Abstract
Objectives. The aim of this study was to investigate the expression of long non-coding RNA (lncRNA) brain and reproductive organ-expressed protein (BRE) antisense RNA 1 (BRE-AS1) in patients with acute myocardial infarction (AMI) and its effect on ischemia/reperfusion (I/R)-induced oxidative stress and apoptosis of cardiomyocytes. Methods. Serum BRE-AS1 levels in patients with AMI was detected using quantitative real-time polymerase chain reaction (qRT-PCR). The diagnostic and prognostic values of BRE-AS1 were evaluated. H9c2 cells were treated with hypoxia/reoxygenation to establish an in vitro myocardial infarction cell model. The levels of inflammatory cytokines such as tumor necrosis factor-α (TNF-α), interleukin-1β (IL-1β), and IL-6 were detected by enzyme-linked immunosorbent assay (ELISA). Levels of lactate dehydrogenase (LDH), malondialdehyde (MDA), superoxide dismutase (SOD), and glutathione peroxidase (GSH-Px) were determined by commercial kits. Cell counting kit-8 (CCK-8) and flow cytometry were used to evaluate the cell viability and cell apoptosis. Results. The expression of BRE-AS1 in serum of patients with AMI is upregulated, which shows the clinical diagnostic value for AMI. In the I/R injury cell model, the knockout of BRE-AS1 can significantly alleviate the increase in TNF-α, IL-1β, and IL-6 levels, inhibit the production of LDH and MDA, increase the activities of SOD and GSH-Px, promote the cell viability and suppress cell apoptosis. Conclusions. Abnormally elevated BRE-AS1 has a high diagnostic value for AMI as well as a prognostic value for major adverse cardiovascular events (MACEs). The elevation of BRE-AS1 promoted oxidative stress injury and cell apoptosis in vitro.
Introduction
Acute myocardial infarction (AMI) is a polygenic disease, the occurrence of which is the product of interactions of genetic and environmental factors. Human cardiomyocytes are one of the few cells that cannot be renewed [Citation1]. When AMI occurs, necrotic myocardial cells are lost, forming persistent tissue scars, leading to sequelae such as heart failure and arrhythmia. Early detection of AMI can reduce complications and improve prognosis [Citation2]. At present, electrocardiography (ECG) is a common method to diagnose myocardial infarction, but approximately 30% of patients have no typical chest pain, and only when the disease occurs can the typical ST segment changes of myocardial infarction be detected by ECG [Citation3]. To date, several blood indicators have been used as biomarkers to assist the diagnosis of AMI, including high-sensitive cardiac troponin I (hs-cTnI) [Citation4]. hs-cTnI is a structural protein of cardiomyocytes, which is usually thought to be released into peripheral blood only when cardiomyocytes are necrotic or damaged. In addition, the non-cardiogenic causes of hs-cTnI elevation include chronic kidney disease, subarachnoid hemorrhage, sepsis, etc. Therefore, the search for new highly sensitive and specific biomarker is critical for the diagnosis and determination of AMI.
In recent years, the regulation of long non-coding RNA (lncRNA) in cardiovascular diseases has become one of the research hotspots. As endogenous competitive RNA, lncRNAs can regulate the biological functions of myocardium and endothelial cells, and participate in the occurrence and development of diseases such as AMI, viral myocarditis, and heart failure [Citation5,Citation6]. For example, ANRIL knockdown weakened the proliferation of vascular smooth muscle cells (VSMCs), which led to changes of gene expression in atherosclerosis-related pathways [Citation7]. Friedrichs et al. reported that SRA1 knockout could lead to ventricular systolic dysfunction in zebrafish, and the relationship between SRA1 and dilated cardiomyopathy was revealed [Citation8]. Brain and reproductive organ-expressed protein antisense RNA 1 (BRE-AS1), also known as NR_028308 or BABAM2, is a 1667 bp non-coding RNA located on human chromosome 2p23.2. BRE-AS1 has been reported to be involved in the regulation of biological functions of various tumor cells, for example, it regulates the proliferation of prostate cancer cells by interacting with miR-145-5p [Citation9], and overexpression of BRE-AS1 inhibits the proliferation of bladder cancer cells by inhibiting signal transduction [Citation10]. With the further development of bioinformatics technology and further exploration of the relationship between lncRNAs and diseases, the relationship between BRE-AS1 and cardiovascular diseases has gradually been discovered. A study found that the increase of BRE-AS1 in the blood of patients with AMI was associated with inflammation and immune responses [Citation11]. Another study demonstrated that the calcium signaling pathway-related gene BRE-AS1 was elevated in patients with ischemic stroke, and it was associated with apoptosis after stroke [Citation12]. At present, there is relatively little research on the role of BRE-AS1 in cardiovascular diseases, and it is of great significance to actively explore its role and mechanism for the diagnosis and treatment of related diseases.
