Abstract
In this study, we report the use of a cyclometalated luminescent iridium(III) complex for the visualization of thiols. The detection of glutathione (GSH) by complex 1 is achieved through the reduction of its phendione N^N donor, which influences the metal-to-ligand charge-transfer (MLCT) of the complex. Complex 1 produced a maximum threefold luminescence enhancement at 587 nm in response to GSH. The linear detection range of 1 for GSH is between 0.2 and 2 M equivalents of GSH, with a detection limit of 1.67 μM. Complex 1 also displays good selectivity for thiols over other amino acids.
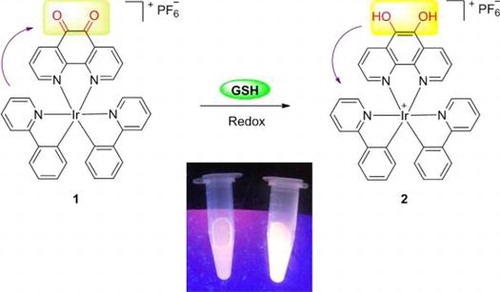
Keywords:
1. Introduction
Biological thiols play essential roles in cell function and maintenance. In particular, glutathione (GSH) is critically involved in redox homeostasis in cellulo. The dysregulation of GSH activity has been linked to diseases such as cancer, cystic fibrosis and neurodegenerative diseases.[Citation1] Therefore, the development of sensitive detection methods for biothiols has recently been an active area of research.
Typical instrumental detection methods for biothiols include liquid chromatography,[Citation2,3] capillary electrophoresis,[Citation4] voltammetry [Citation5] and flow injection.[Citation6] However, those techniques require relatively complex sample preparation protocols and sophisticated instrumentation. Meanwhile, a number of chemosensors have been employed for the detection of thiols based on the thiol addition reaction, reviewed in [Citation7,8]. However, thiol chemosensors are based on organic molecules,[Citation9–17] and only a few examples of transition metal complexes as thiol chemosensors have been reported.
Compared with organic molecules, transition metal complexes are generally relatively easy to synthesize and modify, exhibit large Stokes shifts, and offer long-lived luminescence that could allow them to be potentially used in autofluorescent biological matrices.[Citation18–24] Several iridium(III) complex chemosensors have been reported for thiol detection. Che and co-workers reported a FRET-based luminescent iridium(III) probe for the detection of cysteine (Cys) and homocysteine.[Citation31] Later on some iridium(III) complexes were reported for thiol detection in cellulo.[Citation32, 33] Li, Huang, Yi and co-workers have demonstrated iridium(III) complex chemosensors for selectively detecting homocysteine [Citation34] or both homocysteine and cysteine,[Citation35–38] and have employed these for the visualization of thiol in the cell. While Chao’s group reported an azobis(2,2′-bipyridine)-bridged dinuclear iridium(III) complex for the thiol imaging.[Citation39] Additionally, Chen’s group reported an iridium(III) complex thiol chemosensor based on an α,β-unsaturated ketone motif.[Citation40] In this project, we sought to employ an iridium(III) complex for the detection of thiols based on the redox reaction between GSH and the N^N donor (phendione) of a luminescent iridium(III) complex. We anticipate that the reduction of phendione by thiols would influence the metal-to-ligand charge-transfer (MLCT) state of the iridium(III) complex, thereby allowing the complex to function as a luminescent chemosensor for thiols detection.
2. Experimental details
2.1. Synthesis of [Ir(ppy)2(phendione)](PF6) 1
Complex 1 was reported in previous literature.[Citation41] A suspension of [Ir(ppy)2]2Cl2 (ppy = 2-phenylpyridine) (0.2 mmol) and 1,10-phenanthroline-5,6-dione (phendione) (0.42 mmol) in a mixture of CH2Cl2:MeOH (1:1, 20 ml) was refluxed overnight under N2. The product mixture was then allowed to cool down to 25°C, and was filtered to remove unreacted dimer. To the filtrate, excess amount of NH4PF6 was added and the filtrate was reduced in volume by evaporation until precipitation of the crude product was observed. The precipitate was then filtered and washed by 40 ml water twice followed by 40 ml diethyl ether twice. The product was recrystallized by acetone: diethyl ether vapor diffusion to yield the titled compound as a brown solid.
