Abstract
Au–FexOy composite nanoparticles (NPs) are of great technological interest due to their combined optical and magnetic properties. However, typical syntheses are neither simple nor ecologically friendly, creating a challenging situation for process scale-up. Here we describe conditions for preparing Au–FexOy NPs in aqueous solutions and at ambient temperatures, without resorting to solvents or amphiphilic surfactants with poor sustainability profiles. These magnetic gold nanoclusters (MGNCs) are prepared in practical yields with average sizes slightly below 100 nm, and surface plasmon resonances that extend to near-infrared wavelengths, and sufficient magnetic moment (up to 6 emu g–1) to permit collection within minutes by handheld magnets. The MGNCs also produce significant photoluminescence when excited at 488 nm. Energy dispersive X-ray (EDX) analysis indicates a relatively even distribution of Fe within the MGNCs, as opposed to a central magnetic core.
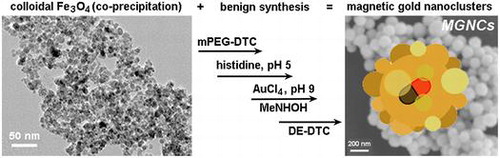
Classification:
- 40 Optical
- magnetic and electronic device materials
- 102 Porous / Nanoporous / Nanostructured materials
- 103 Composites
- 106 Metallic materials
- 203 Magnetics / Spintronics / Superconductors
- 204 Optics / Optical applications
- 301 Chemical syntheses / processing
- 308 Materials resources / recycling
- 501 Chemical analyses
- 503 TEM, STEM, SEM
1. Introduction
Nanoparticles with hybrid magnetic–plasmonic properties are highly prized for applications in separations, biosensing, and nanomedicine [Citation1–11]. These NPs are often comprised of a superparamagnetic iron-oxide core coated with a shell of metallic Au or Ag, which supports a localized plasmon resonance and a certain degree of chemical protection to the magnetic core. The metallic shells can be functionalized by a variety of surface chemistries, many of which enhance the dispersion and compatibility of NPs in biological media [Citation12–19]. This has enabled the use of Au–FexOy NPs for isolating and purifying biomolecular species like proteins and DNA, as multimodal contrast agents in biomedical imaging, and as photothermal agents for hyperthermia-mediated cancer therapies [Citation3,8,10,11,20–26]. The optical properties of these nanocomposites can also be exploited for surface-enhanced Raman scattering (SERS) and other plasmon-enhanced processes, for the specific detection of trace analytes in aqueous media [Citation27–29].
Despite their strong technological potential, nearly all syntheses of Au–FexOy NPs have one or more steps that use nonpolar organic solvents, elevated temperatures, or a high concentration of non-biodegradable surfactants. All of these are negative factors from the perspective of sustainable manufacturing and lifecycle assessment, with significant burdens on the environment, energy consumption, and waste, which translates to higher production costs [Citation30]. Sustainable manufacturing is practiced by chemical industries worldwide, with the intent of meeting the triple bottom-line goals of societal acceptance, cost-effective production, and environmental sustainability. Nano-manufacturing is based on similar principles, but must address inevitable tradeoffs between materials performance and sustainable production while still providing a net technological advantage. We thus seek alternative, greener methods for synthesizing Au–FexOy NPs, with minimum concerns for environmental impact upon scaled production.
Recently, we reported a mild method of synthesizing magnetic gold nanoclusters (MGNCs) that is both simple and eco-friendly, and demonstrated their utility for detecting trace organic pollutants by SERS [Citation29]. In this paper we describe an optimized and highly reproducible method for synthesizing MGNCs in aqueous alcohol, based on systematic adjustments in reagent concentrations and reaction conditions. Energy-dispersive X-ray (EDX) imaging by scanning electron microscopy (SEM) supports a heterogeneous distribution of Fe within MGNCs. The magnetization of the MGNCs can be as high as 6 emu g–1, sufficient for their precipitation by handheld magnets to enable applications in biomolecular separations.
2. Materials and methods
All materials were obtained from commercial sources and used as received, unless otherwise noted. Deionized water was obtained from an ultrafiltration system (Milli-Q, Millipore, Temecula, CA, USA) with a measured resistivity above 18 MΩ·cm, and passed through a 0.22-μm filter to remove particulate matter. CS2 was used as supplied and stored with minimum exposure to air.
