Abstract
Objectives. The aim of this study was to evaluate the effect of myoblast transplantation on left ventricular function, perfusion, and scar formation after compromised coronary flow. Design. A coronary vessel with Ameroid-induced stenosis was ligated and skeletal muscle was biopsied for isolation and cultivation of myoblasts. Two weeks after ligation, animals were randomly selected to receive intramyocardial injections of 2×106 myoblasts or vehicle. Fifteen animals survived the whole study period (n=9 and n=6, respectively). All animals underwent cardiac magnetic resonance imaging (MRI) and angiography pretreatment and four weeks posttreatment. Results. Peak filling rate of the left ventricle improved in the myoblast group (p=0.0048), but not in the control group. Peak ejection rate and duration of diastole improved only in the myoblast group (p=0.049 and p=0.0039, respectively). Ejection fraction or local thickening did not change. Fibrosis and perfusion were similar in both groups, but more microvessels were present histologically in the myoblast group. Conclusions. In this preclinical study, autologous myoblast transplantation improved ischemic heart function via enhanced diastolic filling of the left ventricle.
Heart failure is the leading cause of hospitalization in aging populations of Western countries. Heart failure is caused by diastolic dysfunction in nearly 40% of cases, in which systolic function is normal or near normal. Diastolic heart failure, i.e. cardiac dysfunction with normal ejection fraction, is a major problem for patients and imposes a considerable financial burden on society Citation1, Citation2. Clinical diagnosis and treatment of diastolic heart failure is based on evaluation and elimination of possible causes, especially left ventricular hypertrophy, tachycardia, ischemia, and elevated filling pressures Citation3. The lack of a specific medical treatment for diastolic dysfunction necessitates the search for alternative methods to provide an effective cure.
Results from numerous experimental studies have lead several groups to perform autologous skeletal myoblast transplantations on patients Citation4–7. Feasibility studies have demonstrated safety in transplantation Citation8–10. The MAGIC trial, the first Phase II, double-blind, randomized, multicenter trial, showed a positive effect of myoblasts on the ventricular remodeling process Citation11. This finding suggests that improvement of systolic function is not the sole determinant of the beneficial effect of myoblast transplantation.
Improved diastolic function after cell therapy has previously been reported in an animal model of cryoinjury Citation12, Citation13 and also in human trials Citation14. We have introduced a new large animal model of ischemia-infarction in which both systolic and diastolic dysfunction was explored Citation15. Faster imaging techniques and improved spatial resolution of cardiac magnetic resonance imaging (MRI) have elevated the standards for evaluation of heart volume and function. The aim of our study was to investigate the effect of skeletal myoblast transplantation in terms of functional systolic and diastolic parameters by cardiac MRI in a porcine model of cardiac ischemia-infarction. We also performed angiography to show the effect of cell transplantation on coronary vessels and assessed perfusion by MRI. Amount of scar tissue was evaluated in vivo by MRI and by postmortem histology to examine the effect of myoblast transplantation on fibrosis.
Methods
Domestic pigs (18–26 kg) were used in this study. All animals were maintained and treated in accordance with the “Principles of Laboratory Animal Care” formulated by the National Society for Medical Research and the “Guide for the Care and Use of Laboratory Animals” prepared by the Institute of Laboratory Animal Resources, Commission on Life Science, National Research Council, and published by the National Academy Press, revised 1996. This study was approved by the Local Ethics Committee of Helsinki University Central Hospital and the Provincial State Office of Southern Finland.
The surgical procedures and anesthesia have been described elsewhere Citation15; study design is shown in . In the current study, a model similar to that previously reported Citation15 was used, with the modification of ligation after a two week ischemic period compared to a three week ischemia period in the previous study. The aim of the model is to represent acute-on-chronic myocardial ischemia, with an abrupt occlusion of a chronically stenosed coronary artery. In brief, an Ameroid constrictor was applied around the proximal left circumflex artery (LCx), and a loose thread was placed around the vessel just distal to the constrictor. Two weeks later, the thread ends were tightened with a consequent binding of the coronary artery. During the same anesthesia, a 1 cm3 biopsy was harvested from the lateral vastus muscle. The muscle biopsy was later processed in the laboratory as described below. Two weeks after the ligation, pretreatment angiography and cardiac MRI were performed. The lateral free wall of the left ventricle was then exposed, and 2×106 myoblasts diluted in 2 ml of Dulbecco's modified Eagle medium (DMEM, Gibco) or plain DMEM were injected into the myocardium. The animals were randomized in the cell laboratory by personnel unaware of the results of the heart examinations. Injections were 0.2 ml each, and were applied into the free lateral wall of the left ventricle. The injection area was delineated by a Prolene suture. Four weeks later, the animals were anesthetized again and angiography and MRI were repeated. After completion of studies, sedated animals were sacrificed with an overdose of potassium chloride. Hearts were excised and sliced along the short axis of the left ventricle. Tissue samples were collected from the area of the myoblast injections, and control samples were collected from the anterior septum.
