ministry of Education’s Key Laboratory of TCM (SYXK [Beijing] 2015-0001). The rats were held in a barrier-level animal room with controlled temperature (22-24 °C) and humidity (35%-45%), as well as a 12 h light/dark cycle.
The DN model was constructed according to an established protocol (Lohr et al. Citation1991). After a week of adaptation, the rats were randomly assigned to either the sham operation (SO) group or the operation group, 30 rats from each of the operation and SO groups had been treated throughout the medication intervention. Initially, surgical procedures (uninephrectomies) were performed on the operation group of rats to trigger hyperfiltration and hyperperfusion. Based on our team’s previous experience with this DN rat model, One-week post-surgery, the operation group received a single intraperitoneal injection of 50 mg/kg streptozotocin (STZ) (Sigma, USA). Rats that fitted the model criteria (two consecutive random blood glucose levels > 16.7 mmol/L) were randomly assigned to one of two groups: the model group and the model + M-SYFSF group, which was treated with M-SYFSF at a dose of 11.34 g/kg/d(Converting the human clinical dose to the rat dose. The calculation was as follows: for an adult based on a body weight of 60 kg, one dose of the formula weighing 108 g per day, the clinical dose for a human is 1.8 g/kg/d. Based on the dose per unit body weight, the equivalent dose for a rat was equivalent to 6.3 times that of a human and the dose for a rat is 11.34 g/kg/d)(n = 15), or the model group that was treated with saline (3 mL/day; n = 15). The SO group was randomly assigned to one of two groups: the SO + M-SYFSF group, which was treated with M-SYFSF at a dose of 11.34 g/kg/d (n = 15), or the SO group, which received saline 3 mL/day (n = 15).
Urinary albumin testing
Urinary albumin was tested every four weeks. All the rats were placed in metabolic cages and urine was collected for 24 h. The total urine volume was determined, followed by centrifugation of the supernatant at 3,000 rpm for 10 min and then stored at −80 °C. Urinary albumin concentration was determined using a rat albumin quantification kit (Nanjing Jiancheng Institute of Biological Engineering, Nanjing, China). The 24 h urinary albumin was calculated as urinary albumin (mg/mL) × urine volume (mL). Urine microalbumin (umALB) concentration was determined using rat MAU (Microalbuminuria) ELISA Kit (elabscience, Wuhan, China). And rat urine creatinine (uCr) was detected with the creatinine Assay kit (Nanjing Jiancheng Institute of Biological Engineering, Nanjing, China). The Urine Albumin-Creatinine Ratio (UACR) (μg/μmol) was calculated as μmALB (μg/mL)/uCr (μmol/mL).
Pathological examination of the kidney
Right kidney tissue blocks were fixed with 4% paraformaldehyde (PFA). After dehydration by alcohol and xylene gradient, paraffin-embedded blocks were made. The kidney tissue sections (4 mm) were dewaxed in xylene and stained with hematoxylin and eosin (H&E), Masson and Periodic Acid-Schiff (PAS), and later observed and photographed under light microscopy.
Serum creatinine and blood glucose testing
At the end of 12 weeks of interventions, random blood glucose was measured by OneTouch Ultra Glucometer (Johnson & Johnson, Beechwood Park North, Inverness, UK) in the tail-tip blood of each group of rats, and the blood was collected from the abdominal aorta of rats. The blood was centrifuged at 3000 rpm, 4 °C for 15 min, and serum was retained. Blood creatinine was measured using the instructions of the Creatinine Assay Kit (Nanjing Jiancheng Institute of Biological Engineering, Nanjing, China).
Immunohistochemistry staining
Paraffin sections were baked for 1 h, dewaxed each with xylene gradient for 10 min, then hydrated downstream each with ethanol gradient for 2 min. The sections were mixed with endogenous peroxidase inhibitor for 20 min avoiding light and then washed 3 times with PBS. Slides were blocked with goat serum and then incubated with primary antibody (Collagene I, Abcam, Cambridge, UK) at 4 °C overnight. The next morning, secondary antibodies were exposed for 60 min at room temperature and analyzed using light microscopy after 3 min mixed with DAB.
Cell culture
HK-2 cells (ATCC, Manassas, VA, USA) were grown in Dulbecco’s modified essential medium (1:1 DMEM/F12) (Gibco, USA) supplemented with 10% fetal bovine serum under standard growth conditions (Gibco). The culture medium was replenished every two days. DN cell models were constructed by adding approximately 100 μg/mL of non-glycosylated control bovine serum albumin (Co-BSA) or AGE-BSA (2221-10; BioVision, Mountain View, CA, USA) to the cultures and incubating for 48 h.
Referring to the criteria of the study by Heinrich et al. (Citation2020), HK-2 cells were treated with a medium containing 0/100/200/300 μg/mL M-SYFSF for 24 h and 48 h respectively, and the survival rate of each group was measured using the CCK-8 method to screen the optimal concentration and duration of action of M-SYFSF. Further, DN model cells were treated with a medium containing 0/50/100/150/200/250 μg/mL M-SYFSF for 48 h, and the optimal treatment concentration of M-SYFSF on DN model cells was screened using the CCK-8 method to determine the survival rate of each group of cells. The screened concentrations of 100/150/200 μg/mL were used as the therapeutic concentrations of M-SYFSF for subsequent experiments.