3. Results and discussion
3.1. Design and synthesis of a thiols chemosensor
The photophysical properties of iridium(III) complex are sensitive to both the solvent environment and the nature of their C^N or N^N donor ligands. Phenanthrene-9,10-quinone, which has been associated with the production of reactive oxygen species (ROS), can be reduced back to catechol in futile redox cycles both enzymatically and nonenzymatically.[Citation42] On the other hand, GSH, as the most abundant non-protein thiol, is a major reductant in internal cellular compartments.[Citation15,43] As a consequence, we chose the structurally related 1,10-phenanthroline-5,6-dione (phendione) moiety as the N^N donor for the iridium(III) complex 1,[Citation41] which also coordinates two C^N ligands (ppy). We anticipated that the reduction of the phendione N^N donor by GSH, generating complex 2, may influence the MLCT state of the iridium(III) complex, thereby allowing 1 to act as a luminescent chemosensor for thiols (Figure ).
3.2. Photophysical properties of 1
We then examined the photophysical properties of complex 1. Complex 1 displayed a 4.26 μs lifetime (Table S1), which is in the same order as those exhibited by phosphorescent transition metal complexes, while organic chemosensors generally exhibit lifetimes in the nanosecond range. This demonstrates the benefit of using transition metal complexes as chemosensors, in which their long-lived luminescence could potentially allow their emission to be identified from a strongly autofluorescent background signal by utilizing time-resolved luminescence spectroscopy. Moreover, 1 exhibited a maximum emission wavelength at 587 nm upon excitation at 350 nm. The Stokes shift is approximately 237 nm, which is much higher than those typically exhibited by organic chemosensors.
3.3. Signal response of 1 to GSH
We next examined the emission response of 1 towards GSH. In the absence of GSH, the luminescence intensity of 1 is weak in a 9:1 mixture of dimethyl sulfoxide and 4-(2-hydroxyethyl)-1-piperazineethanesulfonic acid (DMSO:HEPES, 10 mM, pH 7.0). However, upon addition of GSH, a significant enhancement in the luminescence intensity of 1 was recorded. The luminescence of 1 (20 μM) increased with increasing concentration of GSH and was saturated at two molar equivalents of GSH, with a ca. threefold enhancement (Figure ). A linear relationship (R2 = 0.99) was measured in the range of 0.2‒2 M equivalents of GSH, while the limit of detection at a signal-to-noise ratio of 3 was calculated to be 1.67 μM, which is sufficient for detecting GSH in blood (in micro molar levels).[Citation44] Moreover, the presence of GSH is transduced by 1 into an observable signal that could be observed by the naked eye upon UV illumination (Figure ).
3.4. Mechanism validation
To verify our hypothesis that GSH will reduce the non-emissive complex 1 to form the emissive complex 2, the reaction of 1 with GSH was measured by 1H nuclear magnetic resonance spectroscopy (NMR, Figure ). Upon the addition of GSH, the signals of several protons of the phenanthroline ring were significantly shifted. Moreover, high-resolution mass spectrometry analysis of the product mixture revealed the formation of 2 at m/z = 713.1512 (Figure S2), while the expected signal for complex 1 at m/z = 711.1399 was diminished (Figure S3). Furthermore, Job’s plot analysis was employed to study the binding stoichiometry of the iridium(III) complex 1 with GSH. The highest luminescence intensity of complex 1 was achieved at a mole fraction of approximately 50% GSH (Figure ), suggesting that a 1:1 ratio is the most possible binding stoichiometry of complex 1 with GSH. While 1:1 binding in Job’s plot may suggest that radical anion of 1 which prepared by one electron reduction is as emissive as 2 that presumably useful for the extension to radical/H2O2 sensors.
