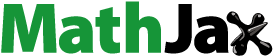
Abstract
We developed a numerical model of the behavior of a red blood cell infected by Plasmodium falciparum malaria on a wall in shear flow. The fluid and solid mechanics of an infected red blood cell (Pf-IRBC) were coupled with the biochemical interaction of ligand-receptor bindings. We used the boundary element method for fluid mechanics, the finite element method for membrane mechanics, and the Monte Carlo method for ligand-receptor interactions. We simulated the behavior of a Pf-IRBC in shear flow, focusing on the effects of bond type. For slip bonds, the Pf-IRBC exhibited firm adhesion, tumbling motion, and tank-treading motion, depending on the applied shear rate. The behavior of catch bonds resembled that of slip bonds, except for a ‘catch’ state at high shear stress. When the reactive compliance decreased to a value in the order of nm, both the slip and catch bonds behaved like an ideal bond. Such bonds do not respond to the force applied to the bond, and the velocity is stabilized at a high shear rate. Finally, we compared the numerical results with previous experiments for A4- and ItG-infected cells. We found that the interaction between PfEMP1 and ICAM-1 could be a nearly ideal bond, with a dissociation rate ranging from
to
.
Graphical Abstract
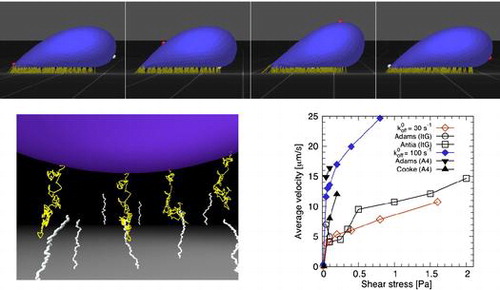
CLASSIFICATION:
1. Introduction
Biomechanical and biochemical properties of cells play important roles in their biological functions. For example, red blood cells (RBCs) transport oxygen to tissues throughout the body, and thus they are highly deformable in order to squeeze through capillaries. However, when an RBC is infected by a malaria parasite, Plasmodium falciparum, the infected red blood cell (Pf-IRBC) changes its biomechanical and biochemical properties. The parasite grows within the host RBC, exporting proteins that modify the cytoskeleton of the cell.[Citation1,Citation2] The Pf-IRBC undergoes a change in its shape from biconcave to more spherical, with knob-like structures on the Pf-IRBC membrane. The stiffness of the membrane then increases, and adhesion molecules are expressed on the surface of the membrane. The parasite ligands, PfEMP1, bind to various receptors on the vascular wall,[Citation3] including intracellular adhesionmolecule-1 (ICAM-1), thrombospondin (TSP) and fcluster of differentiation 36 (CD36).[Citation4–Citation6] Pf-IRBCs adhere to the vascular wall through ligand-receptor interactions to escape splenic clearance, and eventually lead to microvascular occlusion with symptoms including coma or death.[Citation7]
Cooke et al. [Citation8] investigated Pf-IRBC adhesion to walls coated with ICAM-1, TSP, and CD36 using a flow chamber. They found that Pf-IRBCs rolled on ICAM-1, while they firmly adhered on TSP and CD36. An experiment using a microfluidic system [Citation9] also showed Pf-IRBC rolling on ICAM-1 and firm adhesion on CD36. These studies suggest that the interactions between PfEMP1 and these receptors have different biochemical properties, resulting in different Pf-IRBC behaviors. This hypothesis was partly confirmed by a recent experiment using atomic force microscopy (AFM).[Citation10] The AFM experiment demonstrated that the interactions of PfEMP1 with TSP and CD36 were slip bonds, with very low dissociation rate constants that could mediate firm adhesion.
The dissociation rate constant for ICAM-1 is probably higher than that for TSP and CD36. However, AFM experiments for ICAM-1 have not been successful, and its biochemical properties have not been characterized. In addition, a numerical model of a Pf-IRBC failed to simulate rolling motion with slip bonds at a high dissociation rate constant; the Pf-IRBC model exhibited firm adhesion at low shear stress while it detached from the wall at high shear stress.[Citation11,Citation12] Hence, it remains unclear whether slip bonds are even able to mediate Pf-IRBC rolling.