This study aimed to investigate the expression of BRE-AS1 in patients with AMI and its diagnostic value and short-term prognostic value. By constructing a cell model of AMI in vitro, the role of BRE-AS1 in biological function, oxidative stress, and inflammation of the cell model was discussed, which provided a basis for determining the biomarkers of AMI.
Materials and methods
Inclusion of study subjects
Seventy-five patients with AMI in the emergency department of Taihe Hospital were selected as the case group. According to the ESC guidelines for AMI in 2017, the diagnosis of AMI requires to meet the following criteria: (1) precordial and retrosternal pain lasting more than 30 min and was not relieved by oral nitroglycerin; (2) ECG changes (ST elevation or depression; wide and deep nascent Q-waves; T wave inversion); (3) increased levels of typical myocardial injury markers such as CK-MB and cTnI. All patients with AMI were hospitalized within 6 h after onset. Another 70 patients with chest pain were selected as the control group. Control subjects were admitted to the hospital because of chest pain, but were assessed as non-cardiogenic pain by clinical signs and symptoms, laboratory examination, ECG, coronary angiography (CAG), and myocardial perfusion imaging (MPI). After examination, the causes of chest pain in the control group were as follows: cardiac neurosis (five cases), bone or muscle inflammation (21 cases), stomach and esophageal diseases (36 cases), and pneumonia (eight cases). The exclusion criteria are as follows: (1) incomplete clinical information; (2) severe hepatic and renal dysfunction; (3) major surgery or trauma in the last 3 months; (4) history of myocarditis, heart failure or heart valve; and (5) malignant tumors.
The human trials involved in this study confirm to the standards and moral principles of the Declaration of Helsinki. All participants volunteered to take part in this study and signed an informed consent form. This study was approved by the Medical Ethics Committee of Taihe Hospital, Affiliated Hospital of Hubei University of Medicine (Ethical Approval Number: 2021KS022).
Baseline evaluation
Complete information of all subjects was collected, including age, gender, height, weight, medical history, smoking history, and drinking history. Smoking history was defined as past or current smoking ≥100 cigarettes prior to enrollment. Drinking history was defined as drinking any kind of alcohol more than once a week for more than a year. Body mass index (BMI) is calculated by height and weight, the formula: BMI = weight (kg)/height (m)2. In the quiet state, the diastolic blood pressure (DBP) and systolic blood pressure (SBP) of the subjects were measured in supine position with the mercury sphygmomanometer, and the average value of the two measurements was taken. Fasting venous blood was collected for the second day after enrollment, and total cholesterol (TC), triglyceride (TG), high-density lipoprotein cholesterol (HDL-C), low-density lipoprotein cholesterol (LDL-C) and fasting blood glucose (FBG) were measured by Hitachi 7600 automatic biochemical analyzer (Hitachi, Chiyoda City, Japan).
Follow-up analysis
Short-term follow-up was carried out within 30 days after the onset and appropriate treatment was given. According to the average expression level of BRE-AS1, patients with AMI were divided into two groups: high BRE-AS1 expression group (n = 40) and low BRE-AS1 expression group (n = 35). The main follow-up methods were telephone or outpatient follow-up. Major adverse cardiovascular events (MACEs) were recorded, including malignant arrhythmias, myocardial infarction, heart failure, angina pectoris, and cardiac death (see Supplementary Table).
Quantitative real-time PCR analysis
Total RNA in serum was extracted by TRIzol reagent. The absorbance values of the samples at 260 nm and 280 nm were measured by ultraviolet spectrophotometer, and the ratio of A260/A280 and the concentration of RNA samples were calculated. Quantitative real-time polymerase chain reaction (qRT-PCR) was carried out according to the manufacturer’s instructions. The reaction system includes 10 μL of 2× SYBR Green PCR Master Mix, 0.5 μL of forward and reverse primers, 2 μL of cDNA, and the volume was supplemented to 20 μL with RNase-free water. LncRNA BRE-AS1: 5′-CCGCGGTGCCTGACAGTTCC-3′ (forward primer), 5′-TAGTCTCGGTGCACAGCCTC-3′ (reverse primer). GAPDH: 5′-TGAAGGTCGGAGTCAACGGATT-3′ (forward primer), 5′-CATGTGGGCCATGAGGTCCA-3′ (reverse primer). The reaction conditions were as follows: pre-denaturation at 95 °C for 3 min with one cycle, with 40 cycles of denaturation at 95 °C for 30 s, annealing at 60 °C for 30 s, and extension at 72 °C for 30 s. The melting curve confirmed that only one product was amplified in the PCR reaction. △Ct is the difference between the Ct value of the target gene and that of the internal reference GAPDH. The relative expression of target genes was calculated using the 2−△△Ct method.