Nanomaterials were characterized by transmission electron microscopy (TEM) using a Tecnai-T20 microscope (FEI, Hillsboro, OR, USA). TEM samples were prepared by floating carbon-coated grids on top of an aqueous NP dispersion for 30 min, followed by removal of the grid and drying in air for at least 60 min prior to analysis. Energy-dispersive X-ray (EDX) analysis by scanning transmission electron microscopy (STEM) was performed using a Tecnai G2 T20 microscope (FEI) equipped with a LaB6 filament and X-Max 80 silicon drift detector (Oxford, UK), with data collected by a high-angle annular dark field (HAADF) detector (Fischione, Export, PA, USA) and recorded by a 2 k × 2 k CCD imaging camera (Gatan, Pleasanton, CA, USA) using Inca software (ETAS, Stuttgart, Germany). Atomic absorption spectroscopy (AAS) was performed with a Perkin-Elmer (Waltham, MA, USA) 3110 spectrometer, using materials dissolved in aqua regia. Attenuated total reflectance-infrared (ATR-IR) analyses were performed on powder samples deposited on a ZnSe window, using a Nexus 670 spectrometer (Thermo, Waltham, MA, USA) flushed with N2 to remove atmospheric CO2 and moisture. Photoluminescence (PL) imaging was performed on air-dried samples with an Olympus (Tokyo, Japan) FV1000 laser scanning confocal microscope using a 10× confocal objective and 4.5× zoom lens, and three different laser lines with appropriate filters (excitation/emission = 488/505−525 nm; 543/560−620 nm; 635/655−755 nm).
Hydrodynamic size analysis of aqueous dispersions was performed by nanoparticle tracking analysis (NTA) using a Nanosight LM-10 (Malvern Instruments, Worcestershire, UK), with 405-nm laser excitation and distilled, particle-free water stored in polyethylene containers. Three tracking videos were collected per sample with a minimum of 2000 particle tracks per run, yielding hydrodynamic size (dh) values based on mode peak analysis. Inductively coupled plasma mass spectrometry (ICP-MS) was performed at the University of Illinois. Magnetic properties were measured at room temperature on neat powders using a MPMS-3 magnetometer (Quantum Design, San Diego, CA, USA) in vibrating sample magnetometer mode, with applied magnetic fields up to 10 kOe, and calibrated with colloidal γ-Fe2O3 [Citation31]. Extinction spectra were obtained using a Cary-50 visible-near infrared spectrophotometer (Varian, Palo Alto, CA, USA) in transmission mode.
2.1. Synthesis and modification of colloidal iron oxide
Colloidal iron oxide (Fe3O4) was prepared by co-precipitation, using 648 mg of FeCl3 (4 mmol) and 398 mg of FeCl2∙4 H2O (2 mmol) dissolved in 5 ml of deaerated, deionized water, added dropwise to 15 ml of a 28% NH4OH solution over a period of 10 min in a glass test tube, while immersed in an ultrasonic cleaning bath. Colloidal Fe3O4 was formed immediately upon addition; care was taken to maintain anaerobic conditions during the dropwise addition of iron salts to the NH4OH solution. The reaction mixture was then removed from the ultrasonic bath and agitated for 2 min by vortex mixing to generate a homogeneous dispersion. Colloidal Fe3O4 was precipitated by applying an external handheld magnet along the walls of the reaction tube, then redispersed in deionized water. This process was repeated several times to remove weakly magnetized colloidal oxides. Final weights of magnetically active materials were obtained after drying the precipitated colloids in an oven, but were otherwise used as freshly prepared dispersions at a concentration of 8 mg ml–1.
To prepare mPEG-coated Fe3O4, 20 mg of 5-kDa mPEG-NH2 was dissolved in 1 ml of dry, deaerated methanol (4 mM) and stirred for 10 min, treated with one equivalent of CS2 (4 μmol) diluted in methanol and stirred for another 10 min, then treated with triethylamine (4 μmol) and stirred for 30 min at room temperature, resulting in mPEG-dithiocarbamate (DTC). Absorption spectroscopy confirmed DTC formation by the appearance of a doublet at 255 and 295 nm [Citation32]. The freshly prepared mPEG-DTC solution was then combined with 1 ml of colloidal Fe3O4 dispersed in water (8 mg ml–1) and incubated at room temperature for 1 h. Aliquots were removed and air-dried for analytical characterization, but otherwise used as-prepared in the next step.