Myoblast processing and cultures
Skeletal myoblasts were obtained from the porcine vastus lateral muscle. Under sterile conditions, a 1 cm3 sample was cut and washed twice in ice-cold Ca2+-Mg2+ -free Hank's balanced salt solution (HBSS, Gibco, Carlsbad, CA) containing antibiotics (100 IU/ml penicillin and 100 µg/ml streptomycin). It was then carefully minced, and the fragments were enzymatically dissociated by several incubations in serum-free DMEM (Gibco) containing 0.25% trypsin and 1 mg/ml type IV collagenase (Worthington, Lakewood, NJ). Between incubations, the fragments were further dissociated by vigorous pipetting. Cells were then filtered from the digested tissue, centrifuged at 400 g for 10 minutes, and washed twice in DMEM containing 10% fetal calf serum (Gibco) and antibiotics. The resulting cell suspension was plated for 45 minutes in uncoated plastic 25 cm2 cell culture flasks to remove fibroblasts by the differential attachment technique. Unattached cells were then replated on cell culture flasks coated with gelatin (Sigma, St. Louis, MI). Cultures were incubated at 37oC in a humidified atmosphere of CO2 (5%) and air (95%). After one week in culture, the cells were detached with trypsin and subcultured in a 1:3 ratio. After another week, the cells were collected for transplantation and desmin staining. For transplantation, the cell count was optimized to 1×106 cells/ml in serum-free DMEM. Vehicle injections contained serum-free DMEM only.
For evaluating the expression of desmin, a universal marker for myocyte lineage, the cells were fixed in −20oC methanol, washed with PBS, and incubated with monoclonal anti-desmin antibody (hybridome supernatant kindly provided by Professor Ismo Virtanen) for 1 hour. They were then washed with PBS and incubated with horseradish peroxidase-conjugated anti-mouse secondary antibody (Dako, Glostrup, Denmark). Immunoreactivity was visualized using the 3-amino-9-ethyl-carbazole (AEC) chromogen. The cells stained 80–90% positive for desmin ().
Histology
After euthanasia, myocardial samples were collected from the LV lateral wall at the site of the infarction, alongside the infarct area, and a control sample was excised from the anterior septum. Hematoxylin-eosin staining was performed for histological evaluation. Skeletal muscle specific anti-nebulin immunohistochemistry was used to localize skeletal myoblasts in the transplanted hearts and anti-von Willebrand factor (vWf) was used to localize vessels in the sections. Collagen was stained with Van Gieson's stain. The samples were analyzed under a light microscope (Olympus Optical) by an experienced pathologist blinded to randomization. Photographs of histologic sections were taken with an Olympus AX70 microscope (Olympus Optical) and analySIS software (Soft Imaging System). The immunostained sections were photographed in five random spots and the pixel intensity values were limited to 8 bits per sampled pixel (range of values, 0–255). The cut-off point for staining was set on a pixel value of 60/255 shades of gray, and the stained pixel fraction was counted using imageJ software (National Institutes of Health, USA).
Angiography
In vivo coronary angiography was performed under anesthesia at one week and five weeks after myoblast transplantation. Siemens multistar T.O.P. angiography equipment (Siemens AG, Munich, Germany) was used with Hexabrix 200 mgl/ml contrast agent (Laboratoire Guerbet, Roissy, France). Two observers blinded to treatment groups determined the LCx size, the degree of LCx stenosis and graded collateral vessels according to Schwartz classification Citation16.