The objective of this study is to quantify the relationship between biochemical properties of ligand-receptor interactions and macroscopic behaviors of Pf-IRBCs. In particular, we focus on the effects of bond type, i.e. slip, ideal, and catch bonds[Citation13], on the rolling motion of Pf-IRBCs. We have previously performed numerical studies on microcirculatory blood flow in malaria infection,[Citation14–Citation16] but the biochemical properties of bonds have not been fully considered. In this study, we develop a numerical model of a Pf-IRBC, coupling the biochemical interactions with the fluid and solid mechanics of the cell. We show that slip bonds are able to mediate Pf-IRBC rolling, and that the interaction between PfEMP1 and ICAM-1 may be a nearly ideal bond.
2. Methods
2.1. Fluid and solid mechanics
Consider the behavior of a Pf-IRBC in a linear shear flow with shear rate on an infinite planar wall coated with receptors. The cell is suspended in a Newtonian fluid with viscosity
. The Pf-IRBC is modeled as a capsule, in which a Newtonian liquid representing cytoplasm with viscosity
is enclosed by a hyper-elastic membrane.
A constitutive law for a biological membrane [Citation17] is used to model the RBC membrane. The strain energy function is given by
(1)
(1)
where is the surface shear elastic modulus, C is the area dilation constant,
, and
and
are the principal extension ratios. The equilibrium condition is given in a weak form as
(2)
(2)
where is the virtual displacement,
is the load on the membrane,
is the virtual strain,
is the Cauchy stress tensor, and S is the surface area of the membrane. The bending energy is given by
(3)
(3)
where is the bending modulus, H is the mean curvature of the surface and
is the reference curvature.[Citation18] The energy function is converted to a force density as
(4)
(4)
where is the Laplace–Beltrami operator on the surface, K is the Gaussian curvature, and
is the normal vector pointing outward from the membrane.[Citation19]
Figure 1. A schematic of bond types. While the dissociation rate for a slip bond increases with , that for a catch bond decreases.
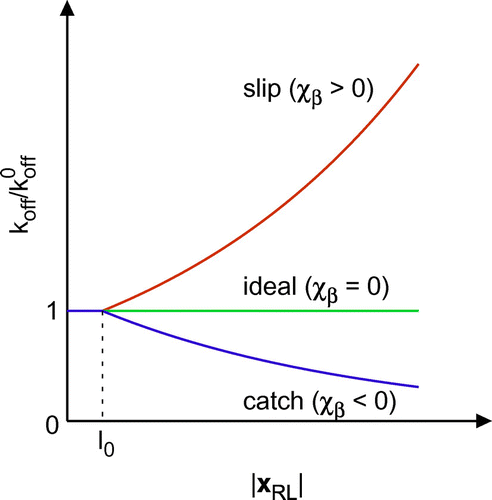
Figure 2. Snapshots of the behaviors of a Pf-IRBC with slip bonds: (a) firm adhesion at , (b) tumbling at
, (c) tank-treading at
, and (d) tank-treading at
. White and red markers represent material points on the major axis of the resting shape.
nm and
.