Cell viability assay
HK-2 cells were inoculated into 96-well plates at a density of 5 × 104 cells/mL and incubated with fresh medium containing different concentrations of M-SYFSF and AGEs for 48 h after conventional medium for 24 h. After 30 min of action using cell counting kit-8 (CCK8), the detection was performed with a microplate reader at 450 nm.
Western blot analysis
Proteins from each group of kidney tissues and HK-2 cells were separated by electrophoresis and transferred to PVDF membrane. Then the PVDF membranes blocked for 1 h, while later incubated overnight with the following primary antibody: α-SMA, ERK, IL6, and β-actin antibodies (Proteintech, USA); p-P38MAPK and p-ERK antibodies (CST, MA, USA); and P38MAPK, JNK, p-JNK, IL1β, and TNF-α antibodies (Abcam, Cambridge, UK). The following day, secondary antibodies were incubated for 1 h. After washing 3 times with TBST, the reaction was performed with ECL hypersensitive luminescent solution and later photographed on a gel imager for analysis.
Statistical analysis
All data were expressed as the mean ± standard error (SEM). We analyzed our data using SPSS 20.0. One-way ANOVA (LSD or Dunnett’s T3 test) was conducted to assess differences between the groups. Differences with p < 0.05 were considered statistically significant.
Results
Network pharmacological analysis of M-SYFSF for diabetic nephropathy
Following the ADME (absorption, distribution, metabolism, excretion) screening, 29 unique compounds and 357 unique targets were identified (). After identifying the targets and compounds, we proceeded to determine potential M-SYFSF compounds and targets for the treatment of DN. shows that 332 genes were shared by DN-M-SYFSF-related genes. We imported the 332 overlapping genes into the STRING database to investigate their correlations with the targets. A PPI network of overlapping genes between M-SYFSF and DN was constructed based on degree screening (), and PTGS2, AKT1, IL6, TP53, and TNF were identified as core targets (degree > average 33.897).
Figure 1. Compounds found in M-SYFSF and their corresponding targets. (A) Compound-target network of M-SYFSF. (B) Veen diagram of compound targets of M-SYFSF and DN-related targets. (C) Compound-target network of M-SYFSF treating DN.
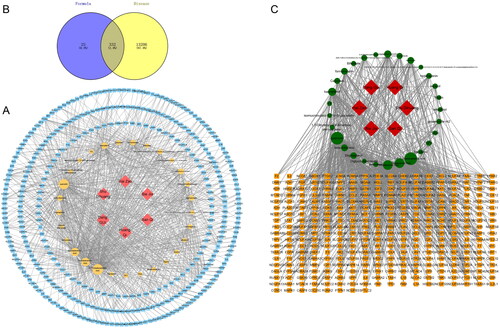
GO and KEGG analyses were performed on overlapping genes to identify the possible mechanisms by which M-SYFSF treats DN (). GO analysis showed that the mode of action of M-SYFSF was mainly related to mitochondrial electron respiratory chain transport, cellular response to drug and stimulus, inflammatory response, oxidation-reduction process, and cell proliferation, metabolism, and death processes.
Figure 2. Overlapped gene-based enrichment analysis: (A-C) gene ontology (GO) enrichment analysis of overlapped genes: (A) cellular compound (CC); (B) biological process (BP) and (C) molecular function (MF); (D) KEGG enrichment analysis of overlapped genes; (E)The MAPK signaling pathway.
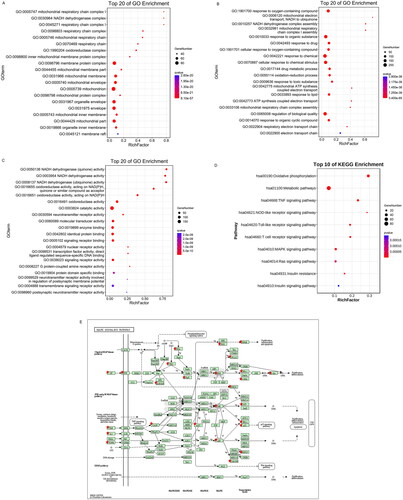
KEGG analysis was performed on overlapping genes to identify the possible mechanisms by which M-SYFSF treats DN. KEGG analysis showed that it was related to oxidative phosphorylation, metabolic pathway, TNF signalling pathway, NOD-like receptor signalling pathway, toll-like receptor signalling pathway, T cell receptor signalling pathway, MAPK signalling pathway, RAS signalling pathway, insulin resistance, insulin signalling pathway, as well as other pathways ().
Screening of bioactive compounds in M-SYFSF by UPLC-ESI-MS/MS analysis
Seven bioactive compounds were identified in the M-SYFSF compound-target network, including quercetin, calycosin, jaranol, 9-octadecenoic acid, 11-decadienoic acid, formononetin, and DIOP ().