Magnetic resonance imaging
MRI was performed at one and five weeks after myoblast transplantation. The animals were anesthetized with a respiratory rate of 12–14 breaths per minute, positioned supine, and imaged with a 1.5 T Siemens Sonata scanner and a body array coil (Siemens AG, Erlangen, Germany). During image acquisition, respiration was suspended. Image analysis was performed using Argus software (Siemens AG with Argus, Erlangen, Germany). Left ventricular global and regional parameters were assessed from pre- and posttreatment images. The short-axis base was determined by ascertaining its location on long-axis MR images. To image the whole left ventricle (LV), two perpendicular long axis planes and 4–8 parallel short axis planes were acquired. TrueFISP cine images were obtained for LV volume measurements and regional wall thickening analysis. The sequence parameters were: slice thickness 7 mm, slice gap 7 mm apart, temporal resolution 33 ms, FOV (field of view) 300 cm, matrix 126×256 pixels, TE (time to echo) 1.57 ms, TR (time for repeat) 5.3 ms, flip angle 60 degrees Citation17. Endo- and epicardial borders were contoured manually at end diastole and end systole of all the short axis slices from base to apex. The LV cavity area was multiplied with the sum of slice thickness and slice gap, or slice thickness and half of the slice gap in the basal and apical slices. Global volumes were calculated as a sum of all short axis slices at each cardiac-imaging phase, producing 20–26 different ventricular volumes between one R-R interval. Minimum left ventricular volume was determined as end systole and maximal volume as end diastole. Left ventricular ejection fraction (LVEF) was calculated as EF=(EDV-ESV)/EDV, where EF=ejection fraction, EDV=end diastolic volume, and ESV=end systolic volume. The LV muscle mass (LVM) was assessed by adding the myocardial volumes of all the short axis slices. To obtain muscle area, the epicardial border of each section was drawn at diastole. The muscle volume was then multiplied with 1.05 density value. Data of the variation of the LVM over the cardiac cycle was provided by Argus software as mean LVM over the cardiac cycle, end diastolic LVM and standard deviation of the LVM variation.
An inversion recovery turboflash image series was obtained during dipyridamole stress (Persantin, 0.5 mmol/kg in four minutes) and manually injected Gd-DTPA bolus (Magnevist, 0.10 mmol/kg). Four short axis planes were imaged repeatedly at every second for three minutes to observe the myocardial first pass of the contrast agent. The sequence parameters were: slice thickness 8 mm, FOV 300, matrix 62×256, TE 0.99, TR 183, flip angle 8 Citation18. Ten minutes after contrast injection, all long and short axis planes were imaged with an inversion recovery late enhancement sequence to observe the infarction. The sequence parameters were: slice thickness 7 mm, FOV 300, matrix 256, inversion time 270, TE 4.3, TR 750 ms Citation19.
Wall thickening at the free LV wall was compared with the normal left anterior descending (LAD) coronary artery region at the anteroseptal wall on the mid-ventricular short-axis slices. Wall thickness at the left circumflex (LCx) region was measured at systole and diastole. The short axis slices were divided in sections according to American Heart Association statement on myocardial imaging Citation20. All six sections of each short axis slice were measured at the midventricular level. The section with the smallest fractional thickening was selected to represent the ischemic or infarcted area. The fractional thickening of the ischemic or infarcted area was normalized to that of the LAD region in the same short axis slice. The normalized wall thickening was compared between the pretreatment and posttreatment studies. Parameters of ventricular ejection and filling were assessed from short-axis cine images. LV cavity time-volume curves were attained by acquiring data-points of LV volume, as described above, throughout the cardiac cycle. Peak filling rate (PFR) was assessed as the maximum rate of filling (ml/s) between two frames in early diastole. Time to peak filling rate (TPR) was measured as the time (ms) from the end systolic frame to the time of peak filling rate. Duration of diastole (DD) was measured as the time (ms) of the cardiac filling, and peak ejection rate (PER) was assessed as the maximum rate of left ventricular emptying between two frames during systole (ml/s).
Perfusion assessment
The transit of the contrast agent into the myocardium during the first pass imaging was measured by pixel intensity change versus time. The region between the papillary muscles at the mid-ventricular lateral wall at the LCx area was determined to be the region of interest (ROI). The mean pixel intensity value of the manually delineated subendocardial area was detected at the ROI area and at the anterior septum of the same short axis slice using Impax Client-Release 4.5 software (Agfa-Gevaert Group, N.V., Ridgefieldpark, NJ, USA). A slope determined by the intensity change in time was created at the LCx perfusion area and it was normalized in the LAD area slope. Normalized LCx slope of four week's study was subtracted from eight week's study, and this angle is presented as ‘slope difference’.