2.2. Synthesis of magnetic gold nanoclusters (MGNCs)
A 0.25-ml aliquot of freshly prepared dispersion of mPEG-DTC-treated colloidal Fe3O4 (1 mg) was added to 4 ml of an aqueous solution of L-histidine (1 mg ml–1), adjusted to pH 5–6 using 0.1 M HCl, then incubated at room temperature for 1 h. In a separate container, a 0.6-ml aliquot of 1% w/v HAuCl4 solution was diluted with 14.8 ml deionized water, adjusted to pH 9–10 using 5 M NaOH, then combined with the colloidal Fe3O4–histidine solution with vortex mixing and allowed to sit for 20 min. The reaction mixture (now pH 8–9) was treated with 20 mM N-methylhydroxylamine (NMH) to initiate reduction, added in five 0.2-ml portions with mixing every 5 min, with a noticeable change in color by the third addition. MGNCs were generated in significant quantities after 2 h at room temperature, and fully formed after 12 h. The reaction gradually increased in acidity to a final range of pH 6–7.
As-produced MGNCs were separated by selective precipitation using a handheld NdFeB magnet producing linear field gradients of 1–3 kG cm–1, followed 15–20 min later by decantation of magnetically unresponsive materials. The retentate was subjected to two more rounds of redispersion into water at twice the original volume with mild sonication, followed by magnetic precipitation, to yield MGNCs that were essentially devoid of non-magnetic gold NPs.
Excess Fe3O4 was removed from MGNCs by treating aqueous suspensions with a 0.5 M solution of diethanol-DTC in methanol (DE-DTC; final concentration 2 mM), prepared in situ from diethanolamine and CS2 [Citation32]. In a typical cleansing procedure, 20 μl of 0.5 M DE-DTC was added to 5 ml of redispersed MGNCs (O.D. 0.4), followed by vortex mixing and mild sonication for several seconds, incubation for 1 h at room temperature, then two rounds of magnetic precipitation and redispersion in water.
3. Results and discussion
The co-precipitation of iron salts (commonly referred to as the Massart synthesis) is one of the simplest and most cost-effective approaches for preparing colloidal Fe3O4 [Citation33]. However, this method is known to be sensitive to multiple reaction parameters, and often produces colloidal Fe3O4 with a broad size polydispersity, making it less suitable for NP syntheses requiring strict size control. On the other hand, the Massart synthesis is ideal from the perspective of sustainable materials chemistry, as it generates no organic waste or toxic byproducts. In our hands, co-precipitation typically yielded polycrystalline aggregates of Fe3O4 with domain sizes of 5–6 nm, and we have found these to be a reliable feedstock in the preparation of magnetic gold nanoclusters.
Preliminary studies on the electroless deposition of Au onto colloidal Fe3O4 were inspired by the work of Gao and coworkers, who showed that metallization can be induced on surfaces coated with poly-L-histidine via hydroxylamine reduction under basic conditions [Citation11]. We find that the amino acid L-histidine also facilitates the reduction of HAuCl4 onto colloidal Fe3O4, but typically yields submicron composites in the absence of other surface-active agents (see below). After testing several different surface modifiers, we determined that treating colloidal Fe3O4 with mPEG-DTC (formed in situ from 5-kDa mPEG-NH2 and CS2 [Citation34,35]) provided the best control in MGNC synthesis, with average cluster sizes close to 100 nm (Figure ). Optical extinction spectroscopy indicated a broad plasmon resonance band with a maximum at 600 nm, but extending far into the near-infrared region. Previous electron diffraction analysis of MGNCs established the Au component to have an fcc structure [Citation29].
Figure 1. (a) Synthetic scheme for magnetic gold nanoclusters (MGNCs). (b) TEM image of clean MGNCs (inset: MGNC prior to DE-DTC treatment). (c) Optical extinction spectrum of MGNCs. (d) MGNCs before and after magnetic precipitation.
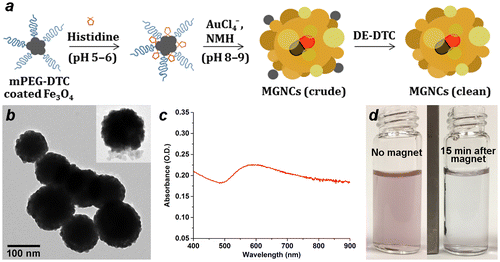
TEM images reveal that the MGNCs are often accompanied by significant amounts of extraneous inorganic material, presumed to be residual iron oxide. To remove this we turned to water-soluble dithiocarbamates (DTCs), which are well known for their chelation of transition-metal ions [Citation36], and for their chemisorption onto metal surfaces [Citation32,37]. DTCs have an especially high affinity for Fe2+ and Fe3+, suggesting utility as a digestive deferrating agent. This proved to be the case: treatment of as-prepared MGNC dispersions with DE-DTC, prepared in situ from a 2:1 mixture of diethanolamine and CS2 in methanol, removed all visible oxide from the MGNC surfaces and also from solution (Figure (b)). DE-DTC treatment does not introduce any notable changes to the optical or magnetic properties of the final dispersions, indicating that the MGNCs are uncompromised by the cleansing step. Likewise, attenuated total reflectance infrared (ATR-IR) spectroscopy indicates essentially no difference between MGNCs before and after treatment with DE-DTC (Figure ).