Cell culture and cell transfection
Rat cardiomyocytes H9c2 were purchased from the American Type Culture Collection (ATCC). The cells were grown in DMEM containing 10% FBS, and were incubated in a constant-temperature incubator at 37 °C after cell resuscitation.
Establishment of ischemia/reperfusion (I/R) injury cell model: H9c2 cells in the logarithmic growth period were added to serum-free medium, cultured for 6 h in an anoxic incubator, and the cells were taken out immediately after the end of the anoxic culture period, and the FBS-free medium was replaced with FBS-containing medium. Then, the cells were cultured in a conventional incubator and incubated for 10 h for reoxygenation culture.
Cell transfection: The cells were divided into four groups: control group, I/R group, I/R + si-negative control group, and I/R + si-BRE-AS1 group. The siRNA negative control (si-NC) and si-BRE-AS1 were purchased from GenePharma (Shanghai, China) and cell transfection was conducted with Lipofectamine 3000 (Invitrogen, Carlsbad, CA). I/R was performed immediately after cell transfection.
Detection of oxidative stress parameters
Lactate dehydrogenase (LDH) release was detected based on a previous study [Citation13]. Two hundred microliters of cell culture supernatant was collected from each group, the corresponding absorbance value was detected according to the kit instructions, and LDH activity was calculated. The thiobarbituric acid method was used to detect the level of malondialdehyde (MDA) in H9c2 cells [Citation14]. The cells were completely lysed, and the supernatant was collected after centrifugation. The reagents were added according to the manufacturer’s instructions. After the reaction was completed, the absorbance value of each group at 532 nm was measured and the MDA content was calculated. The nitroblue tetrazolium (NBT) method was used to measure the superoxide dismutase (SOD) activity. After the cells were lysed, the supernatant was collected and relevant reagents were added. After the reaction was completed, the absorbance of the sample was measured at 550 nm, and the SOD content was calculated. Dinitrothiocyano benzene (DNTB) method was used to detect the concentration of glutathione peroxidase (GSH-Px). Commercially available GSH and GSSG kits were used to detect the interactions between GSH and DNTB.
Enzyme-linked immunosorbent assay
The enzyme-linked immunosorbent assay (ELISA) kit (R&D Systems, Minneapolis, MN) was used to detect the concentration of inflammatory cytokines in H9c2 cells, such as tumor necrosis factor-α (TNF-α), interleukin-1β (IL-1β) and IL-6. H9c2 cells were inoculated into a 96-well plate, and when the cell growth increased to 80%, the cells were treated according to the method described above. One hundred microliters of culture medium was added to the enzyme-labeled plate coated with TNF-α antibody, and incubated at 37 °C for 90 min. Then, the culture medium was removed, the plate was washed with PBS for three times, and TNF-α antibody was added for another 60 min. Subsequently, the working solution of the enzyme conjugate was added to the plate, and the plate was incubated for 30 min. After washing the plate, the color-developing substrate was added, and the plate was incubated for 15 min. The optical density (OD) at 450 nm was measured using a microplate reader. The contents of IL-1β and IL-6 were detected using the same procedure as that for TNF-α.
Cell viability
Cell viability was evaluated using a cell counting kit-8 (CCK-8) assay. Cells in the logarithmic growth phase were seeded into 96-well plates with a density of 1 × 105 cells per well and incubated for 24 h. After the cells were grouped and treated according to the experimental scheme, the CCK-8 solution was added to the culture plate at a preset time point. After another 2 h of cell culture, the absorbance at 450 nm was measured using a microplate reader.
Cell apoptosis
Cell apoptosis was assessed using flow cytometry and the double-staining method. Cells in the logarithmic growth phase were seeded into six-well plates at a density of 5 × 104 cells per well and incubated for 24 h. After grouping and processing the cells according to the experimental scheme, the cells were harvested, and Annexin V-FITC was added to the cells and incubated for 15 min in the dark, followed by the addition of propidium iodide (PI) to the cells and incubation for 15 min in the dark. Finally, after 400 L of the binding solution was added to the cells, apoptosis was detected by flow cytometry.