Scar detection
Manual delineation of regions with high signal intensity was drawn from the short axis slices and multiplied by the slice thickness (Impax software). The scar volume of all the short axis segments was summed for the total scar volume. From the same images the whole left ventricular muscle volume was measured to calculate the percent of scar from the left ventricular mass (%LVM).
Statistical analysis
Statistical analysis was performed with GraphPad Prism (GraphPad Software, Inc, San Diego, CA). All data are expressed as median and range. Intraindividual comparison between pretreatment and posttreatment studies was carried out using Wilcoxon test. Comparison between treatment and control groups was performed using Mann-Whitney U-test. Fisher test was used to compare mortality between the groups. A p-value less than 0.05 was considered statistically significant.
Results
Fifteen of 44 animals survived. Four animals died due to the Ameroid application, 15 died in the ligation procedure, one died in the transplantation, and nine died during the imaging procedures. Cause of death was in almost all cases sustained ventricular fibrillation with no response to cardioversion or medication. The mortality after myoblast transplantation was two animals of 11 (18%). Assessed from the same time point, in the control group no animals died (p=0.51, Fisher's exact test). Median weight of the surviving animals was 21 (range 18–26) kg at the beginning of the study, and no differences between groups were detected. Median weight at the end of the study was 61 (range 50–73) kg, with no differences between groups. All animals showed ischemic changes histologically. Increased fibrosis was present at the border area in all animals; we were unable to show difference between groups. In the remote area, there was no evidence of increased fibrosis in any of the animals. In the myoblast group, anti-nebulin stained myoblasts were observed at the injection site, alongside the fibrotic area (). Significantly more vWf-stained microvessels were seen in the myoblast group in the area at risk along the LCx perfusion area at the lateral left ventricular wall, where the cells were transplanted ().
Figure 3. The upper images (A and B) demonstrate immunostaining for the skeletal muscle-specific nebulin, in samples of the lateral part of the left ventricle, where myoblasts were transplanted. The lower panels (C and D) show specificity controls for the nebulin antibody. The anti-nebulin antibody does not react with myocardium (C), but specifically recognizes skeletal muscle (D).
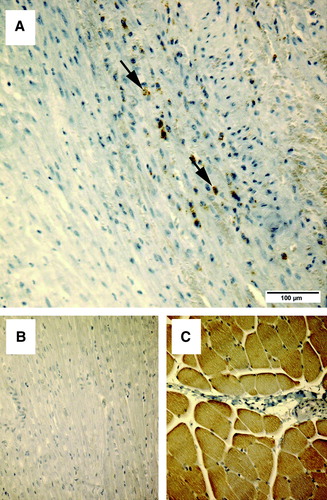
Angiography
One week after the ligation, the pretreatment angiography in the myoblast transplantation group showed a median 100% (range 85–100%) stenosis of the LCx. At five weeks after the ligation, the median LCx stenosis was 100% (range 90–100%). In the control group, the stenosis at both three and seven weeks was 100% (range 90–100% for both, see ). Neither the degree of LCx stenosis nor the size of the LCx vessels differed between the groups. Filling of the LCx via collaterals, according to Schwartz grade, did not differ between groups.
Table I. LCx=left circumflex coronary artery, LVM=left ventricular mass
MRI – Perfusion and scar tissue measurements
The median slope difference in the myoblast group was 22°(range 12–64°) on pre-treatment MRI and 12°(range 5–28) on post-treatment MRI, p=0.09. In the control group, the median slope difference on pre-treatment MRI was 18°(range 0–42°) and on post-treatment MRI was 12°(range 0–22), p=0.12. The change in slope difference did not differ between the groups (p=0.56), indicating that there was no difference in perfusion in the myoblast group and control group in the pretreatment or post-treatment studies.
The median amount of scar tissue in the myoblast group was 2.4 (range 0.2–24)%LVM on pre-treatment MRI, and 3.6 (range 0–16)%LVM on post-treatment MRI, p=0.82. In the control group, the amount of the pre-treatment scar was 6.7 (range 0–14)%LVM on pre-treatment MRI and 8.1 (range 0–15)%LVM on post-treatment MRI, p=0.63, see . There was no difference in the scar%LVM between groups in the pre- or post-treatment studies.