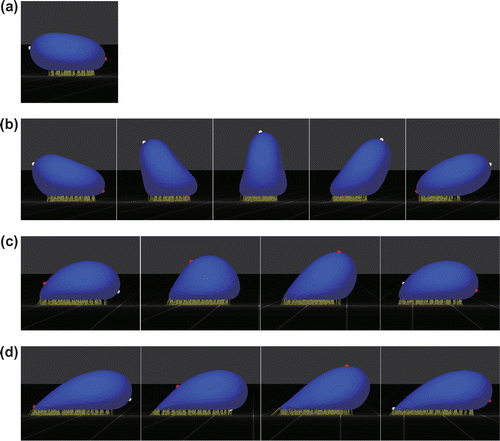
Because the particle Reynolds number is muchsmaller than unity, we assume the flow field to be a Stokes flow. The velocity at a material point
on the membrane is given by the boundary integral equation,
(5)
(5)
where is the background velocity,
is the total force density, and
and
are the Green’s function and associated stress tensor for a fluid domain bounded by an infinite planar wall.[Citation20,Citation21]
The finite element method for membrane mechanics is coupled with the boundary element method for fluid mechanics.[Citation22] Bending resistance is computed as found in Boedec et al. [Citation23] We have developed a graphics processing unit (GPU) computing method for the fluid–structure interaction problem,[Citation24] and have successfully applied it to the rheological analysis of capsule suspensions.[Citation25,Citation26]
2.2. Biochemical interaction
A mathematical model of slip bonds was proposed by Bell [Citation27]. The dissociation rate of a bond is described by(6)
(6)
where is the dissociation rate constant,
is the reactive compliance, and
is the thermal energy. The force
generated by the bond is given by a linear spring model as
(7)
(7)
where is the spring constant,
is the receptor position
on the wall relative to the ligand position
on the membrane, and
is the natural length of the spring. In this study, ideal and catch bonds are also modeled using Equation (Equation6
(6)
(6) ). In the case of a catch bond,
takes a negative value, while
for an ideal bond. A schematic of bond types is shown in Figure .
Figure 3. Snapshots of the behaviors of a Pf-IRBC with catch bonds: (a) tank-treading at and (b) ‘catch’ state at
.
and
. Arrows indicate highly extended bonds.
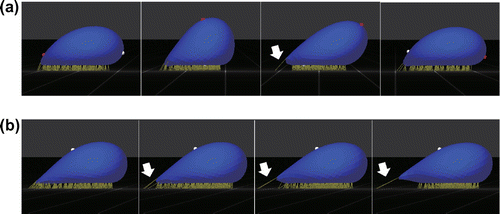
Figure 4. State diagram of the behavior of Pf-IRBCs, where transition refers to transition from tumbling to tank-treading. The state diagram is applicable in the range .
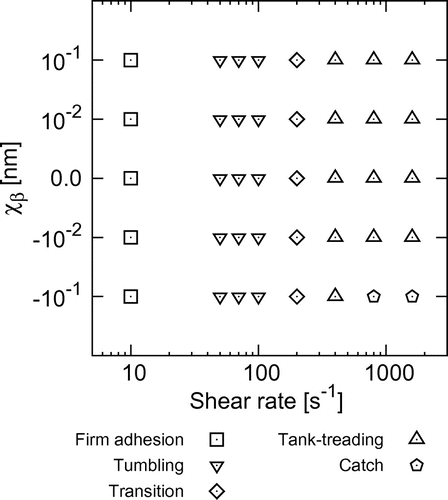
The association rate of a new bond is given by(8)
(8)
where is the association rate constant. In this study, we assume that
and
when the spring is compressed, i.e.
. Bond dissociation and association are computed by a Monte Carlo method [Citation28] with probabilities
(9)
(9)
and(10)
(10)
respectively, where is the time step size.
2.3. Modeling of Pf-IRBCs
We assume that the developmental stage of a malaria parasite is the trophozoite stage. The shape of Pf-IRBCs at the trophozoite stage becomes more spherical relative to healthy RBCs. The relative volume to healthy RBCs is 1.17, and the relative surface area is 0.99.[Citation29] The resting shape of the Pf-IRBC is then modeled as an oblate ellipsoid with major and minor radii of m and
m. The major radius is used as the characteristic length of the problem, i.e.
m. The surface shear elastic modulus is determined to be
N/m by a numerical test problem mimicking the stretching of Pf-IRBCs by optical tweezers,[Citation30] with
and
. The fluid viscosity is assumed to be
s, and the viscosity ratio of cytoplasm to blood plasma is
. A volume constraint [Citation31] is also applied to the cell.