Figure 2. ATR-IR spectra acquired from pelleted samples after each step. Weak signals between 1900 and 2300 cm−1 are artifacts from instrumentation.
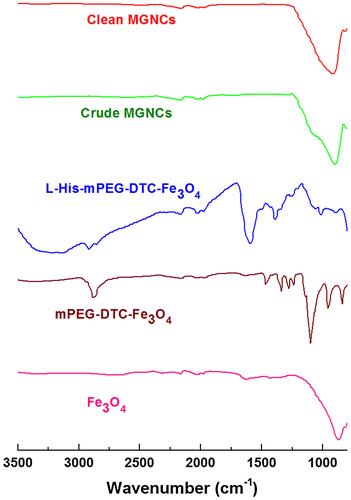
The MGNCs are isotropic with morphologies varying from roughened spheres to raspberry-like clusters, with the latter being most common. TEM analysis indicates a range of grain sizes within MGNCs between 8 and 25 nm; again, treatment with DE-DTC did not appear to have any significant impact on either MGNC morphology or grain size.
Earlier reports have shown that colloidal gold nanoparticles and nanoclusters can produce detectable levels of linear photoluminescence (PL) when excited at visible wavelengths, with emission intensities scaling roughly with particle volumes [Citation38,41]. Analysis by laser scanning confocal microscopy (λex 488 nm) yields a strong PL within the spectral window of 505–525 nm; however, no appreciable emissions are observed upon excitation at longer wavelengths (Figure ). This is in accord with the reported mechanism for PL, which is produced by plasmon-enhanced emissions from excited d-band holes within gold nanostructures [Citation38].
Figure 3. Laser scanning confocal fluorescence of MGNCs at different excitation wavelengths: (a) 488, (b) 546, (c) 647 nm. (d) Corresponding transmission image.
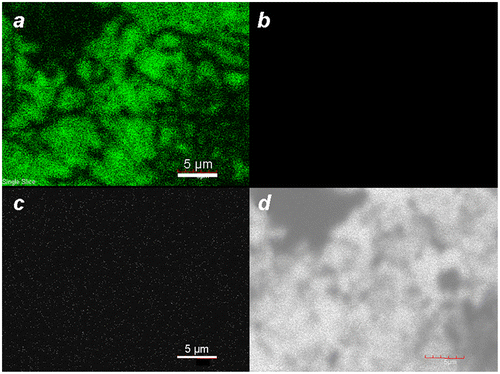
Control experiments illustrate the essential roles of both histidine and a peptizing agent for MGNC synthesis. Removal of either from the process results in the formation of small (10–12 nm) Au particles either loosely associated with iron-oxide nanoparticles, or embedded in a poorly dispersible oxide matrix (Figure ). A weakly coordinating ligand such as histidine is necessary to encourage the adsorption of gold ions onto Fe3O4 surfaces, while a strongly anchored dispersant encourages the dissociation of iron-oxide particles from the parent aggregate. While the precise role of mPEG-DTC remains unclear, replacing it with low molecular-weight DTCs does not produce the desired MGNCs. We note that the reaction is sensitive to histidine concentration (0.2 g ml–1 after dilution); too much prevents the deposition of Au onto colloidal Fe3O4, resulting instead in smaller, non-magnetic NPs. The pH of the Fe3O4–histidine solution is also important, as histidine is cationic at pH 5–6 and can adsorb in that form onto Fe3O4 surfaces.
Figure 4. TEM images of (a) Au nanoparticles produced without L-histidine treatment of mPEG-DTC-coated Fe3O4 NPs; (b) aggregates produced without mPEG-DTC treatment of histidine-coated Fe3O4 NPs. Bar = 50 nm.