Statistical analysis
The Kolmogorov–Smirnov test was used to evaluate the normality of the data, and the continuous variables conforming to the normal distribution were expressed as mean ± standard deviation (SD). Student’s t-test was used for comparison of two groups, and one-way analysis of variance (ANOVA) was used for comparison of multiple groups. Categorical variables are expressed in frequency and percentage terms (n, (%)), and the Chi-square test was used for comparative analysis. Receiver operating characteristic (ROC) curve analysis was used to calculate the area under the curve (AUC) value. Logistic regression analysis was used to evaluate the relationship between each factor and AMI occurrence. Kaplan–Meier’s survival curve was drawn to evaluate the difference in short-term prognosis of AMI patients, and log-rank test was used to compare the two groups. Multivariate Cox’s regression analysis was used to express individual prognostic factors with the risk ratio of 95% confidence interval (CI). Gender (male = 1, female = 0), smoking (yes = 1, no = 0), drinking (yes = 1, no = 0). Other factors such as SBP, DBP, TC, TG, LDL-C, HDL-C and FBG were ≥“mean value” = 1 and <“mean value” = 0, respectively. Each experiment was repeated at least thrice. p < .05 is considered a significant difference. Statistical software SPSS 22.0 (SPSS Inc., Chicago, IL) was used for data analysis.
Results
Demographic and clinical characteristics of the two cohorts
As shown in , there were no significant differences in sex, age, or BMI between the two groups (p > .05). In addition, analysis of other indicators and factors related to heart disease showed that there are no differences between the control and AMI groups in terms of drinking and smoking history, DBP, TC, TG, LDL-C, and FBG. However, there were significant differences in SBP and HDL-C levels between the two groups (p < .001).
Table 1. Comparison of baseline data between control and experimental group.
Expression level and diagnostic value of BRE-AS1
The expression of BRE-AS1 in serum of all subjects was analyzed by qRT-PCR. Compared with the control group, the serum expression of BRE-AS1 in the AMI group was upregulated (, p < .001), suggesting that the abnormal increase of BRE-AS1 might be associated with the occurrence of AMI. The clinical diagnostic value of BRE-AS1 was verified via an ROC curve. As shown in , the AUC of BRE-AS1 was 0.868, and its sensitivity and specificity were 82.70% and 81.40%, respectively, which showed that BRE-AS1 has a strong ability to distinguish patients with AMI from patients with chest pain. The influencing factors of AMI were analyzed by logistic regression model. After the data of each factor were included in the model analysis, as shown in , it was found that BRE-AS1 (OR = 2.60, 95%CI = 1.27–5.32, p = .009) was an independent influencing factor for the occurrence of AMI.
Figure 1. The expression and clinical diagnostic value of BRE-AS1 in AMI. (A) The serum expression level of BRE-AS1 in patients with AMI (data are presented as mean and standard deviation, independent samples t-test was used). (B) ROC curve analysis. The area under the curve (AUC) of ROC curve is 0.868, the sensitivity is 82.70% and the specificity is 81.40%. ***p < .001 vs. control group. BRE-AS1: brain and reproductive organ-expressed protein (BRE) antisense RNA 1; AMI: acute myocardial infarction; ROC: receiver operator characteristic; AUC: area under the curve.
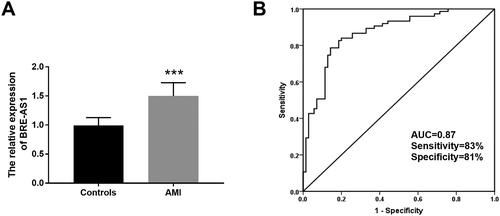
Table 2. Association of different variables with the occurrence of AMI.
Prognostic analysis
According to the average expression level of BRE-AS1, patients with AMI were divided into low BRE-AS1 expression group (n = 35) and high BRE-AS1 expression group (n = 40). summarizes the baseline data of the two groups. The results showed that the levels of SBP, DBP and TG in the high BRE-AS1 expression group were significantly higher than those in the low BRE-AS1 expression group (p < .05). The Kaplan–Meier survival curve was drawn based on the occurrence of MACEs in patients with AMI within 30 days after systemic treatment. Four cases of MACEs occurred in the low BRE-AS1 expression group, including one case of malignant arrhythmia, two cases of angina, and one case of cardiac death. There were 13 cases of MACEs in the high BRE-AS1 expression group, including four cases of malignant arrhythmia, two cases of myocardial infarction, five cases of angina pectoris, and two cases of cardiac death. The results showed that the probability of MACEs in patients with AMI with high BRE-AS1 expression was significantly higher than that in patients with low BRE-AS1 expression within 30 days (, log-rank p = .026). Next, the clinical value of various factors in the short-term prognosis of patients was further evaluated. As shown in , Cox regression model analysis showed that BRE-AS1 was a risk factor affecting the prognosis of patients with AMI (HR = 4.00, 95%CI = 1.14–13.97, p = .030). Besides, Cox regression results also showed that age (HR = 2.45, 95%CI = 0.75–7.97, p = .138) was a risk factor affecting the prognosis of AMI patients, and the risk of poor prognosis in patients ≥60 years old was 2.45 times that of patients <60 years old, although the difference was not significant in this analysis.