In an AFM experiment,[Citation32] the number of knobs increased to 7 knobs/ by the final schizont stage. It is thought that up to 10 PfEMP1 ligands are present on the top of a knob when the ligands are packed.[Citation33] We set the number density of knobs to be 5 knobs/
and the number density of ligands to be 7 PfEMP1 ligands/knob.
Li et al. [Citation10] quantified Bell model parameters for TSP and CD36 at 310 K using AFM: ,
N/m, and
nm for TSP;
,
N/m, and
nm for CD36. In this study, we fix the spring constant to be
N/m and the thermal energy to be
(
and
). We focus on the rolling motion of Pf-IRBCs with slip, ideal, and catch bonds. Three values of the dissociation rate constant are examined up to
, and the reactive compliance is varied from -0.1 nm to 0.1 nm. In addition, to cover a wide range of physiological flow conditions, the shear rate is varied from
. Measurement of the association rate constant is difficult, and there is no report on association rates for PfEMP1. The reaction rate is fixed to be
to set a high reaction rate condition. We also assume a receptor-rich condition, such that the receptor density on the wall is 64 receptors/
.
One Pf-IRBC is initially placed near the wall, and a slow flow is applied to allow formation of ligand-receptor bonds. Then, the shear rate is raised gradually until a specified shear rate is attained. The average velocity of the cell is obtained from the distance traveled over a given time period. In this study, the mesh size of the boundary element method and finite element method was set to be approximately on an unstructured mesh with 1280 elements. We also set the natural length of bonds
for computational stability, while the actual length of knobs is approximately
.[Citation32,Citation34] Molecular-scale fluctuations are not resolved in the present model, but such fluctuations would have a minor effect on the time-averaged velocity of the Pf-IRBC. In addition, the membrane near the wall moves only at the rear part of the cell during rolling motion, and no lubrication flow is generated between the membrane and wall.
3. Results
3.1. Behavior of a Pf-IRBC on the wall
First, we investigated the behavior of Pf-IRBCs with slip bonds. Typical behaviors of a Pf-IRBC are shown in Figure , where nm. The Pf-IRBC firmly adhered to the wall at a low shear rate of
. The Pf-IRBC experienced lift forces from the wall, but the forces were not large enough to rupture the rearmost bond. A new bond formed at the rearmost part of the cell before rolling motion could occur, and the cell did not move (Figure a). When the shear rate was increased to
, the cell exhibited rolling motion similar to the rotation of a rigid body, called tumbling motion (Figure b). Membrane material points initially on the major axis of the resting shape aligned with the instantaneous orientation of the cell. When the shear rate was increased to a value in the order of
, the Pf-IRBC motion transitioned to another type of rolling motion characterized by tank-treading of the membrane. The material points no longer aligned with the cell orientation. The deformed shape of the Pf-IRBC oscillated during tank-treading, or swinging (Figure c), and the extent of shape oscillation decreased with increasing shear rate (Figure d).
A transition from firm adhesion to tumbling, then tank-treading was also found for Pf-IRBCs with ideal and catch bonds, with the exception of an additional ‘catch’ state. Figure shows a Pf-IRBC with catch bonds at high shear rates. At , the Pf-IRBC exhibited tank-treading motion with shape oscillation similar to that found for slip bonds, but highly extended bonds sometimes appeared at the rearmost part of the cell (Figure a). When the shear rate was increased further, such highly extended bonds finally stopped the cell motion, which we call the catch state (Figure b).
Pf-IRBC behaviors are summarized in a state diagram with respect to the reactive compliance and shear rate. As shown in Figure , cell behaviors do not depend on the bond type, except for the catch state. A Pf-IRBC firmly adheres to the wall until the shear stress reaches a threshold shear rate of approximately 30-50 . The Pf-IRBC then exhibits tumbling and, after that, transitions to tank-treading at a shear rate of 200
. The Pf-IRBC shows tank-treading at high shear stress even for a weak catch bond (
nm), but it transitions to the catch state when
nm. We also examined lower dissociation rate constants of
and
, and the same behaviors were obtained. Hence, the state diagram shown in Figure is applicable at least in the range
.