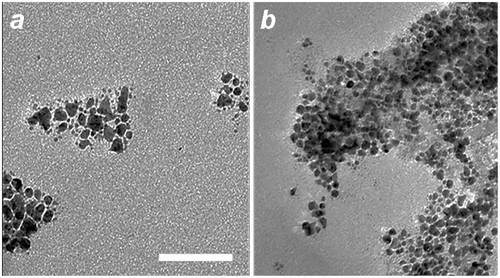
The MGNCs are responsive to field gradients produced by handheld NdFeB magnets, enabling their facile separation and decantation from magnetically inactive gold NPs within a 15–20 min period. The exact composition of the iron oxide is unknown but likely to be a mixture of Fe3O4 and γ-Fe2O3, the latter produced upon oxidation by Au ions. Several experiments were conducted to optimize the incorporation of FexOy in the MGNCs, in order to achieve higher magnetic moment. The mole ratio of gold to iron (Au/Fe) was varied to evaluate its effect on MGNC formation, size distribution, and magnetic response (Figure ). A mole ratio of 6–9 produced MGNCs of relatively uniform size and shape, but lower Au/Fe ratios had variable effects on MGNC structures and also produced greater amounts of residual iron oxide, to the extent that their removal was problematic. Conversely, Au/Fe ratios well above 9 produce considerable amounts of non-magnetic colloidal Au (Figure (d)), which is readily determined by the residual reddish tint in solution after magnetic precipitation.
Figure 5. MGNCs prepared with different Au/Fe mole ratios: (a) 2; (b) 4; (c) 9; (d) 27. Bar = 100 nm.
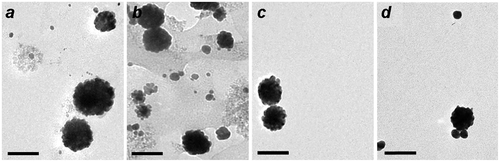
The effect of solution pH on MGNC formation was also evaluated at a fixed Au/Fe mole ratio of 9. Reductions performed under relatively acidic conditions (initial pH < 8) produces poorly dispersed MGNCs trapped in a matrix of amorphous iron oxide, whereas reactions performed at an initial pH of 8–9 produces well-dispersed MGNCs with a narrower size and shape polydispersity (Figure ). Reactions performed at pH > 9 generates very small particles and with poor dispersion stability.
Figure 6. MGNCs synthesized using a fixed Au/Fe mole ratio of 9, with different initial pH values: (a) 6–8; (b) 8–9; (c) 9–10. Bar = 50 nm.
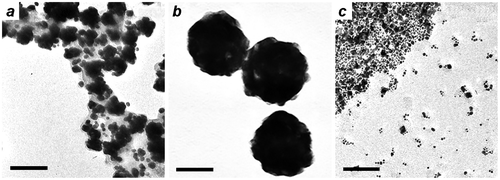
All reactions up to this point were performed on a small (1–5 ml) scale for the systematic evaluation of reaction conditions. To determine whether the optimized MGNC synthesis could be reproduced on a larger scale, the reaction was performed in bigger tubes (ca. 20 ml final volume; see Materials and methods), which produced MGNCs very similar in size as those made on a 1–5 ml scale (Figure ). The dry weight of MGNCs following removal of residual iron oxide (see below) was estimated to be 10 mg (42% yield). The reproducibility rate is 50%, which is acceptable given the heterogeneous nature of the colloidal Fe3O4 feedstock. Refinement of co-precipitation conditions may further improve the reproducibility of Au deposition onto colloidal Fe3O4 surfaces.
Figure 7. (a, b) TEM images of MGNCs prepared on a 20-ml scale, before and after cleansing with DE-DTC. (c) Size histogram of DTC-treated MGNCs by TEM image analysis (dav = 95 nm). (d) Hydrodynamic analysis of MGNCs by NTA (dh = 109 nm; standard error = 3 nm); Scale bar = 50 nm.
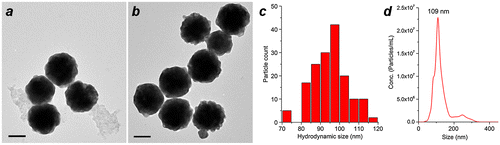
The size distribution of DE-DTC-treated MGNCs prepared on a 20-ml scale was characterized by TEM image analysis (d = 95 ± 9 nm; N = 161) and by nanoparticle tracking analysis (NTA; dh from 3 runs = 109 nm). The size analysis outcomes are similar and validate the accuracy of the latter method, in accord with other studies showing similar matches between TEM and NTA [Citation35,39].
Elemental analysis was performed using ICP-MS and AAS to determine the percentage and distribution of iron within the DTC-cleansed MGNCs. Despite an initial Au/Fe ratio of 9 in the reaction mixture, ICP-MS and AAS both show MGNCs to have much higher mole ratios (Au/Fe = 35.5 and 26.8, respectively), meaning that most of the starting Fe3O4 is not incorporated into the product. We presume that (a) the reduction of Au onto histidine-coated Fe3O4 is inefficient, relative to its autocatalytic deposition on Au islands formed subsequently, and (b) much iron oxide is lost by the speciation of dissolved Fe upon addition of AuCl4, which is a strong oxidant and a source of halide counterions.