3.5. Selectivity of iridium(III) complex 1 for GSH
As selectivity is an important parameter for a chemosensor, we evaluated the selectivity of 1 by introducing 0.8 M equivalents of GSH and Cys or 1 M equivalent of common amino acids into a solution of 1 (20 μM) (Figure ). Encouragingly, the luminescence response of 1 towards GSH and Cys was significantly stronger than that of 1 M equivalent of other amino acids while the luminescence enhancement of GSH is twofold higher than that of Cys. This is presumably due to the different oxidizing ability. These results demonstrate the selectivity of the chemosensor 1 for thiols over amino acids. We also performed a competition experiment to investigate the response of 1 towards GSH and (1 M equivalent) in the presence of a mixture of all interfering amino acids (1 M equivalent each). Encouragingly, the luminescence intensity of 1 was not significantly affected upon the addition of different interfering amino acids, indicating that 1 could possibly be utilized to detect thiols in a real sample matrix in the presence of other amino acids.
4. Conclusions
In conclusion, we have employed the iridium(III) complex 1 for the detection of thiols. We postulate that the reduction of the phendione N^N donor by thiols may influence the MLCT state of complex 1, thereby enabling 1 to function as a luminescent chemosensor for thiols. The proposed mechanism of the chemosensor was supported by NMR and high-resolution mass spectrometry analysis. Complex 1 produced a maximum threefold luminescence enhancement at 583 nm in response to GSH. The linear detection range of 1 for GSH is 0.2‒2 M equivalents of GSH, with a detection limit of 1.67 μM. Complex 1 also displays good selectivity for GSH and Cys over common amino acids. Compared with common organic chemosensors, 1 displays a large Stokes shift and a long-lived luminescence that may favor its use in strongly autofluorescent biological samples.
Funding
This work is supported by Hong Kong Baptist University FRG2/14-15/004 and FRG2/15-16/002), the Health and Medical Research Fund [HMRF/14130522], the Research Grants Council [HKBU/201811, HKBU/204612 and HKBU/201913], the French Agence Nationale de la Recherche/Research Grants Council Joint Research Scheme (A-HKBU201/12; Oligoswitch ANR-12-IS07-0001), National Natural Science Foundation of China [21575121], Guangdong Province Natural Science Foundation [2015A030313816], Hong Kong Baptist University Century Club Sponsorship Scheme 2015, Interdisciplinary Research Matching Scheme [RC-IRMS/14-15/06], the Science and Technology Development Fund, Macao SAR [098/2014/A2], the University of Macau [MYRG091(Y3-L2)-ICMS12-LCH, MYRG2015-00137-ICMSQRCM, MRG023/LCH/2013/ICMS and MRG044/LCH/2015/ICMS)].
Disclosure statement
No potential conflict of interest was reported by the authors.
References
- Townsend DM, Tew KD, Tapiero H. The importance of glutathione in human disease. Biomed. & Pharm. 2003;57:145–155.
- Tcherkas YV, Denisenko AD. Simultaneous determination of several amino acids, including homocysteine, cysteine and glutamic acid, in human plasma by isocratic reversed-phase high-performance liquid chromatography with fluorimetric detection. J Chrom A. 2001;913:309–313.10.1016/S0021-9673(00)01201-2
- Amarnath K, Amarnath V, Amarnath K, et al. A specific HPLC-UV method for the determination of cysteine and related aminothiols in biological samples. Talanta. 2003;60:1229–1238.
- Chen G, Zhang L, Wang J. Miniaturized capillary electrophoresis system with a carbon nanotube microelectrode for rapid separation and detection of thiols. Talanta. 2004;64:1018–1023.10.1016/j.talanta.2004.04.022
- Ndamanisha JC, Bai J, Qi B, et al. Application of electrochemical properties of ordered mesoporous carbon to the determination of glutathione and cysteine. Anal BioChem. 2009;386:79–84.
- Zhao C, Zhang J, Song J. Determination of l-cysteine in amino acid mixture and human urine by flow-injection analysis with a biamperometric detector. Anal BioChem. 2001;297:170–176.
- Yin C, Huo F, Zhang J, et al. Thiol-addition reactions and their applications in thiol recognition. Chem Soc Rev. 2013;42:6032–6059.10.1039/c3cs60055f
- Wang J, Liu H-B, Tong Z, et al. Fluorescent/luminescent detection of natural amino acids by organometallic systems. Coor Chem Rev. 2015;303:139–184.