3.2. Rolling velocity
Next, rolling velocity was quantified for various values of shear rates and reactive compliances. Figure shows the normalized velocities. We found that the values of the normalized velocities are similar for different dissociation rate constants, suggesting that the velocity is nearly proportional to the dissociation rate constant. The velocity first jumped at the transition from firm adhesion to tumbling. For ideal bonds, the velocity increased with the shear rate, with a gradually decreasing slope. Even in the case of slip bonds, the slope slightly decreased with increasing shear rate. This is likely due to a relatively small value of the dissociation rate constant. Catch bonds showed a different velocity response, in which the velocity decreased as the cell approached the catch state. However, when the magnitude of the reactive compliance decreased to nm, the velocities were comparable among the slip, ideal, and catch bonds. Such nearly ideal bonds do not respond to the force applied to the bond, and the velocity was stabilized at high shear rates.
Figure 6. Average velocities of Pf-IRBCs infected with different parasite clones, ItG and A4, in experiments by Adams et al. [Citation38], Antia et al. [Citation9], and Cooke et al. [Citation8]. ICAM-1 concentrations were in [Citation38],
in [Citation9], and 100 or
in [Citation8]. The velocities of the Pf-IRBC model with ideal bonds and dissociation rate constants of
and
are also plotted.
![Figure 6. Average velocities of Pf-IRBCs infected with different parasite clones, ItG and A4, in experiments by Adams et al. [Citation38], Antia et al. [Citation9], and Cooke et al. [Citation8]. ICAM-1 concentrations were 17.5μg/ml in [Citation38], 50μg/ml in [Citation9], and 100 or 400μg/ml in [Citation8]. The velocities of the Pf-IRBC model with ideal bonds and dissociation rate constants of koff0=30s-1 and 100s-1 are also plotted.](/cms/asset/0b0c0bec-f72b-4a2d-9837-e5dd4ee3a8cd/tsta_a_1211462_f0006_oc.gif)
4. Discussion
Quantifying the relationship between the biomechanical and biochemical properties of a cell and its macroscopic behavior is important to understand cell function and related diseases. Plasmodium falciparummalaria is a typical example, in which Pf-IRBCs express adhesion molecules and sequester in the microvasculature through ligand-receptor interactions. A theoretical model of cell adhesion,[Citation35] for example, was applied to analyze experimental data of Pf-IRBC adhesion.[Citation36] However, it is difficult to control molecular conditions in experiments, and the relationship between bond types and Pf-IRBC rolling has not been well understood. We have developed a numerical model of Pf-IRBCs, coupling these biochemical interactions with the fluid and solid mechanics of the cell. Our model demonstrated that the Pf-IRBC transitions from firm adhesion to tumbling, then to tank-treading with increasing shear rate. The state diagram does not depend on the bond type, except for the ‘catch’ state. When the reactive compliance decreases to a value in the order of nm, both the slip and catch bonds behave like an ideal bond. Such nearly ideal bonds do not respond to the force applied to the bond, and the velocity is stabilized at high shear rate.