MRI – diastolic parameter
The median change in PFR (peak filling rate) between pre- and post-treatment angiograms indicated significantly improved diastolic filling in the myoblast group, but not in the control group. The median PFR in the myoblast group was 279 ml/s (range 146–441) in the four week's study and 470 ml/s (range 226–1012) in the eight week's study (a). In the control group, the median PFR at one week after ligation was 279 ml/s (range 221–511) and at five weeks after ligation was 339 ml/s (range 267–606) (b). Duration of diastole (DD) was increased in the myoblast group from 324 ms (range 169–528) to 535 ms (range 266–590) during the surveillance (c). DD in the control group was 322 ms (range 225–465) at one week after ligation and 389 ms (range 250–537) at five weeks after ligation (d). There was no change in the TPR (time to peak filling rate) in the myoblast group or in the control group between the one-week and five-week studies. Heart rate and duration of systole did not differ between the groups at pre- or posttreatment (e-h). No difference existed between the groups in end diastolic left ventricular muscle volume according to MRI at one or five weeks (p=0.68 and p=0.27, respectively).
MRI – systolic parameters
The global systolic function in myoblast group, measured as LVEF, was median 51% (range 29–69%) at pretreatment MRI and 50% (range 44–65%) at posttreatment MRI (p=0.18). Respective LVEF in the control group was 57% (range 42–62%) and 49% (range 41–65%) (p=0.56). No difference was present between groups in pretreatment LVEF (p=0.34). Local systolic thickening at the lateral LV wall showed no improvement in either the myoblast group or the control group at the myoblast transplantation area. The median PER (peak ejection rate) in the myoblast group was 277 ml/s (range 155–604) at one week after ligation and 389 ml/s (range 211–655) at five weeks after ligation; this improvement was significant (p=0.049). In the control group, median PER was 266 ml/s (range 205–439) at one week and 389 ml/s (range 211–655) at five weeks postligation (p=0.84). Data of the variation of the LVM over the cardiac cycle is presented in the .
Table II. As left ventricular mass remains unchanged over the cardiac cycle, the variation in the mass at each cardiac-imaging frame represents the (in)accuracy of the left ventricular volume measurement in MRI. This table shows the mean, sem and ED left ventricular mass of each cardiac MRI.
Discussion
In our newly developed porcine model of ischemia-infarction, cardiac diastolic function was improved by autologous myoblast transplantation. This model was specifically designed to imitate the course of ischemic myocardial failure. Our data demonstrate that transplantation of autologous myoblasts after brief expansion in vitro induce beneficial effects on cardiac function. When improved myocardial remodeling after extensive myoblast cultivation has been reported in, for example, the MAGIC trial Citation11, these data suggest that the beneficial effects of myoblast transplantation take place throughout the cardiac cycle and cannot be evaluated only by LVEF or regional wall thickening.
The extensively used Ameroid model of myocardial ischemia and the porcine Ameroid-ligation model Citation15 closely mimic the clinical course of events in the development of myocardial infarction with progressive coronary stenosis, ischemia, and formation of collateral vessels, followed by an abrupt occlusion of the stenosed vessel and consequent infarction of the collateralized myocardium. Importantly, the porcine heart anatomy closely resembles its human counterpart in, for example, the sparse basal network of collateral vessels. It is thus important and clinically relevant to follow the natural course of disease in this model, where a single acute occlusion may produce uncontrollable infarct size, tissue damage, immediate cardiac fibrillations, and death. Moreover, reduced myocardial blood flow as well as increased fibrosis through myocardial infarction presents a clinically significant reason for diastolic dysfunction. In our model, both of these disturbances are present. Because of the nature of our model in which we attempt to imitate acute on chronic myocardial changes, diverse pathophysiological states can be assumed to be present, including infarction with possible edema, hibernation and stunning, or fibrosis with exercise ischemia. As can be seen in the , infarct size varied between individuals.
We were able to show an increased microvessel count in the scar area by vWf staining in the myoblast group compared to control group. Despite this, MRI first pass perfusion images or angiography could not show this difference. In the case of conventional angiograms the reason for the decreased sensitivity is the relatively low resolution of the images. MRI first-pass contrast-enhanced myocardial perfusion imaging measures the function of the coronary flow. When minor differences of perfusion are assessed, no clearly superior method exists. MRI first pass images offer similar sensitivity and specificity as single electron positron emission tomography, but there is still improvement to make. Furthermore, in this model, spontaneous collateral vessels developed before ligation Citation16. In a recent study, skeletal myoblasts were labeled with oxygen-sensing probes before transplantation in mouse hearts. The results revealed significant enhancement of myocardial oxygenation at the site of cell transplant compared with untreated controls Citation21. This finding with a highly sensitive methodology suggests that myoblast transplantation can increase tissue oxygen delivery despite the lack of sensitivity in demonstrating an effect by angiography or by MRI first pass imaging.