Molecular docking of major components to key targets
Potential active compounds in M-SYFSF were analyzed by network pharmacology combined with UPLC-ESI-MS/MS Analysis. The results showed that quercetin, calycosin, and formononetin were the core compounds. The core compounds (quercetin, calycosin, formononetin) and core targets of MAPK signalling pathway (ERK, JNK, P38MAPK) were then selected, and the CB dock platform was employed for molecular docking verification. We observed that the binding energies of the identified active components and their related main targets were all < −5 kcal/mol (, ), indicating strong binding activity.
Table 1. Molecular docking of major compounds to core targets.
Effect of M-SYFSF on renal function and renal injury
As shown in , the blood glucose level of the model group was significantly higher than that of the SO group (Model vs. SO group, 32.95 ± 0.80 vs. 6.76 ± 0.71, p < 0.01); the levels of serum creatinine (Model vs. SO group, 118.70 ± 49.57 vs. 9.03 ± 2.15, p < 0.01), the 24 h urinary albumin and UACR increased (Model vs. SO group, 24 h urinary albumin: 111.78 ± 4.28 vs. 16.00 ± 2.47, UACR: 53.94 ± 16.01 vs. 7.18 ± 2.46; after 12 weeks interventions, p < 0.05), which indicated that the DN rat model was successfully prepared by uninephrectomy + STZ injection.
Figure 5. Effect of M-SYFSF on renal function and renal injury. (A) Blood glucose (mmol/L); (B) Serum creatinine (μmol/L); (C) 24h urinary albumin(mg); (D) Urine albumin-creatinine ratio (UACR) (ug/umol); *p < 0.05, **p < 0.01 compared to the so group; #p < 0.05 compared to the model group; (E) Hematoxylin and eosin (HE) staining; (F) Periodic acid-Schiff (PAS) staining; Magnification: 400×.
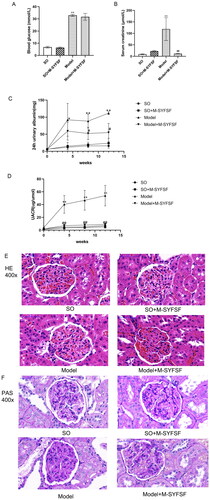
Compared with the model group at the same stage, the serum creatinine in the Model + M-SYFSF group was significantly lower (Model + M-SYFSF vs. Model group, 11.56 ± 1.70 vs. 118.70 ± 49.57, p < 0.05). The 24 h urine albumin and UACR in the Model + M-SYFSF group were substantially lower (Model + M-SYFSF vs. Model group, 24 h urinary albumin: 54.90 ± 26.67 vs. 111.78 ± 4.28, UACR: 8.87 ± 1.69 vs. 53.94 ± 16.01; after 12 weeks M-SYFSF intervention, p < 0.05) than those in the model group at the same stage. Furthermore, compared with the model group, M-SYFSF intervention did not show the effect of lowering blood glucose (M-SYFSF vs. Model group, 31.69 ± 2.82 vs. 32.95 ± 0.80, p > 0.05). It demonstrates that M-SYFSF can slow the advancement of diabetic nephropathy without lowering blood glucose.
showed that the renal glomerular and tubulointerstitial structures of rats in the SO and SO + M-SYFSF groups were normal. In the model group, however, the volume of the glomeruli increased, the capillary loops of the glomerulus were slightly enlarged, the mesangial matrices increased and numerous inflammatory cells infiltrated were filled in the renal interstitium. Compared to the model group, the structure of the basement membrane in the kidney tissue of rats in the Model + M-SYFSF group was normal, the volume of glomeruli was smaller than that of the model group, and the infiltration of renal interstitial inflammatory cells and mesangial matrix were also decreased.
showed that the glomerular structures of the rats in the SO and SO + M-SYFSF groups were relatively complete and there was no mesangial matrix hyperplasia. Compared with the SO group, the renal mesangial area of the model group was significantly wider, the mesangial matrix proliferated and the glomerular basement membrane thickened. Besides, the model + M-SYFSF intervention group showed improved mesangial matrix hyperplasia and decreased basement membrane thickening as compared to the model group.
Effect of M-SYFSF on renal fibrosis
Masson staining showed clear intraglomerular basement membrane thylakoid structure and less basement membrane thylakoid hyperplasia in the renal tissues of SO and SO + M-SYFSF rats. In contrast, glomerular thylakoid stromal hyperplasia was seen in the kidney tissue of the model group, and fibrotic hyperplasia was seen in the renal interstitium (). As shown by immunohistochemistry, compared to the model group, expression of collagen I () was significantly decreased in the kidney tissues of rats in the M-SYFSF, SO and SO + M-SYFSF groups. Additionally, expression of a-SMA was significantly higher in the model group compared to the SO group, while it was significantly lower in the kidney tissue of rats in the model + M-SYFSF group compared to the model group (). However, these results suggested that M-SYFSF is involved in the development of DN via regulating tubulointerstitial fibrosis.