Figure 2. Kaplan–Meier’s curves of survival probability of patients with AMI stratified by the serum BRE-AS1 expression levels. BRE-AS1: brain and reproductive organ-expressed protein (BRE) antisense RNA 1; AMI: acute myocardial infarction.
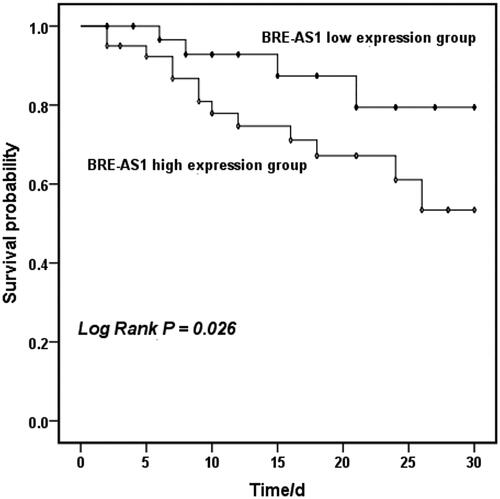
Table 3. Comparison of baseline data between low BRE-AS1 expression group and high BRE-AS1 expression group.
Table 4. Multivariate Cox regression analysis for the overall survival of AMI patients.
Effect of BRE-AS1 on inflammatory response in I/R cell model
The expression of intracellular BRE-AS1 was regulated by in vitro cell transfection. shows that compared with the control group, the expression of BRE-AS1 was significantly enhanced in the I/R injury cell model, and this enhancement effect could be inhibited by transfection with si-BRE-AS1 (p < .001). Subsequently, the levels of pro-inflammatory cytokines were detected by ELISA. Results showed that the levels of TNF-α, IL-1β, and IL-6 in the I/R injury cell model were higher than those in the control group, which indicated that I/R treatment could cause inflammatory damage in H9c2 cells. Furthermore, we also observed an interesting phenomenon: when BRE-AS1 was downregulated, the concentrations of TNF-α, IL-1β, and IL-6 are also reduced (, p < .001).
Figure 3. The effect of BRE-AS1 on inflammatory response in I/R injury cell model. (A) Effect of transfection of si-BRE-AS1 on intracellular BRE-AS1 expression after I/R treatment. Effect of inhibition of BRE-AS1 on the production of (B) TNF-α, (C) IL-1β and (D) IL-6 in I/R cell models. ***p < .001 vs. control group, ###p < .001 vs. I/R + si-NC group. The values shown are mean and standard deviation, one-way ANOVA was used. BRE-AS1: brain and reproductive organ-expressed protein (BRE) antisense RNA 1; I/R: ischemia/reperfusion; TNF-α: tumor necrosis factor-α; IL-1β: interleukin-1β.
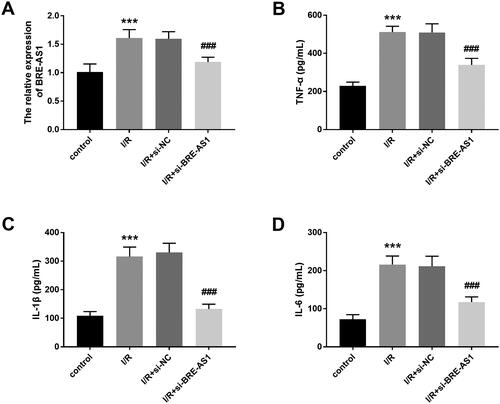
Effect of BRE-AS1 on oxidative stress reaction in I/R cell model
LDH is a main enzyme in the glycolytic pathway and is usually increased significantly in myocardial infarction and hepatitis. Through the detection of LDH, it was found that inhibiting the expression of BRE-AS1 downregulated the increase in LDH after I/R treatment (, p < .001). MDA content is an important parameter reflecting the antioxidant capacity of the body. Hypoxia can produce a lot of oxygen free radicals in myocardial tissue. Our study showed that the content of intracellular MDA increased after I/R treatment, and transfection of si-BRE-AS1 reversed this trend (, p < .001). In addition, the results suggested that the activities of SOD and GSH-Px in H9c2 cells were significantly inhibited by hypoxia, but the activities of SOD and GSH-Px were significantly promoted when the level of BRE-AS1 was downregulated (, p < .001).