The most important finding in our study was the significant improvement of diastolic function with myoblast cell therapy, while no beneficial effect on LVEF or local systolic thickening was observed. Similarly, in a rabbit model of cardiac cryoinjury, cell therapy was shown to have a beneficial impact on diastolic function Citation12, Citation13. An improvement in diastolic function was also implicated by the BOOST cell transplantation trial utilizing bone marrow-derived cells Citation14. In the rabbit cryoinjury model, myoblast therapy was associated with preserved static stiffness of the LV wall, preserved resting myocardial segment length, and increased strain, suggesting improved compliance of the LV wall. This suggests that infarcted myocardium treated with myoblast therapy and engraftment responds with improved diastolic properties Citation13. In bone marrow cell-transplanted rats, an improvement in diastolic function was associated with reduced collagen density. Additionally, bone marrow-derived angioblasts and mesenchymal stem cells have been shown to reduce collagen in the LV wall after acute myocardial infarction (AMI) Citation23, Citation24. In our study, we were unable to show difference in the amount of collagen staining between the groups. Our results suggest an improvement in the elastic properties of the muscle due to increased microvessel density at the area at risk.
Our data indicate that MRI is of excellent value in the evaluation of functional parameters following myoblast transplantation, both in large animal models as well as in clinical settings. The abundant clinically relevant data produced by porcine models speak in favor of more experimental cell transplantation studies using these models.
The mechanism of improved diastolic function by a relatively small mass myoblasts remains unclear. Increased histological count of microvessels at the transplantation area might improve local oxygen supply Citation22. Also release of endocrine or paracrine factors by grafted cells might result in adverse remodeling and thus improved diastolic properties of the LV. The grafted cells were introduced rather early after the ischemic insult and the grafted myotubes might change the physical characteristics of the scar. Further studies are needed to clarify these issues.
According to the Frank-Starling law, diastolic filling is a significant determinant of systolic contractility. Thus, it might be speculated if the increase in LV peak ejection rate was expected as diastolic function improved. Based on our observations, the myoblast-transplanted hearts seemed to function more efficiently, performing systolic work in a reduced time. However, it should be emphasized that the duration of diastole, diastolic filling rate, and LV ejection rate are all determinants of the same cardiac cycle and might be correlated with each other. When both the duration of the diastole and the fastest LV filling slope are increased, the slope of the LV emptying curve might thus be affected (). In contrast to the clear beneficial effects observed in diastolic functional parameters, no effect was evident on systolic parameters, such as ventricular thickening, at the site of myoblast transplantation. This is consistent with the theory that the benefits of myoblast transplantation are more closely linked to mechanisms other than direct improvement of systolic mechanical work. The absolute heart rate is higher in the posttreatment MRI, although it does not reach statistical significance. Also the range varies. This explains at least partly the statistical absurdity of increased duration of diastole, when the duration of systole and heart rate remained unchanged.
Figure 4. Graph of left ventricular volume over time. End systole (es) is the phase of lowest left ventricular volume. The slow filling phase follows until the time to the peak filling rate (tpf) has been reached. The slope of the rapid filling determines the peak filling rate (pfr). The peak ejection rate (per) is the fastest slope of the systolic phase.
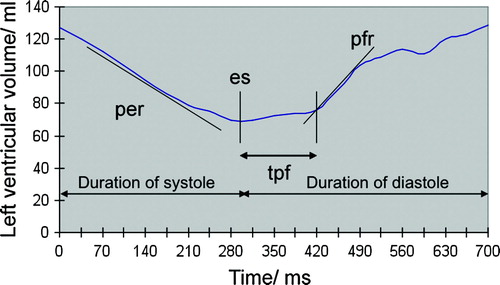
Figure 5. Peak filling rate (panels A and B) increased significantly in myoblast-transplanted animals, but not in control animals. Duration of diastole (panels C and D) is the phase between end systole and end diastole in the cardiac cycle. Diastolic duration improved significantly in myoblast-transplanted animals. A similar improvement was not seen in control animals. Duration of systole (panels E and F) or heart rate (panels G and H) did not change between the study points in either group.