EDX imaging was performed to determine the spatial distribution of Fe within the MGNCs. Low-resolution EDX–SEM imaging of MGNCs cleansed with DE-DTC confirmed colocalization of iron and gold within the MGNCs, but with a low content of Fe (Figure ). To determine whether a core–shell morphology might be present, a higher resolution analysis was performed on individual MGNCs in HAADF–STEM mode, prior to DE-DTC cleaning (Figure ). These images indicate a heterogeneous distribution of Fe with significant signal intensities within the MGNCs, but offer no evidence of a well-defined core-shell morphology.
Figure 8. EDX–SEM data for DTC-cleansed MGNCs. (a) Backscattering image (bar = 500 nm), (b–d) elemental mapping for Au (Mα1: 2,123 eV), Fe (Lα1,2: 705 eV), and merged Au/Fe respectively. (e) Linescan across MGNCs, confirming the colocalization of Au and Fe.
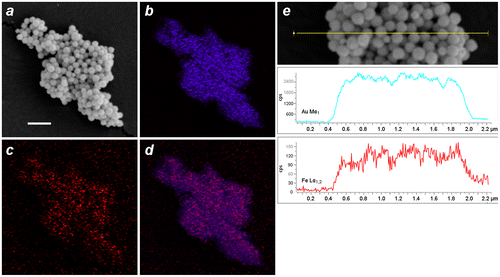
Figure 9. EDX data for MGNCs in HAADF–STEM mode, prior to cleansing by DE-DTC. (a) HAADF–STEM image (bar = 100 nm), (b–d) elemental maps for Au, Fe, and merged Au/Fe respectively. The distribution of Fe within MGNCs is relatively even.
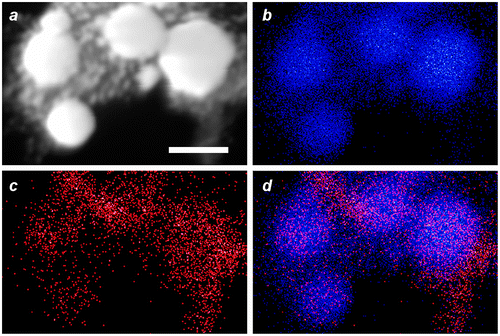
We postulate that Fe3O4 is entrapped by the rapid growth and coalescence of Au domains, resulting in composite Au nanoparticles containing clusters or veins of superparamagnetic iron oxide. We note that a similar deposition mechanism has been described in the formation of Au–Fe3O4 ‘nanoroses’, in which 5-nm Fe3O4 particles were used to nucleate the deposition and growth of Au clusters [Citation22]. Interestingly, EDX analyses from that study were also unable to confirm the existence of well-defined iron-oxide cores.
Despite the low Fe concentration, the MGNCs have sufficient moment to respond to local field gradients produced by rare-earth magnets (Figure (d)). The magnetic properties of MGNCs were characterized in powder form using a superconducting quantum interference device (SQUID) in vibrating sample magnetometry mode, with a field sweep of ± 10 kOe. Several MGNC samples were measured, yielding saturation magnetization (MS) values between 1.6 and 6.0 emu g–1 (Figure (a)). This variability in magnetization is to be expected, given the stochastic nature of Fe3O4 incorporation into the MGNCs during electroless deposition. On the one hand, this variability may limit the use of MGNCs in situations that require well-defined moment-to-mass ratios; on the other, many applications can tolerate a significant variation in magnetic moment, particularly those that require a magnetomotive function. It is worth mentioning that MGNCs are weakly ferromagnetic at room temperature, with HC on the order of 35 Oe (Figure (b)). The source of coercivity in MGNCs remains to be determined; however, we note that remanent magnetization has been observed in other magnetic gold NPs, including those without any ferromagnetic elements [Citation40].
4. Conclusions
A practical, eco-friendly synthesis of magnetic gold nanoclusters can be performed in aqueous alcohol without the use of harsh reagents, amphiphilic surfactants, or phase transfer from organic solvents. The final particles are produced with a narrow size distribution close to 100 nm, absorb strongly at NIR wavelengths, and can be collected within minutes using handheld magnets. EDX-SEM imaging supports the colocalization of Fe and Au within individual MGNCs. The MGNCs produce significant photoluminescence when excited at 488 nm. Lastly, ongoing studies have confirmed that MGNCs are highly biocompatible with cellular systems, and can thus be used to support a variety of bionanotechnology applications.
Disclosure statement
No potential conflict of interest was reported by the authors.
Funding
This work was supported by the Division of Civil, Mechanical and Manufacturing Innovation [grant number 1344654] and National Cancer Institute [grant number P30 CA023168].