Figure 4. The effect of BRE-AS1 on oxidative stress reaction in I/R cell model. The levels of (A) LDH and (B) MDA in I/R cell model was significantly down-regulated by silencing BRE-AS1 expression. The downregulation of BRE-AS1 increased the concentrations of (C) SOD and (D) GSH-Px in H9c2 cells treated with I/R. ***p < .001 vs. control group, ###p < .001 vs. I/R + si-NC group. The values shown are mean and standard deviation, one-way ANOVA was used. BRE-AS1: brain and reproductive organ-expressed protein (BRE) antisense RNA 1; I/R: ischemia/reperfusion; LDH: lactate dehydrogenase; MDA: malondialdehyde; SOD: superoxide dismutase; GSH-Px: glutathione peroxidase.
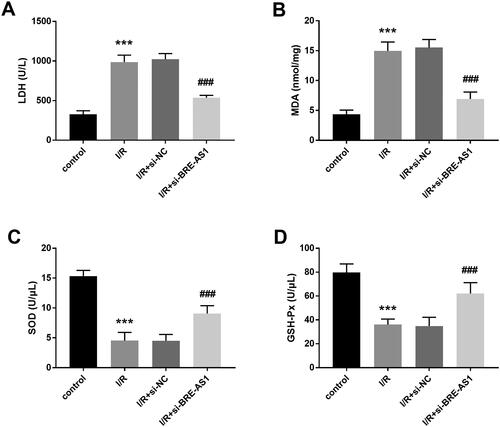
Effect of BRE-AS1 on cell viability and apoptosis in I/R cell model
The effects of BRE-AS1 on the function of H9c2 cells were evaluated by the determination of cell viability and apoptosis. The CCK-8 assay revealed that the cell viability was directly inhibited by hypoxia, and it was enhanced after downregulation of BRE-AS1 (, p < .01). The same trend was observed in the cell apoptosis assay. BRE-AS1 silencing had the ability to reverse I/R-induced apoptosis (, p < .01).
Figure 5. Effect of BRE-AS1 on (A) cell viability and (B) apoptosis in I/R cell model. ***p < .001 vs. control group, ##p < .01, ###p < .001 vs. I/R + si-NC group. The values shown are mean and standard deviation, one-way ANOVA was used. BRE-AS1: brain and reproductive organ-expressed protein (BRE) antisense RNA 1; I/R: ischemia/reperfusion.
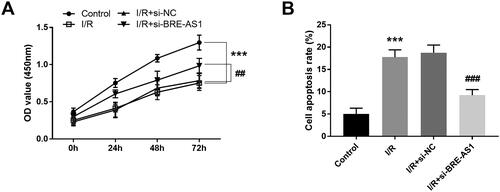
Discussion
In the current study, the expression of BRE-AS1 in the AMI group was significantly higher than that in controls, which suggests that the difference in expression between the two groups may be a result of AMI. This finding is consistent with that of Wang and Jin [Citation11]. In addition, our results have also been supported by Li et al. who identified overexpressed genes in AMI patients, including BRE-AS1 [Citation15]. Here, ROC analysis demonstrates that BRE-AS1 had high accuracy in distinguishing between two groups of patients (chest pain patients and AMI patients), and the AUC value was 0.868. Moreover, the Kaplan–Meier curve and Cox regression analysis showed that the expression level of BRA-AS1 affected the short-term prognosis of patients with AMI, and showed certain prognostic value. LncRNAs, as nucleotide fragments, are not degraded by circulating ribonucleotide enzymes, so they can stably exist in various tissues, such as blood and urine [Citation16]. Owing to its wide distribution and stable properties in vivo, lncRNA may be used as a novel marker for disease diagnosis and therapeutic targets [Citation17]. Studies of lncRNAs as a biomarker for disease diagnosis and prognosis have been reported widely. For example, Peng et al. found that NR-104161 was significantly upregulated in patients with essential hypertension, which may be used as a diagnostic marker for hypertension [Citation18]. Li et al. reported that the expression level of ENST000044488.1 in patients with AMI was 1.5 times higher than that in non-AMI patients, and the ROC curve showed that this lncRNA has a good diagnostic accuracy for AMI [Citation19]. Although BRE-AS1 showed high diagnostic accuracy and prognostic value in patients with AMI, a larger-scale confirmatory study is needed before it can be used as a biomarker. Based on the existing results, larger longitudinal studies should be carried out to verify the diagnostic value of BRE-AS1 for AMI. This could include evaluating the expression of BRE-AS1 in a larger sample size, and following up patients over time to evaluate the predictive value of this marker for future cardiac events.