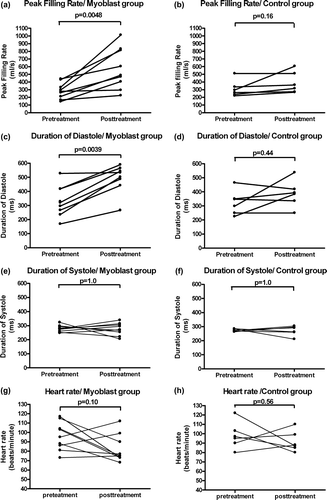
Figure 6. Von Willebrand factor (vWF) staining intensities at core infarct (Core) and infarct border (Border) areas as percentages of vWF staining intensity of the unaffected remote area in control and myoblast-transplanted groups.
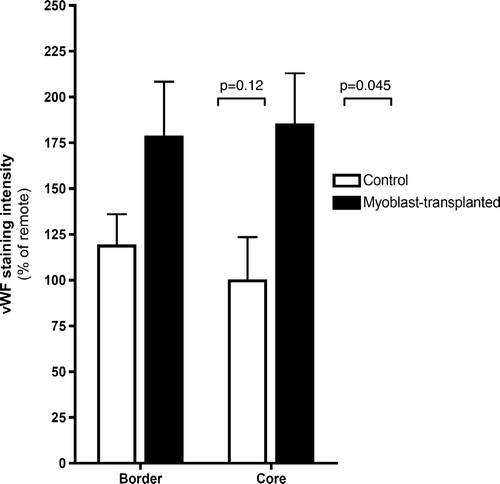
Study limitations include the small number of animals. Because echocardiography (echo) is the most clinically used method for evaluation of diastolic function, the parallel use of echo might have provided more practical information. Also, a longer period of surveillance would have revealed the chronic effect of the myoblasts on diastolic function. Compromise must be done to reduce image acquisition time in MRI, and the use of slice gap decrease the resolution of ventricular volumes. Our MRI data showed some variation in the left ventricular mass over the cardiac cycle, which represents the imprecision in the LV volume measurements ().
In conclusion, our study showed that myoblasts in a porcine model had a beneficial effect on cardiac diastolic function. The benefits of autologous myoblast transplantation lie beyond the improvement in LVEF in this study. The effect of cardiac cell therapy needs to be evaluated from a wider perspective using clinically applicable and objective methodologies.
Acknowledgements
We thank Irina Suomalainen and Lahja Eurajoki for their skillful technical assistance in laboratory work and histological examinations.
References
- Jessup M, Brozena S. Heart Failure. New Engl J Med 2003; 348: 2007–8
- Rich MW, Nease RF. Cost-effectiveness analysis in clinical practise: The case of heart failure. Arch Intern Med 1999; 159: 1690–700
- Aurigemma G, Gaasch W. Diastolic heart failure. New Engl J Med 2004; 351: 1097–105
- Dowell JD, Rubart M, Pasumarthi KB, Soonpaa MH, Field LJ. Myocyte and myogenic stem cell transplantation in the heart. Cardiovasc Res 2003; 58: 336–50
- Menasche P, Desnos M, Hagege AA. Myoblast transplantation during cardiac surgery. Eur Heart J Suppl 2006; 8(Suppl. H): H52–H56
- Niagara MI, Haider H, Jiang S, Ashraf M. Pharmacologically preconditioned skeletal myoblasts are resistant to oxidative stress and promote angiomyogenesis via release of paracrine factors in the infarcted heart. Circ Res 2007; 100: 545
- Murry CE, Field LJ, Menasché P. Cell-based cardiac repair. Reflections at the 10-year Point. Circulation 2005; 112: 3174–83
- Siminiak T, Kalawski R, Fiszer D, Jerzykowska O, Rzezniczak J, Rozwadowska N, et al. Autologous skeletal myoblast transplantation for the treatment of postinfarction myocardial injury: Phase I clinical study with 12 months of follow-up. Am Heart J 2004; 148: 531–7
- Dib N, Michler RE, Pagani FD, Wright S, Kereiakes D, Lengerich R, et al. Safety and feasibility of autologous myoblast transplantation in patients with ischemic cardiomyopathy: Four-year follow-up. Circulation 2005; 112: 1748–55
- Hagege A, Marolleau JP, Vilquin JT, Alheritiere A, Peyrard S, Duboc D, et al. Skeletal myoblast transplantation in ischemic heart failure: Long-term follow-up of the first Phase I cohort of patients. Circulation 2006; 114: 108–13
- Menasché P, Alfieri O, Janssens S, McKenna W, Reichenspurner H, Trinquart L, et al. The Myoblast Autologous Grafting in Ischemic Cardiomyopathy (MAGIC) trial: First randomized placebo-controlled study of myoblast transplantation. Circulation 2008; 117: 1189–200
- Atkins BZ Hueman MT Meuchel JM Hutcheson KA Glower DD Taylor DA. Cellular cardiomyoplasty improves diastolic properties of injured heart. J Surg Res. 85:234–42.