References
- Mikhaylova M, Kim DK, Bobrysheva N, et al. Superparamagnetism of magnetite nanoparticles: dependence on surface modification. Langmuir. 2004;20:2472–2477.10.1021/la035648e
- Wang LY, Luo J, Fan Q, et al. Monodispersed core-shell Fe3O4@Au nanoparticles. J Phys Chem B. 2005;109:21593–21601.10.1021/jp0543429
- Mandal M, Kundu S, Ghosh SK, et al. Magnetite nanoparticles with tunable gold or silver shell. J Colloid Interface Sci. 2005;286:187–194.10.1016/j.jcis.2005.01.013
- Wang L, Bai J, Li Y, et al. Multifunctional nanoparticles displaying magnetization and near-IR absorption. Angew Chem Int Ed. 2008;47:2439–2442.10.1002/(ISSN)1521-3773
- Pal S, Morales M, Mukherjee P, et al. Synthesis and magnetic properties of gold coated iron oxide nanoparticles. J Appl Phys. 2009;105:07B504.10.1063/1.3059607
- Xu ZH, Li CX, Kang XJ, et al. Synthesis of a multifunctional nanocomposite with magnetic, mesoporous, and near-IR absorption properties. J Phys Chem C. 2010;114:16343–16350.10.1021/jp106325c
- Lim JK, Majetich SA, Tilton RD. Stabilization of superparamagnetic Iron oxide core-gold shell nanoparticles in high ionic strength media. Langmuir. 2009;25:13384–13393.10.1021/la9019734
- Smolensky ED, Neary MC, Zhou Y, et al. Fe3O4@Organic@Au: core-Shell nanocomposites with high saturation magnetisation as magnetoplasmonic MRI contrast agents. Chem Commun. 2011;47:2149–2151.10.1039/C0CC03746J
- Dong W, Li Y, Niu D, et al. Facile synthesis of monodisperse superparamagnetic Fe3O4 Core@Hybrid@Au shell nanocomposite for bimodal imaging and photothermal therapy. Adv Mater. 2011;23:5392–5397.10.1002/adma.201103521
- Meledandri CJ, Stolarczyk JK, Brougham DF. Hierarchical gold-decorated magnetic nanoparticle clusters with controlled size. ACS Nano. 2011;5:1747–1755.10.1021/nn102331c
- Jin Y, Jia C, Huang S-W, et al. Multifunctional nanoparticles as coupled contrast agents. Nat Commun. 2010;1:41.
- Yu H, Chen M, Rice PM, et al. Dumbbell-like Bifunctional Au-Fe3O4 nanoparticles. Nano Lett. 2005;5:379–382.10.1021/nl047955q
- Wei Y, Klajn R, Pinchuk AO, et al. Synthesis, shape control, and optical properties of hybrid Au/Fe3O4 "Nanoflowers". Small. 2008;4:1635–1639.10.1002/smll.v4:10
- Levin CS, Hofmann C, Ali TA, et al. Magnetic−plasmonic core−shell nanoparticles. ACS Nano. 2009;3:1379–1388.10.1021/nn900118a
- Wei Q, Song H-M, Leonov AP, et al. Gyromagnetic imaging: dynamic optical contrast using gold nanostars with magnetic cores. J Am Chem Soc. 2009;131:9728–9734.10.1021/ja901562j
- Song H-M, Wei Q, Ong QK, et al. Plasmon-resonant nanoparticles and nanostars with magnetic cores: synthesis and magnetomotive imaging. ACS Nano. 2010;4:5163–5173.10.1021/nn101202h
- Kim C, Song H-M, Cai X, et al. In Vivo photoacoustic mapping of lymphatic systems with plasmon-resonant nanostars. J Mater Chem. 2011;21:2841–2844.10.1039/c0jm04194g
- Miao X, Wang T, Chai F, et al. A facile synthetic route for the preparation of gold nanostars with magnetic cores and their reusable nanohybrid catalytic properties. Nanoscale. 2011;3:1189–1194.10.1039/c0nr00704h
- Bhana S, Rai BK, Mishra SR, et al. Synthesis and properties of near infrared absorbing magneto-optical nanopins. Nanoscale. 2012;4:4939–4942.10.1039/c2nr31291c
- Aaron JS, Oh J, Larson TA, et al. Increased optical contrast in imaging of epidermal growth factor receptor using magnetically actuated Hybrid Gold/Iron Oxide nanoparticles. Opt Express. 2006;14:12930–12943.10.1364/OE.14.012930