Figure 6. The effect of M-SYFSF on tubulointerstitial fibrosis. (A) Masson staining; (B) Col-I expression as determined by immunohistochemistry staining. Original magnification: 400×. (C) Western blot analysis of α-SMA expression (n = 3). *p < 0.05 compared to the so group; ##p < 0.01 compared to the model group.
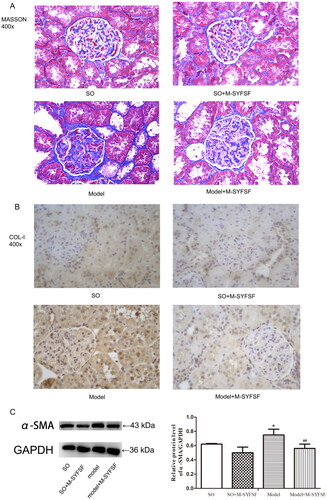
Effect of M-SYFSF on cell viability in AGEs-treated HK 2 cells
CCK-8 results showed that M-SYFSF was not toxic to HK-2 cells in the range of 0-300 μg/mL within 48 h. Considering the construction time of the DN cell model, the optimal duration of action of M-SYFSF was selected as 48 h (. The results of the optimal treatment concentration of M-SYFSF on DN model cells showed that the cell survival rate was highest in the 100/150/200 μg/mL concentration group, and the resulting 100/150/200 μg/mL were screened as the low, medium and high concentrations of M-SYFSF treatment for subsequent experiments ().
Figure 7. The effect of M-SYFSF on cell viability in AGEs-treated HK 2 cells. (A) Screening for optimal concentration and duration of action of M-SYFSF. (B) Optimal therapeutic concentration of M-SYFSF on HK-2 cells treated with AGEs. **p < 0.01 compared to the control group; ##p < 0.01 compared to the model group.
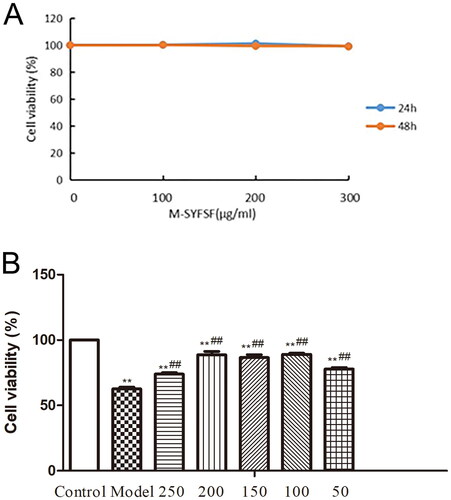
Effect of M-SYFSF on MAPK signalling pathway in HK2 cells
To investigate the potential of M-SYFSF to affect the MAPK signalling pathway in HK2 cells, immunoblot analysis was performed on JNK, P38MAPK, ERK, and phosphorylation pathways (). Compared to the control group, p-JNK, p-P38MAPK, and p-ERK were significantly increased in AGEs-treated HK-2 cells. However, p-JNK, p-P38MAPK, and p-ERK were significantly decreased in AGEs-treated HK-2 cells after certain concentrations of M-SYFSF interventions.
Figure 8. The effect of M-SYFSF on the MAPK signaling pathway in HK-2 cells. (A) Western blot analysis of JNK and p-JNK expression (n = 3); (B) Western blot analysis of P38MAPK and p-P38MAPK expression (n = 3); (C) Western blot analysis of ERK and p-ERK expression (n = 3). *p < 0.05, **p < 0.01 compared to the control group; #p < 0.05, ##p < 0.01 compared to the model group.
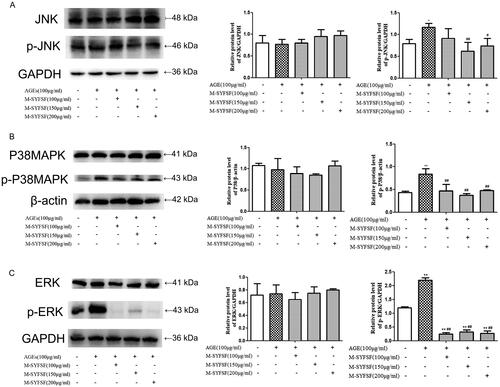
Effect of M-SYFSF on inflammation in HK2 cells
To investigate the effect of M-SYFSF on inflammation in HK2 cells, western blot analysis was performed on IL6, IL1β, and TNF-α (). Compared to the control group, IL6, IL1β, and TNF-α in HK-2 cells were significantly increased after AGEs intervention. However, expression of IL6, IL1β and TNF-α in AGEs-treated HK2 cells was significantly reduced after M-SYFSF interventions.
Discussion
DN is a clinical syndrome characterized by persistent proteinuria with a progressive decline in renal function (Lim Citation2014; Selby and Taa Citation2020). The main pathological manifestations of this syndrome include glomerular lesions, such as diffuse and nodular mesangial expansion, as well as thickening of the glomerular basement membrane (Najafian et al. Citation2011; Maezawa et al. Citation2015), with tubulointerstitial lesions, such as tubular atrophy, tubulointerstitial fibrosis, and interstitial inflammation (Gilbert and Cooper Citation1999; Qi et al. Citation2017). Reducing renal inflammation, urinary protein excretion and pathological renal damage is an important therapeutic objective in DN.