- Atkins BZ, Hueman MT, Meuchel JM, Cottman MJ, Hutcheson KA, Taylor DA. Myogenic cell transplantation improves in vivo regional performance in infarcted rabbit myocardium. J Heart Lung Transplant 1999; 18: 1173–80
- Schaefer A Meyer G Fuchs M Klein G Kaplan M Wollert K et al Impact of intracoronary bone marrow cell transfer on diastolic function in patients after acute myocardial infarction: Results from the BOOST trial. Eur Heart J. 27:929–35.
- Ikonen T Pätilä T Virtanen K Lommi J Lappalainen K Kankuri E et al Ligation of ameroid-stenosed coronary artery leads to reproducible myocardial infarction – A pilot study in a porcine model. J Surg Res. 2007.
- Schwartz H, Leiboff RH, Bren GB, Wasserman AG, Katz RJ, Varghese PJ, et al. Temporal evolution of the human coronary collateral circulation after myocardial infarction. J Am Coll Cardiol 1984; 4: 1088–93
- Francois CJ., Fieno DS., Shors SM., Finn JP. Left ventricular mass: Manual and automatic segmentation of true FISP and FLASH cine MR images in dogs and pigs. Radiology 2004; 230: 389–95
- Sakuma H, Suzawa N, Ichikawa Y, Makino K, Hirano T, Kitagawa K, et al. Diagnostic accuracy of stress first-pass contrast-enhanced myocardial perfusion MRI compared with stress myocardial perfusion scintigraphy. AJR Am J Roentgenol 2005; 185: 95–102
- Selvanayagam JB, Jerosch-Herold M, Porto I., Sheridan D, Cheng AS, Petersen SE, et al. Resting myocardial blood flow is impaired in hibernating myocardium: A magnetic resonance study of quantitative perfusion assessment. Circulation 2005; 112: 3289–96
- Cerqueira MD, Weissman NJ, Dilsizian V, Jacobs A, Kaul S, Laskey W, et al. Standardized myocardial segmentation and nomenclature for tomographic imaging of the heart: A statement for healthcare professionals from the Cardiac Imaging Committee of the Council on Clinical Cardiology of the American Heart Association. Circulation 2002; 105: 539–42
- Pätilä T, Ikonen T, Rutanen J, Ahonen A, Lommi J, Lappalainen K, et al. Vascular endothelial growth factor C-induced collateral formation in a model of myocardial ischemia. J Heart Lung Transplant. 2006; 25: 206–13
- Wisel S, Chacko SM, Kuppusamy ML, Pandian RP, Khan M, Kutala VK, et al. Labeling of skeletal myoblasts with a novel oxygen-sensing spin probe for noninvasive monitoring of in situ oxygenation and cell therapy in heart. Am J Physiol Heart Circ Physiol. 2007; 292: H1254–H1261
- Kocher AA, Schuster MD, Szabolcs MJ, Takuma S, Burkhoff D, Wang J, et al. Neovascularization of ischemic myocardium by human bone marrow-derived angioblasts prevents cardiomyocyte apoptosis, reduces remodeling and inproves cardiac function. Nat Med. 2001; 7: 430–6
- Nagaya N, Fujii T, Iwase T, Ohgushi H, Itoh T, Uematsu M, et al. Intravenous administration of mesenchymal stem cells improves cardiac function in rats with acute myocardial infarction through angiogenesis and myogenesis. Am J Physiol Heart Circ Physiol. 2004; 287: H2670–H2672