- Liu S-R, Wu S-P. Hypochlorous acid turn-on fluorescent probe based on oxidation of diphenyl selenide. Org Lett. 2013;15:878–881.
- Zhang Y, Shao X, Wang Y, et al. Dual emission channels for sensitive discrimination of Cys/Hcy and GSH in plasma and cells. Chem Commun. 2015;51:4245–4248.
- Xu K, Qiang M, Gao W, et al. A near-infrared reversible fluorescent probe for real-time imaging of redox status changes in vivo. Chem Sci. 2013;4:1079–1086.
- Lou Z, Li P, Sun X, et al. A fluorescent probe for rapid detection of thiols and imaging of thiols reducing repair and H2O2 oxidative stress cycles in living cells. Chem Commun. 2013;49:391–393.
- Kim G-J, Yoon D-H, Yun M-Y, et al. Ratiometric fluorescence probes based on a Michael acceptor type of coumarin and their application for the multichannel imaging of in vivo glutathione. RSC Adv. 2014;4:18731–18736.
- Zhai D, Lee S-C, Yun S-W, et al. A ratiometric fluorescent dye for the detection of glutathione in live cells and liver cancer tissue. Chem Commun. 2013;49:7207–7209.
- Dooley CT, Dore TM, Hanson GT, et al. Imaging dynamic redox changes in mammalian cells with green fluorescent protein indicators. J Biol Chem. 2004;279:22284–22293. 10.1074/jbc.M312847200
- Ji S, Guo H, Yuan X, et al. A highly selective OFF-ON red-emitting phosphorescent thiol probe with large stokes shift and long luminescent lifetime. Org Lett. 2010;12:2876–2879.
- Yu F, Li P, Song P, et al. Facilitative functionalization of cyanine dye by an on-off-on fluorescent switch for imaging of H2O2 oxidative stress and thiols reducing repair in cells and tissues. Chem Commun. 2012;48:4980–4982.
- Zhao Q, Liu S, Shi M, et al. Series of New Cationic Iridium(III) complexes with tunable emission wavelength and excited state properties: structures, theoretical calculations, and photophysical and electrochemical properties. Inorg Chem. 2006;45:6152–6160.
- Liu Q, Yin B, Yang T, et al. A general strategy for biocompatible, high-effective upconversion nanocapsules based on triplet-triplet annihilation. J Am Chem Soc. 2013;135:5029–5037.10.1021/ja3104268
- Liu Q, Yang T, Feng W, et al. Blue-emissive upconversion nanoparticles for low-power-excited bioimaging in Vivo. J Am Chem Soc. 2012;134:5390–5397. 10.1021/ja3003638
- Yu M, Zhao Q, Shi L, et al. Cationic Iridium(III) complexes for phosphorescence staining in the cytoplasm of living cells. Chem Commun. 2008;2115–2117.
- Li C, Yu M, Sun Y, et al. A Nonemissive Iridium(III) complex that specifically lights-up the nuclei of living cells. J Am Chem Soc. 2011;133:11231–11239.10.1021/ja202344c
- Tao Y, Li M, Ren J, et al. Metal nanoclusters: novel probes for diagnostic and therapeutic applications. Chem Soc Rev. 2015;44:8636–8663.10.1039/C5CS00607D
- Kirsch J, Siltanen C, Zhou Q, et al. Biosensor technology: recent advances in threat agent detection and medicine. Chem Soc Rev. 2013;42:8733–8768.10.1039/c3cs60141b
- Leung C-H, Chan D-S, He H-Z, et al. Luminescent detection of DNA-binding proteins. Nucleic Acids Res. 2011;40:941–955.
- Ma D-L, Chan D-S, Leung C-H. Group 9 organometallic compounds for therapeutic and bioanalytical applications. Acc. Chem. Res. 2014;47:3614–3631.
- Leung C-H, Zhong H-J, He H-Z, et al. Luminescent oligonucleotide-based detection of enzymes involved with DNA repair. Chem. Sci. 2013;4:3781–3795.