M-SYFSF is a clinically effective formula for treating DN by reducing urinary protein excretion and delaying renal function decline. In this experiment, a DN-induced rat model was constructed using intraperitoneal STZ injections and uninephrectomy. Consistent with previous studies (Wang et al. Citation2019), we observed increased serum creatinine and urine albumin levels and altered renal tissues in the DN model, including increased glomerular volume, basement membrane thickening, diffuse mesangial expansion, increased collagen I and α-SMA expression, and massive inflammatory cell infiltration and fibrous hyperplasia in the renal interstitium. After M-SYFSF treatment, the levels of serum creatinine and the 24 h urinary albumin were significantly reduced, and renal pathological damage was notably improved. However, the underlying mechanism of action of these formulas remains unclear. Therefore, this study employed a network pharmacological analysis approach that combined drug-like screening, network analysis, target prediction, and experimental verification to identify M-SYFSF potential targets and the material basis for the treatment of DN.
As a result of the screening, the potential active compounds in M-SYFSF were analyzed by network pharmacological analysis combined with UPLC-ESI-MS/MS analysis. The results showed that quercetin, calycosin, and formononetin were the core compounds; some of which had been shown to alleviate pathological renal damage in STZ-induced animal models through multiple pathways. Therefore, it is likely that the active compounds of M-SYFSF in DN treatment are quercetin, calycosin, and formononetin. However, this study only provides theoretical evidence and further experimental validation is needed to draw definitive conclusions.
Network pharmacological analysis indicated that M-SYFSF affects DN progression, probably via the MAPK signalling pathway, to reduce the inflammatory response. The role of the screened core targets PTGS2, AKT1, IL6, and TNF in DN is also closely related to the MAPK signalling pathway (Gratton et al. Citation2001; Honma et al. Citation2014; Malik et al. Citation2017). The MAPK signalling pathway is an important eukaryotic signal transduction network comprising three classical subfamilies including ERK, P38 MAPK, and JNK, which are involved in numerous aspects of cell proliferation, differentiation, apoptosis, and inflammation in the body (Peti and Page Citation2013; Yue and López Citation2020). Under persistent hyperglycemia, ERK, JNK, and P38MAPK in MAPK generate activity through phosphorylation of their serine and threonine residues, and then participate in regulating the functions of various downstream transcription factors, affecting the progression of DN (Zhang et al. Citation2020; Li et al. Citation2021; Samsu Citation2021). Studies have shown that in DN, the activities of P38MAPK (p-P38), ERK (p-ERK), and JNK (p-JNK) in renal tissue are significantly increased (Geng et al. Citation2020; He et al. Citation2020). In addition, the generation of renal fibrosis cytokines and collagen in renal tissues is suppressed when the MAPK signalling pathway is blocked. This suggests that the progression of DN is influenced by the MAPK signalling pathway (Lakshmanan et al. Citation2012; Cheng et al. Citation2013). The molecular docking results showed that the binding energies between the main components of M-SYFSF (quercetin, calycosin, formononetin) and core targets of MAPK signaling pathway (ERK, JNK, P38MAPK) were all <−5 kcal/mol and had strong binding activity, suggesting that M-SYFSY may play a role in delaying DN progression by regulating MAPK pathway.
Proximal tubular cells are initiators and key therapeutic targets for DN (Gilbert Citation2017). As such, HK-2 cells were selected as the research model for M-SYFSF acting on the MAPK signalling pathway. AGEs intervention simulates the renal tissue environment during the accumulation of diabetic products. Compared to the control group, p-JNK, p-P38MAPK, and p-ERK were significantly increased in AGEs-treated HK-2 cells, which is consistent with previous studies (Geng et al. Citation2020; He et al. Citation2020).
The optimal therapeutic concentration of M-SYFSF obtained by CCK8 screening was divided into low, medium, and high concentrations (100, 150, and 200 μg/mL) for intervention. The results showed that p-JNK, p-P38MAPK, and p-ERK were significantly decreased in AGEs-treated HK-2 cells after certain concentrations of M-SYFSF interventions. Therefore, MAPK signaling pathway is likely to be involved in the effects of M-SYFSF in the treatment of DN.
The MAPK signalling pathway is a prototypical pro-inflammatory system whose hyperactivation has been linked to a variety of inflammatory disorders. Under persistent hyperglycemia, the MAPK pathway is activated, stimulating the downstream expression of inflammatory substances (Chen Citation2018). The results of in vitro experiments showed that the expression of IL-1β, IL6 and TNF-α in HK-2 cells treated with AGEs were significantly increased. The expression of IL-1β, IL6 and TNF-α can be significantly reduced under the intervention of different concentrations of M-SYFSF, indicating that M-SYFSF is likely to reduce the inflammatory response by inhibiting the MAPK signalling pathway, thereby it can delay the progression of DN. However, this study predicts that the therapeutic effect of M-SYFSF on DN involves multiple signalling pathways and targets, and further research is needed.