The dynamic changes of myocardial cells during AMI were simulated by cell level in in vitro study. Studies have shown that oxygen free radicals can be degraded by antioxidant enzymes, maintaining the balance of oxygen free radicals in the body [Citation20]. SOD and GSH-Px are both antioxidant enzymes, and the decrease of their activities can promote cellular oxidative damage [Citation21]. Excessive intracellular oxygen free radicals can accelerate lipid peroxidation of cell membranes, destroy the integrity of cell membrane and promote the release of LDH from cells to the outside [Citation22]. MDA is a product of lipid peroxidation, and its increase can cause intracellular oxidative stress and promote cell apoptosis [Citation23]. However, an increase in cytokine levels can aggravate local inflammatory reactions and promote cell damage, among which TNF-α can promote protein synthesis, increase the synthesis of myosin and actin, and eventually leading to myocardial dysfunction [Citation24]. Increased expression of IL-1β can change the structure and function of myocardial cells, promote myocardial fibrosis, and cause myocardial hypoxia and injury [Citation25]. The combination of these factors aggravates the damage of myocardial cells. The results of this study displayed that the expression of BRE-AS1 in I/R-induced H9c2 cells increased, which was consistent with our previous clinical studies. The inflammatory response was activated after I/R induction, indicating that the levels of TNF-α, IL-1β, and IL-6 increased. At the same time, I/R treatment induced oxidative stress, which mainly manifested in the increase in LDH and MDA, and a decrease in SOD and GSH-Px. In addition, downregulation of BRE-AS1 can improve the inhibition of cell viability and enhancement of apoptosis induced by I/R. It is worth noting that the downregulation of BRE-AS1 significantly ameliorated the above-mentioned adverse effects brought by I/R. In vitro studies show that BRE-AS1 is involved in the occurrence and development of AMI. On this basis, we explored the potential value of BRE-AS1 as the target in the treatment of AMI. This may include testing the efficacy of BRE-AS1 inhibitors or other targeted therapies in reducing oxidative stress and apoptosis of myocardial cells in vitro and in animal models of AMI.
This study had several limitations. First, the sample size of the subjects in the clinical studies is small, which may not completely avoid selection bias. Second, in vitro, we only observed the cellular phenomenon induced by I/R and BRE-AS1 downregulation but did not explore the underlying mechanism. Future studies should continue to explore the possible mechanism of BRE-AS1 in promoting oxidative stress and apoptosis of myocardial cells. This may include exploring the signal pathways and molecular interactions involved in these processes. In future experimental studies, multicenter cohort with large sample size should be selected to make the experimental results more convincing.
In conclusion, the expression of BRE-AS1 increased in the serum of patients with AMI, which has certain clinical diagnostic value for AMI. In the I/R-induced cell model, the levels of BRE-AS1 were upregulated, and inhibition of BRE-AS1 improved oxidative stress, inflammatory response, cell viability, and apoptosis. These results may provide a foundation for studying the relationship between AMI and BRE-AS1 and may provide a powerful tool for the diagnosis and treatment of AMI.
Supplemental Material
Download MS Word (15.6 KB)Disclosure statement
No potential conflict of interest was reported by the author(s).
Additional information
Funding
References
- Hesse M, Welz A, Fleischmann BK. Heart regeneration and the cardiomyocyte cell cycle. Pflugers Arch. 2018;470(2):241–248. doi: 10.1007/s00424-017-2061-4.
- Murphy A, Goldberg S. Mechanical complications of myocardial infarction. Am J Med. 2022;135(12):1401–1409. doi: 10.1016/j.amjmed.2022.08.017.
- Vafaie M. State-of-the-art diagnosis of myocardial infarction. Diagnosis. 2016;3(4):137–142. doi: 10.1515/dx-2016-0024.
- Li H, Zhang P, Li F, et al. Plasma miR-22-5p, miR-132-5p, and miR-150-3p are associated with acute myocardial infarction. Biomed Res Int. 2019;2019:5012648. doi: 10.1155/2019/5012648.
- Fang Y, Xu Y, Wang R, et al. Recent advances on the roles of LncRNAs in cardiovascular disease. J Cell Mol Med. 2020;24(21):12246–12257. doi: 10.1111/jcmm.15880.
- Chen G, Li H, Li X, et al. Loss of long non-coding RNA CRRL promotes cardiomyocyte regeneration and improves cardiac repair by functioning as a competing endogenous RNA. J Mol Cell Cardiol. 2018;122:152–164. doi: 10.1016/j.yjmcc.2018.08.013.
- Alfeghaly C, Sanchez A, Rouget R, et al. Implication of repeat insertion domains in the trans-activity of the long non-coding RNA ANRIL. Nucleic Acids Res. 2021;49(9):4954–4970. doi: 10.1093/nar/gkab245.