The interaction between PfEMP1 and ICAM-1 is involved in the pathogenesis of cerebral malaria. The expression level of ICAM-1 is increased in endothelial cells located in the brain, but that of CD36 is not elevated.[Citation37] Hence, the behavior of Pf-IRBCs on ICAM-1 has been extensively studied in experiments using flow chambers and microfluidics. Cooke et al. [Citation8] used a flow chamber to investigate the behavior of Pf-IRBCs on a wall coated with ICAM-1. The majority of Pf-IRBCs exhibited rolling motion except when the shear stress was reduced to 0.02 Pa. This is consistent with our results: the Pf-IRBC model firmly adhered on the wall and did not tumble until the shear rate reached (or corresponding shear stress of 0.03 Pa). In their experiment, Pf-IRBCs continued rolling even when the shear stress was raised to 1 Pa. In our simulation as well, no detachment of Pf-IRBCs from the wall was observed under the examined conditions. Cooke et al. [Citation8] also reported the velocity of rolling Pf-IRBCs as
m/s at 0.1 Pa and
m/s at 0.2 Pa. They used the P. falciparum line A4, but the rolling velocity depends on the type of clonal variants. Adams et al. [Citation38] compared Pf-IRBC rolling between the clonal variants A4 and ItG, and they observed different rolling velocities between the variants. The velocity of A4-infected cells was
m/s at 0.05 Pa and
m/s at 0.1 Pa. The velocity of ItG-infected cells was lower than that of A4-infected cells, at
m/s for 0.05 Pa and
m/s for 0.1 Pa. Antia et al. [Citation9] also usedItG-infected cells and measured rolling velocity for a wide range of shear stresses using a microfluidic device. The velocity was smaller than that for A4-infected cells:
m/s even at 1 Pa. They also reported that the slope of velocity plots as a function of shear stress was not uniform. While the slope gradually increased with the shear stress up to 0.5 Pa, the slope decreased at high shear stress. Our results show that nearly ideal bonds are able to exhibit such a stabilization at high shear stress. Hence, the experimental data mentioned above are summarized in Figure with the numerical data for ideal bonds, where
and
compare with ItG- and A4-infected cells, respectively. Note that Cooke et al. [Citation8] used a saturated concentration of ICAM-1, but Adams et al. [Citation38] and Antia et al. [Citation9] used lower concentrations. Lower velocities are expected if ICAM-1 concentrations are saturated in their experiments. The ideal bond curves follow the experimental data well. This result suggests that the interaction between PfEMP1 and ICAM-1 may behave like a nearly ideal bond, particularly at high shear stress, with a dissociation rate ranging from
to
. This type of stabilizing behavior at high shear stress is also found in leukocyte rolling on selectins,[Citation39] where the reactive compliance for selectin-ligand bonds was reported to be small.[Citation39,Citation40] For ItG-infected cells, the bonds may also behave like a catch bond at low shear stress, and transition to a more nearly ideal bond at high shear stress.
In the numerical model developed by Fedosov et al. [Citation11,Citation12], Pf-IRBC rolling was not observed with slip bonds. They proposed a wall-shear-stress-dependent spring model. This model reproduced the rolling velocities reported in Antia et al. [Citation9], but it remains unclear whether such a complex mechanism is involved in the interaction between PfEMP1 and ICAM-1. We have instead demonstrated that a Pf-IRBC model with slip bonds could roll on the wall. The discrepancy between the two numerical models likely arose from different slip bond models and different values of bond parameters; for example, Fedosov et al. [Citation11,Citation12] used a lower ligand density of 4 ligands and a lower receptor density of 4 receptors
. These conditions will only allow a low number of bond formations, resulting in the detachment of Pf-IRBCs from the wall at higher shear stresses than 0.317 Pa. It remains unclear whether PfEMP1-ICAM-1 bonds are a nearly ideal bond or a wall-shear-stress-dependent bond. Although AFM experiments for ICAM-1 have not been successful, our results will be helpful in understanding experimental data.
In summary, we have developed a numerical model of a Pf-IRBC to clarify the relationship between the biochemical properties of ligand-receptor interactions and the macroscopic behavior of the cell. The Pf-IRBC model exhibited firm adhesion, tumbling motion, and tank-treading motion, depending on the applied shear rate for slip bonds. The behavior for catch bonds resembled that for slip bonds, except for an additional ‘catch’ state at high shear stress. When reactive compliance decreased to a value in the order of nm, both the slip and catch bonds behaved like an ideal bond. Such nearly ideal bonds were able to stabilize the rolling velocity at high shear rate. We compared the numerical results with previous experiments for ItG- and A4-infected cells. We found that the interaction between PfEMP1 and ICAM-1 could be a nearly ideal bond, with a dissociation rate ranging from
to
.