Conclusion
In the present study, we conducted research through network pharmacological analysis combined with experimental validation. This research project systematically and comprehensively investigated the M-SYFSF mechanism in the treatment of DN and clarified the synergistic multicomponent and multitarget mechanism of M-SYFSF for the first time. The results demonstrated that M-SYFSF was likely to reduce inflammation by inhibiting the MAPK signalling pathway, thereby delaying the progression of DN. It provides a theoretical basis for the clinical application of M-SYFSF in the treatment of DN.
Authors contributions
Borui Yu, Mengqi Zhou, Wei Jing Liu, Hongfang Liu, and Yaoxian Wang contributed to the study design, practical work, manuscript writing and revision. Huijuan Zheng, Jingwei Zhou, and Guoyong Yu participated in the research design and manuscript writing. Zhaocheng Dong, Yuxue Zhao, Chao Zhang, and Fudong Wei participated in the experiments and data analysis. All authors read and approved the final manuscript.
Disclosure statement
No potential conflict of interest was reported by the author(s).
Additional information
Funding
References
- Barutta F, Bruno G, Grimaldi S, Gruden G. 2015. Inflammation in diabetic nephropathy: moving toward clinical biomarkers and targets for treatment. Endocrine. 48(3):730–742. doi: 10.1007/s12020-014-0437-1.
- Chen P, Yuan Y, Zhang T, Xu B, Gao Q, Guan T. 2018. Pentosan polysulfate ameliorates apoptosis and inflammation by suppressing activation of the p38 MAPK pathway in high glucose-treated HK-2 cells. Int J Mol Med. 41(2):908–914. doi: 10.3892/ijmm.2017.3290.
- Cheng HT, Xu X, Lim PS, Hung KY. 2021. Worldwide epidemiology of diabetes-related end-stage renal disease, 2000-2015. Diabetes Care. 44(1):89–97. doi: 10.2337/dc20-1913.
- Cheng X, Gao W, Dang Y, Liu X, Li Y, Peng X, Ye X. 2013. Both ERK/MAPK and TGF-Beta/Smad signaling pathways play a role in the kidney fibrosis of diabetic mice accelerated by blood glucose fluctuation. J Diabetes Res. 2013:463740. doi: 10.1155/2013/463740.
- Doshi SM, Friedman AN. 2017. Diagnosis and management of type 2 diabetic kidney disease. Clin J Am Soc Nephrol. 12(8):1366–1373. doi: 10.2215/CJN.11111016.
- Forbes JM, Cooper ME. 2013. Mechanisms of diabetic complications. Physiol Rev. 93(1):137–188. doi: 10.1152/physrev.00045.2011.
- Geng XQ, Ma A, He JZ, Wang L, Jia YL, Shao GY, Li M, Zhou H, Lin SQ, Ran JH, et al. 2020. Ganoderic acid hinders renal fibrosis via suppressing the TGF-β/Smad and MAPK signaling pathways. Acta Pharmacol Sin. 41(5):670–677. doi: 10.1038/s41401-019-0324-7.
- Gilbert RE, Cooper ME. 1999. The tubulointerstitium in progressive diabetic kidney disease: more than an aftermath of glomerular injury? Kidney Int. 56(5):1627–1637. doi: 10.1046/j.1523-1755.1999.00721.x.
- Gilbert RE. 2017. Proximal tubulopathy: prime mover and key therapeutic target in diabetic kidney disease. Diabetes. 66(4):791–800. doi: 10.2337/db16-0796.
- Gratton JP, Morales-Ruiz M, Kureishi Y, Fulton D, Walsh K, Sessa WC. 2001. Akt down-regulation of p38 signaling provides a novel mechanism of vascular endothelial growth factor-mediated cytoprotection in endothelial cells. J Biol Chem. 276(32):30359–30365. doi: 10.1074/jbc.M009698200.
- Guo JC, Pan HC, Yeh BY, Lu YC, Chen JL, Yang CW, Chen YC, Lin YH, Chen HY. 2021. Associations between using Chinese herbal medicine and long-term outcome among pre-dialysis diabetic nephropathy patients: a retrospective population-based cohort study. Front Pharmacol. 12(12):616522. doi: 10.3389/fphar.2021.616522.
- He S, Hu Q, Xu X, Niu Y, Chen Y, Lu Y, Su Q, Qin L. 2020. Advanced glycation end products enhance M1 macrophage polarization by activating the MAPK pathway. Biochem Biophys Res Commun. 525(2):334–340. doi: 10.1016/j.bbrc.2020.02.053.
- Heinrich M, Appendino G, Efferth T, Fürst R, Izzo AA, Kayser O, Pezzuto JM, Viljoen A. 2020. Best practice in research - overcoming common challenges in phytopharmacological research. J Ethnopharmacol. 246:112230. doi: 10.1016/j.jep.2019.112230.