- Friedrichs F, Zugck C, Rauch GJ, et al. HBEGF, SRA1, and IK: three cosegregating genes as determinants of cardiomyopathy. Genome Res. 2009;19(3):395–403. doi: 10.1101/gr.076653.108.
- Chen Z, Zhen M, Zhou J. LncRNA BRE-AS1 interacts with miR-145-5p to regulate cancer cell proliferation and apoptosis in prostate carcinoma and has early diagnostic values. Biosci Rep. 2019;39(3):BSR20182097. doi: 10.1042/BSR20182097.
- Zhang L, Liu B, Deng QH, et al. LncRNA BRE-AS1 acts as a tumor suppressor factor in bladder cancer via mediating STAT3. Eur Rev Med Pharmacol Sci. 2020;24(10):5320–5328. doi: 10.26355/eurrev_202005_21314.
- Wang L, Jin Y. Noncoding RNAs as biomarkers for acute coronary syndrome. Biomed Res Int. 2020;2020:3298696. doi: 10.1155/2020/3298696.
- Lin W, Wang Y, Chen Y, et al. Role of calcium signaling pathway-related gene regulatory networks in ischemic stroke based on multiple WGCNA and single-cell analysis. Oxid Med Cell Longev. 2021;2021:8060477. doi: 10.1155/2021/8060477.
- Wang W, Sun X, Jin W. Determination of lactate dehydrogenase in human erythrocytes by capillary electrophoresis with electrochemical detection. J Chromatogr B Analyt Technol Biomed Life Sci. 2003;798(1):175–178. doi: 10.1016/j.jchromb.2003.09.036.
- Li X, Li B, Jia Y. The hepatoprotective effect of Haoqin Qingdan decoction against liver injury induced by a chemotherapeutic drug cyclophosphamide. Evid Based Complement Alternat Med. 2015;2015:978219. doi: 10.1155/2015/978219.
- Li L, Cong Y, Gao X, et al. Differential expression profiles of long non-coding RNAs as potential biomarkers for the early diagnosis of acute myocardial infarction. Oncotarget. 2017;8(51):88613–88621. doi: 10.18632/oncotarget.20101.
- Liu W, Zhuang R, Feng S, et al. Long non-coding RNA ASB16-AS1 enhances cell proliferation, migration and invasion via functioning as a ceRNA through miR-1305/Wnt/β-catenin axis in cervical cancer. Biomed Pharmacother. 2020;125:109965. doi: 10.1016/j.biopha.2020.109965.
- Dong Q, Zhou L, Liu F, et al. Long non-coding RNAs in the development, diagnosis and prognosis of nasopharyngeal carcinoma. Int J Clin Exp Pathol. 2017;10(8):8098–8105.
- Peng W, Cao H, Liu K, et al. Identification of lncRNA-NR_104160 as a biomarker and construction of a lncRNA-related ceRNA network for essential hypertension. Am J Transl Res. 2020;12(10):6060–6075.
- Li L, Wang L, Li H, et al. Characterization of LncRNA expression profile and identification of novel LncRNA biomarkers to diagnose coronary artery disease. Atherosclerosis. 2018;275:359–367. doi: 10.1016/j.atherosclerosis.2018.06.866.
- Jo WS, Yang KM, Park HS, et al. Effect of microalgal extracts of Tetraselmis suecica against UVB-induced photoaging in human skin fibroblasts. Toxicol Res. 2012;28(4):241–248. doi: 10.5487/TR.2012.28.4.241.
- Zhan F, Luo J, Sun Y, et al. Antioxidant activity and cell protection of glycosylated products in different reducing sugar duck liver protein systems. Foods. 2023;12(3):540. doi: 10.3390/foods12030540.
- Huang K, Shi C, Min J, et al. Study on the mechanism of curcumin regulating lung injury induced by outdoor fine particulate matter (PM2.5). Mediators Inflamm. 2019;2019:8613523. doi: 10.1155/2019/8613523.
- Zhao H, Wang X, Liu X, et al. Antioxidant and hypolipidemic activities of acid-depolymerised exopolysaccharides by termitomyces albuminosus. Oxid Med Cell Longev. 2019;2019:8915272. doi: 10.1155/2019/8915272.
- Xiping Z, Chuyang L, Jie Z, et al. Protection of Salvia miltiorrhizae to the spleen and thymus of rats with severe acute pancreatitis or obstructive jaundice. Mediators Inflamm. 2009;2009:186136. doi: 10.1155/2009/186136.
- Tian M, Yuan YC, Li JY, et al. Tumor necrosis factor-alpha and its role as a mediator in myocardial infarction: a brief review. Chronic Dis Transl Med. 2015;1(1):18–26. doi: 10.1016/j.cdtm.2015.02.002.