Additional information
Funding
Notes
No potential conflict of interest was reported by the authors.
References
- Glenister FK, Goppel RL, Cowman AF, et al. Contribution of parasite proteins to altered mechanical properties of malaria-infected red blood cells. Blood. 2002;99:1060–1063.
- Mills JP, Diez-Silva MD, Quinn J, et al. Effect of plasmodial RESA protein on deformability of human red blood cells harboring Plasmodium falciparum. Proc. Nat. Acad. Sci. USA. 2007;104:9213–9217.
- Magowan C, Wollish W, Anderson L, et al. Cytoadherence by Plasmodium falciparum-infected erythrocytes is correlated with the expression of a family of variable proteins on infected erythrocytes. J. Exp. Med. 1988;168:1307–1320.
- Barnwell JW, Asch AS, Nachman RL, et al. A human 88-kD membrane glycoprotein (CD36) functions in vitro as a receptor for a cytoadherence ligand on Plasmodium falciparum-infected erythrocytes. J. Clin. Invest. 1989;84:765–772.
- Berendt AR, Simmons DL, Tansey J, et al. Intercellular adhesion molecule-1 is an endothelial cell adhesion receptor for Plasmodium falciparum. Nature. 1989;341:57–59.
- Ockenhouse CF, Tandone NN, Magowan C, et al. Identification of a platelet membrane glycoprotein as a falciparum malaria sequestration receptor. Science. 1992;243:1469–1471.
- Cooke BM, Mohandas NM, Coppel RL. The malaria-infected red blood cell: structural and functional changes. Adv. Parasitol. 2001;50:1–86.
- Cooke BM, Berendt AR, Craig AG, et al. Rolling and stationary cytoadhesion of red blood cells parasitized by Plasmodium falciparum: separate roles for ICAM-1, CD36 and thrombospondin. Br J. Haematol. 1994;87:162–170.
- Antia M, Herricks T, Rathod PK. Microfluidic Modeling of Cell-Cell Interactions in Malaria Pathogenesis. PLoS Pathogen. 2007;3:e99.
- Li A, Lim TS, Shi H, et al. Molecular Mechanistic Insights into the Endothelial Receptor Mediated Cytoadherence of Plasmodium falciparum-Infected Erythrocytes. PLoS One. 2011;6:e16929.
- Fedosov DA, Caswell B, Suresh S, et al. Quantifying the biophysical characteristics of Plasmodium-falciparum-parasitized red blood cells in microcirculation. Proc. Nat. Acad. Sci. USA. 2011;108:35–39.
- Fedosov DA, Caswell B, Karniadakis GE. A wall-shear-stress based model for adhesive dynamics of red blood cells in malaria. Biophys. J. 2011;100:2084–2093.
- Rakshit S, Zhang Y, Manibog K, et al. Ideal, catch, and slip bonds in cadherin adhesion. Proc. Nat. Acad. Sci. USA. 2012;109:18815–18820.
- Imai Y, Kondo H, Ishikawa T, et al. Modeling of hemodynamics arising from malaria infection. J. Biomech. 2010;43:1386–1393.
- Imai Y, Nakaaki K, Kondo H, et al. Margination of red blood cells infected by Plasmodium falciparum in a microvessel. J. Biomech. 2011;44:1553–1558.
- Kondo H, Imai Y, Ishikawa T, et al. Hemodynamic analysis of microcirculation in malaria infection. Ann. Biomed. Eng. 2009;37:702–709.
- Skalak R, Tozeren A, Zarda RP, et al. Strain Energy Function of Red Blood Cell Membranes. Biophys. J. 1973;13:245–264.
- Helfrich W. Elastic Properties of Lipid Bilayers: Theory and Possible Experiments. Z Naturforsch C. 1973;28:693–703.