- Honma S, Shinohara M, Takahashi N, Nakamura K, Hamano S, Mitazaki S, Abe S, Yoshida M. 2014. Effect of cyclooxygenase (COX)-2 inhibition on mouse renal interstitial fibrosis. Eur J Pharmacol. 740:578–583. doi: 10.1016/j.ejphar.2014.06.027.
- Hu C, Sun L, Xiao L, Han Y, Fu X, Xiong X, Xu X, Liu Y, Yang S, Liu F, et al. 2015. Insights into the mechanisms involved in the expression and regulation of extracellular matrix proteins in diabetic nephropathy. Curr Med Chem. 22(24):2858–2870. doi: 10.2174/0929867322666150625095407.
- International Diabetes Federation. 2021. IDF Diabetes Atlas, 10th edn. Brussels, Belgium. https://www.diabetesatlas.org
- Lakshmanan AP, Thandavarayan RA, Watanabe K, Sari FR, Meilei H, Giridharan VV, Sukumaran V, Soetikno V, Arumugam S, Suzuki K, et al. 2012. Modulation of AT-1R/MAPK cascade by an olmesartan treatment attenuates diabetic nephropathy in streptozotocin-induced diabetic mice. Mol Cell Endocrinol. 348(1):104–111. doi: 10.1016/j.mce.2011.07.041.
- Li J, Jin S, Barati MT, Rane S, Lin Q, Tan Y, Cai L, Rane MJ. 2021. ERK and p38 MAPK inhibition controls NF-E2 degradation and profibrotic signaling in renal proximal tubule cells. Life Sci. 287:120092. doi: 10.1016/j.lfs.2021.120092.
- Lim A. 2014. Diabetic nephropathy – complications and treatment. Int J Nephrol Renovasc Dis. 7:361–381. doi: 10.2147/IJNRD.S40172.
- Lin YK, Gao B, Liu L, Ang L, Mizokami-Stout K, Pop-Busui R, Zhang L. 2021. The prevalence of diabetic microvascular complications in China and the USA. Curr Diab Rep. 21(6):16. doi: 10.1007/s11892-021-01387-3.
- Lohr J, Mazurchuk RJ, Acara MA, Nickerson PA, Fiel RJ. 1991. Magnetic resonance imaging (MRI) and pathophysiology of the rat kidney in streptozotocin-induced diabetes. Magn Reson Imaging. 9(1):93–100. doi: 10.1016/0730-725x(91)90102-r.
- Lu Z, Zhong Y, Liu W, Xiang L, Deng Y. 2019. The efficacy and mechanism of Chinese herbal medicine on diabetic kidney disease. J Diabetes Res. 2019:2697672. doi: 10.1155/2019/2697672.
- Lv J, Wang Z, Wang Y, Sun W, Zhou J, Wang M, Liu WJ, Wang Y. 2017. renoprotective effect of the Shen-Yan-Fang-Shuai formula by inhibiting TNF-α/NF-κB signaling pathway in diabetic rats. J Diabetes Res. 2017:4319057. doi: 10.1155/2017/4319057.
- Ma LL, Wang MZ, Liu YN, Yang HT, Wang YX. 2020. Exploratory study of nephritis prevention solution to delay the timing of dialysis in non-dialysis patients with chronic kidney disease stage 5. Chinese J Trad Chinese Med. 35:2120–2123. Chinese.
- Maezawa Y, Takemoto M, Yokote K. 2015. Cell biology of diabetic nephropathy: roles of endothelial cells, tubulointerstitial cells and podocytes. J Diabetes Investig. 6(1):3–15. doi: 10.1111/jdi.12255.
- Malik S, Suchal K, Khan SI, Bhatia J, Kishore K, Dinda AK, Arya DS. 2017. Apigenin ameliorates streptozotocin-induced diabetic nephropathy in rats via MAPK-NF-κB-TNF-α and TGF-β1-MAPK-fibronectin pathways. Am J Physiol Renal Physiol. 313(2):F414–F422. doi: 10.1152/ajprenal.00393.2016.
- Najafian B, Alpers CE, Fogo AB. 2011. Pathology of human diabetic nephropathy. Contrib Nephrol. 170:36–47.
- Navarro JF, Milena FJ, Mora C, León C, García J. 2006. Renal pro-inflammatory cytokine gene expression in diabetic nephropathy: effect of angiotensin-converting enzyme inhibition and pentoxifylline administration. Am J Nephrol. 26(6):562–570. doi: 10.1159/000098004.
- Ouyang Y, Rong Y, Wang Y, Guo Y, Shan L, Yu X, Li L, Si J, Li X, Ma K. 2021. A systematic study of the mechanism of acacetin against sepsis based on network pharmacology and experimental validation. Front Pharmacol. 12:683645. doi: 10.3389/fphar.2021.683645.
- Peti W, Page R. 2013. Molecular basis of MAP kinase regulation. Protein Sci. 22(12):1698–1710. doi: 10.1002/pro.2374.
- Qi C, Mao X, Zhang Z, Wu H. 2017. Classification and differential diagnosis of diabetic nephropathy. J Diabetes Res. 2017:8637138. doi: 10.1155/2017/8637138.