- Park H-Y, Schadt MJ, Wang L, et al. Fabrication of magnetic core@shell Fe Oxide@Au nanoparticles for interfacial bioactivity and bio-separation. Langmuir. 2007;23:9050–9056.10.1021/la701305f
- Ma LL, Feldman MD, Tam JM, et al. Small multifunctional nanoclusters (Nanoroses) for targeted cellular imaging and therapy. ACS Nano. 2009;3:2686–2696.10.1021/nn900440e
- Mohammad F, Balaji G, Weber A, et al. Influence of gold nanoshell on Hyperthermia of superparamagnetic Iron Oxide nanoparticles. J Phys Chem C. 2010;114:19194–19201.10.1021/jp105807r
- Xie H-Y, Zhen R, Wang B, et al. Fe3o4/Au core/shell nanoparticles modified with Ni2+-Nitrilotriacetic acid specific to Histidine-tagged proteins. J Phys Chem C. 2010;114:4825–4830.10.1021/jp910753f
- Wang CG, Chen J, Talavage T, et al. Gold Nanorod/Fe3O4 nanoparticle "Nano-pearl-necklaces" for simultaneous targeting, dual-mode imaging, and photothermal ablation of cancer cells. Angew Chem Int Edit. 2009;48:2759–2763.10.1002/anie.200805282
- Huang W-C, Tsai P-J, Chen Y-C. Multifunctional Fe3O4@Au nanoeggs as photothermal agents for selective killing of nosocomial and antibiotic-resistant bacteria. Small. 2009;5:51–56.10.1002/smll.v5:1
- Zhou X, Xu W, Wang Y, et al. Fabrication of cluster/shell Fe3O4/Au nanoparticles and application in protein detection via a SERS method. J Phys Chem C. 2010;114:19607–19613.10.1021/jp106949v
- Hu Y, Sun Y. Stable magnetic hot spots for simultaneous concentration and ultrasensitive surface-enhanced Raman scattering detection of solution analytes. J Phys Chem C. 2012;116:13329–13335.10.1021/jp303775m
- Kadasala NR, Wei A. Trace detection of tetrabromobisphenol A by SERS with DMAP-modified magnetic gold nanoclusters. Nanoscale. 2015;7:10931–10935.10.1039/C4NR07658C
- Kralisch D, Ott D, Gericke D. Rules and benefits of life cycle assessment in green chemical process and synthesis design: a tutorial review. Green Chem. 2015;17:123–145.10.1039/C4GC01153H
- Batis-Landoulsi H, Vergnon P. Magnetic moment of γ-Fe2O3 microcrystals: morphological and size effect. J Mater Sci. 1983;18:3399–3403.10.1007/BF00544166
- Zhu H, Coleman DM, Dehen CJ, et al. Assembly of Dithiocarbamate-anchored monolayers on gold surfaces in aqueous solutions. Langmuir. 2008;24:8660–8666.10.1021/la801254b
- Massart R. Preparation of aqueous magnetic liquids in alkaline and acidic media. IEEE Trans Magn. 1981;17:1247–1248.10.1109/TMAG.1981.1061188
- Huff TB, Hansen MN, Zhao Y, et al. Controlling the cellular uptake of gold nanorods. Langmuir. 2007;23:1596–1599.10.1021/la062642r
- Mehtala JG, Wei A. Nanometric resolution in the hydrodynamic size analysis of ligand-stabilized gold nanorods. Langmuir. 2014;30:13737–13743.10.1021/la502955h
- Hogarth, G. Transition-metal dithiocarbamates: 1978-2003. In: Karlin KD, editor. Progress in Inorganic Chemistry. 53. New York: John Wiley and Sons; 2005. p. 71–561.
- Zhao Y, Pérez-Segarra W, Shi Q, et al. Dithiocarbamate assembly on gold. J Am Chem Soc. 2005;127:7328–7329.10.1021/ja050432f
- Dulkeith E, Niedereichholz T, Klar TA, et al. Plasmon emission in photoexcited gold nanoparticles. Phys Rev B. 2004;70:205424.10.1103/PhysRevB.70.205424
- Park J, Kadasala NR, Abouelmagd SA, et al. Polymer–iron oxide composite nanoparticles for EPR-independent drug delivery. Biomaterials. 2016;101:285–295.10.1016/j.biomaterials.2016.06.007
- Nealon GL, Donnio B, Greget R, et al. Magnetism in gold nanoparticles. Nanoscale. 2012;4:5244–5258.
- Su YH, Tu S-L, Tseng S-W, et al. Influence of surface plasmon resonance on the emission intermittency of photoluminescence from gold nano-sea-urchins. Nanoscale. 2010;2:2639–2646.10.1039/c0nr00330a