- Zhong-Can O, Helfrich W. Bending energy of vesicle membranes: General expressions for the first, second, and third variation of the shape energy and applications to spheres and cylinders. Phys. Rev. A. 1989;39:5280–5288.
- Blake JR. A note on the image system for a stokeslet in a no-slip boundary. Math. Proc. Camb. Philos. Soc. 1971;70:303–310.
- Nix S, Imai Y, Ishikawa T. Lateral migration of a capsule in a parabolic flow. J. Biomech. 2016;49:2249–2254.
- Walter J, Salsac A-V, Barthès-Biesel D, et al. Coupling of finite element and boundary integral methods for a capsule in a Stokes flow. Int. J. Numer. Meth. Eng. 2010;83:829–850.
- Boedec G, Leonetti M, Jaeger M. 3D vesicle dynamics simulations with a linearly triangulated surface. J. Comput. Phys. 2011;230:1020–1034.
- Matsunaga D, Imai Y, Omori T, et al. A full GPU implementation of a numerical method for simulating capsule suspensions. J. Biomech. Sci. Eng. 2014;14:00039.
- Matsunaga D, Imai Y, Yamaguchi T, et al. Deformation of a spherical capsule under oscillating shear flow. J. Fluid Mech. 2015;762:288–301.
- Matsunaga D, Imai Y, Yamaguchi T, et al. Rheology of a dense suspension of spherical capsules under simple shear flow. J. Fluid Mech. 2016;786:110–127.
- Bell GI. Models for the specific adhesion of cells to cells. Science. 1978;200:618–627.
- Hammer DA. Adhesive Dynamics. J. Biomech. Eng. 2014;136:021006.
- Esposite A, Choimet J-B, Skepper JN, et al. Quantitative imaging of human red blood cells infected with Plasmodium falciparum. Biophys. J. 2010;99:953–960.
- Suresh S, Spatz J, Mills JP, et al. Connections between single-cell biomechanics and human disease states: gastrointestinal cancer and malaria. Acta Biomater. 2005;1:15–30.
- Freund JB. Leukocyte margination in a model microvessel. Phys. Fluids. 2007;19:023301.
- Li A, Mansoor AH, Tan KSW, et al. Observations on the internal and surface morphology of malaria infected blood cells using optical and atomic force microscopy. J Microbiol. Meth. 2006;66:434–439.
- Carvalho PA, Diez-Silva M, Chen H, et al. Cytoadherence of erythrocytes invaded by Plasmodium falciparum: quantitative contact-probing of a human malaria receptor. Acta Biomater. 2013;9:6349–6359.
- Nagao E, Kaneko O, Dvorak JA. Plasmodium falciparum-infected erythrocytes: qualitative and quantitative analyses of parasite-induced knobs by atomic force microscopy. J. Struct. Biol. 2000;130:34–44.
- Efremov A, Cao J. Bistability of cell adhesion in shear flow. Biophys. J. 2011;101:1032–1040.
- Xu X, Efremov AK, Li A, et al. Diabetes Mellitus and Risk of Bladder Cancer: A Meta-Analysis of Cohort Studies. PLoS One. 2013;8:e64763.
- Turner GDH, Morrison H, Jones M, et al. An immunohistochemical study of the pathology of fatal malaria. Evidence for widespread endothelial activation and a potential role for intercellular adhesion molecule-1 in cerebral sequestration. Am. J. Pathol. 1994;145:1057–1069.
- Adams S, Turner GDH, Nash GB, et al. Differential binding of clonal variants of Plasmodium falciparum to allelic forms of intracellular adhesion molecule 1 determined by flow adhesion assay. Infect. Immun. 2000;68:264–269.
- Yago T, Leppänen A, Qiu H, et al. Distinct molecular and cellular contributions to stabilizing selectin-mediated rolling under flow. J. Cell. Biol. 2002;158:787–799.
- Chen S, Springer TA. Selectin receptor-ligand bonds: Formation limited by shear rate and dissociation governed by the Bell model. Proc. Nat. Acad. Sci. USA. 2001;98:950–955.