- Ranganathan P, Jayakumar C, Ramesh G. 2013. Proximal tubule-specific overexpression of netrin-1 suppresses acute kidney injury-induced interstitial fibrosis and glomerulosclerosis through suppression of IL-6/STAT3 signaling. Am J Physiol Renal Physiol. 304(8):F1054–65. doi: 10.1152/ajprenal.00650.2012.
- Roy A, Maiti A, Sinha A, Baidya A, Basu AK, Sarkar D, Sanyal D, Biswas D, Maisnam I, Pandit K, et al. 2020. Kidney disease in type 2 diabetes mellitus and benefits of sodium-glucose cotransporter 2 inhibitors: a consensus statement. Diabetes Ther. 11(12):2791–2827. doi: 10.1007/s13300-020-00921-y.
- Ru J, Li P, Wang J, Zhou W, Li B, Huang C, Li P, Guo Z, Tao W, Yang Y, et al. 2014. TCMSP: a database of systems pharmacology for drug discovery from herbal medicines. J Cheminform. 6:13–18. doi: 10.1186/1758-2946-6-13.
- Samsu N. 2021. Diabetic nephropathy: challenges in pathogenesis, diagnosis, and treatment. Biomed Res Int. 2021:1497449. doi: 10.1155/2021/1497449.
- Selby NM, Taal MW. 2020. An updated overview of diabetic nephropathy: diagnosis, prognosis, treatment goals and latest guidelines. Diabetes Obes Metab. 22 (Suppl 1):3–15. doi: 10.1111/dom.14007.
- Sharma D, Bhattacharya P, Kalia K, Tiwari V. 2017. Diabetic nephropathy: new insights into established therapeutic paradigms and novel molecular targets. Diabetes Res Clin Pract. 128:91–108. doi: 10.1016/j.diabres.2017.04.010.
- Sharma D, Gondaliya P, Tiwari V, Kalia K. 2019. Kaempferol attenuates diabetic nephropathy by inhibiting RhoA/Rho-kinase mediated inflammatory signalling. Biomed Pharmacother. 109:1610–1619. doi: 10.1016/j.biopha.2018.10.195.
- Sharma D, Verma S, Vaidya S, Kalia K, Tiwari V. 2018. Recent updates on GLP-1 agonists: current advancements & challenges. Biomed Pharmacother. 108:952–962. doi: 10.1016/j.biopha.2018.08.088.
- Wang J, Xiang H, Lu Y, Wu T, Ji G. 2021. New progress in drugs treatment of diabetic kidney disease. Biomed Pharmacother. 141:111918. doi: 10.1016/j.biopha.2021.111918.
- Wang W, Sun W, Cheng Y, Xu Z, Cai L. 2019. Role of sirtuin-1 in diabetic nephropathy. J Mol Med (Berl). 97(3):291–309. doi: 10.1007/s00109-019-01743-7.
- Wang X, Hu Y, Zhou X, Li S. 2022. Editorial: network pharmacology and traditional medicine: setting the new standards by combining In silico and experimental work. Front Pharmacol. 13(13):1002537. doi: 10.3389/fphar.2022.1002537.
- Wang X, Wang ZY, Zheng JH, Li S. 2021. TCM network pharmacology: a new trend towards combining computational, experimental and clinical approaches. Chin J Nat Med. 19(1):1–11. doi: 10.1016/S1875-5364(21)60001-8.
- Warren AM, Knudsen ST, Cooper ME. 2019. Diabetic nephropathy: an insight into molecular mechanisms and emerging therapies. Expert Opin Ther Targets. 23(7):579–591. doi: 10.1080/14728222.2019.1624721.
- Wei X, Hou W, Liang J, Fang P, Dou B, Wang Z, Sai J, Xu T, Ma C, Zhang Q, et al. 2021. Network pharmacology-based analysis on the potential biological mechanisms of sinisan against non-alcoholic fatty liver disease. Front Pharmacol. 12:693701. doi: 10.3389/fphar.2021.693701.
- Wu Y, Zhang F, Yang K, Fang S, Bu D, Li H, Sun L, Hu H, Gao K, Wang W, et al. 2019. SymMap: an integrative database of traditional Chinese medicine enhanced by symptom mapping. Nucleic Acids Res. 47(D1):D1110–D1117. doi: 10.1093/nar/gky1021.
- Yao JQ, Gao YX, Wang YX, Teng FB. 2019. Experimental case of diabetic nephropathy treated by staging with the ‘micro-Zhengjia’ theory. Chinese J Clinicians. 47:121–123. Chinese.
- Yue J, López JM. 2020. Understanding MAPK signaling pathways in apoptosis. Int J Mol Sci. 21:2346. doi: 10.3390/ijms21072346.
- Zhang YH, Zhang YQ, Guo CC, Wang LK, Cui YJ, Dong JJ, Liao L. 2020. Prostaglandin E1 attenuates high glucose-induced apoptosis in proximal renal tubular cells by inhibiting the JNK/Bim pathway. Acta Pharmacol Sin. 41(4):561–571. doi: 10.1038/s41401-019-0314-9.