- Lu L, Chan D-S, Kwong D-W, et al. Detection of nicking endonuclease activity using a G-quadruplex-selective luminescent switch-on probe. Chem. Sci. 2014;5:4561–4568.
- Lin S, Gao W, Tian Z, et al. Luminescence switch-on detection of protein tyrosine kinase-7 using a G-quadruplex-selective probe. Chem. Sci. 2015;6:4284–4290.
- Leung K-H, He H-Z, He B, et al. Label-free luminescence switch-on detection of hepatitis C virus NS3 helicase activity using a G-quadruplex-selective probe. Chem. Sci. 2015;6:2166–2171.
- Shiu H-Y, Wong M-K, Che C-M. “Turn-on” FRET-based luminescent iridium(iii) probes for the detection of cysteine and homocysteine. Chem Commun. 2011;47:4367–4369.
- Dong YP, Shi MJ, Tong BH, et al. Chemiluminescence of a cyclometallated iridium(III) complex and its application in the detection of cysteine. Luminescence. 2012;27:414–418.10.1002/bio.v27.5
- Tang Y, Yang H-R, Sun H-B, et al. Rational design of an “OFF–ON” phosphorescent chemodosimeter based on an iridium(III) complex and its application for time-resolved luminescent detection and bioimaging of cysteine and homocysteine. Chem Eur J. 2013;19:1311–1319.10.1002/chem.201203137
- Chen H, Zhao Q, Wu Y, et al. Selective phosphorescence chemosensor for homocysteine based on an iridium(III) complex. Inorg Chem. 2007;46:11075–11081.
- Liu X, Xi N, Liu S, et al. Highly selective phosphorescent nanoprobes for sensing and bioimaging of homocysteine and cysteine. J Mater Chem. 2012;22:7894–7901.10.1039/c2jm15946e
- Ma Y, Liu S, Yang H, et al. Water-soluble phosphorescent iridium(iii) complexes as multicolor probes for imaging of homocysteine and cysteine in living cells. J Mater Chem. 2011;21:18974–18982.10.1039/c1jm13513a
- Ma Y, Liu S, Yang H, et al. A water-soluble phosphorescent polymer for time-resolved assay and bioimaging of cysteine/homocysteine. J Mater Chem B. 2013;1:319–329.10.1039/C2TB00259K
- Cao X, Wu Y, Liu K, et al. Iridium complex triggered white-light-emitting gel and its response to cysteine. J Mater Chem. 2012;22:2650–2657.10.1039/C2JM13826C
- Li G, Chen Y, Wu J, et al. Thiol-specific phosphorescent imaging in living cells with an azobis(2,2[prime or minute]-bipyridine)-bridged dinuclear iridium(iii) complex. Chem Commun. 2013;49:2040–2042.
- Zhao N, Wu Y-H, Shi L-X, et al. A sensitive phosphorescent thiol chemosensor based on an iridium(iii) complex with [small alpha],[small beta]-unsaturated ketone functionalized 2,2[prime or minute]-bipyridyl ligand. Dalton Trans. 2010;39:8288–8295.10.1039/c0dt00456a
- Shamsipur M, Roushani M, Mansouri G. Highly sensitive and selective amperometric detection of periodate at glassy carbon electrode modified with a cyclometalated iridium(III) complex and single-wall carbon nanotubes. J Chi Chem Soc. 2013;60:171–178.10.1002/jccs.201200146
- Matsunaga T, Arakaki M, Kamiya T, et al. Involvement of an aldo-keto reductase (AKR1C3) in redox cycling of 9,10-phenanthrenequinone leading to apoptosis in human endothelial cells. Chem Biol Inter. 2009;181:52–60.10.1016/j.cbi.2009.05.005
- Chen X, Zhou Y, Peng X, et al. Fluorescent and colorimetric probes for detection of thiols. Chem Soc Rev. 2010;39:2120–2135.10.1039/b925092a
- Anderson ME, Meister A. Dynamic state of glutathione in blood plasma. J Biol Chem. 1980;255:9530–9533.