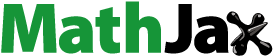
ABSTRACT
Quadruple perovskite oxides AA′3B4O12 demonstrate a rich variety of structural and electronic properties. A large number of constituent elements for A/A′/B-site cations can be introduced using the ultra-high-pressure synthesis method. Development of novel functional materials consisting of earth-abundant elements plays a crucial role in current materials science. In this paper, functional properties, especially oxygen reaction catalysis, for quadruple perovskite oxides CaCu3Fe4O12 and AMn7O12 (A = Ca, La) composed of earth-abundant elements are reviewed.
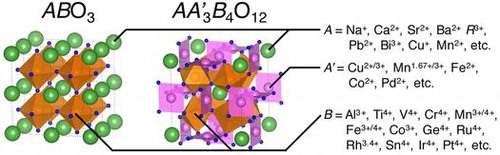
1. Introduction
Perovskite oxides ABO3 (A = alkaline, alkaline-earth and rare-earth metal ions; B = d-block transition metals) have been extensively investigated as promising functional materials in the past decades [Citation1–3]. A large number of perovskite and related materials were synthesized and their properties were exhaustively illustrated. Partial chemical substitutions of A- and/or B-sites for ABO3-type perovskite and related structures drive structural and electronic transformations, leading to functional properties such as large magnetoresistance and high-temperature superconductivity [Citation4,5]. A couple of different metal ions occupying the identical crystallographic sites make spatial ordering of atoms, crystallizing in ordered perovskite structures with multiplied chemical formulae such as A2BB′O6, AA′B2O6 and AA′3B4O12. Sr2FeMoO6 is a double perovskite consisting of two B-site ions, Fe3+ and Mo5+, in which a significant difference in valence states results in rock-salt type ordering of these two ions [Citation6]. YBaMn2O6 is another type of double perovskites with layered ordering of A-site ions Y3+ and Ba2+ [Citation7]. The quadruple perovskite oxide family AA′3B4O12 is derived from 1:3-type A-site cation ordering; one quarter (A) is occupied by conventional A-site ions in icosahedral coordination, the remaining three quarters by transition metal ions in pseudosquare-planar coordination (Figure ). The quadruple perovskite oxide family was discovered by Bochu et al. in the 1970s [Citation8,9], and has now been intensively investigated because of a lot of syntheses of new compounds using ultra high pressures above 10 GPa. Combinations of A-, A′- and B-site ions provide unexpected properties, some of which are functional. In this paper, recent advances on the catalytic properties of the quadruple perovskite oxides, especially those consisting of earth-abundant elements, are reviewed.
2. Quadruple perovskite oxides
2.1. Syntheses, compositions and crystal structures
Most quadruple perovskite oxides AA′3B4O12 crystallize in the cubic symmetry of Im space group (No. 204), containing smaller transition metal ions at pseudosquare-coordinated A′-sites. High-pressure syntheses under several GPa are usually adopted to stabilize smaller A′-site ions at sites with an originally high coordination number of 12. Ultra-high-pressure syntheses above 10 GPa efficiently expand the variety of A′-site cations. Together with Jahn-Teller active ions with 3d4 and 3d9 electron configurations Mn3+ and Cu2+, respectively [Citation8,9], non-Jahn-Teller ions such as Mn1.67+, Fe2+, Co2+, Cu3+ and Pd2+ can be incorporated [Citation10–14]. Tri- and tetra-valent B-site cations in this series are reported: Al3+, Ti4+, V4+, Cr3+/4+, Mn3+/4+, Fe3+/4+, Co3+, Ge4+, Ru4+, Rh3.4+, Sn4+, Ir4+ and Pt4+ [Citation9,15–23]. The icosahedral A-sites are occupied by conventional alkaline (Na+, K+), alkaline-earth (Ca2+, Sr2+, Ba2+) and rare-earth (R3+: R = La–Lu, Ce4+) metal ions, and also by Cd2+, Pb2+, Th4+, Bi3+, Ag+ [Citation24–26]. Unusual A-site ions in this series are Mn2+ and Cu+ ions for ACu3V4O12 [Citation27,28], and the most remarkable example is ζ-Mn2O3 (MnMn3Mn4O12) [Citation29]. Recently, quadruple perovskite PbCoO3 (Pb2+Pb4+3Co2+2Co3+2O12), in which both A- and B-site aliovalent ions are simultaneously ordered, has been reported [Citation30]. Several examples with B-site ordering of a different kind of cations in Pn
symmetry (space group No. 201) are also known: CaCu3Ga2Sb2O12, CaCu3Cr2Sb2O12 and CaCu3Fe2B′2O12 (B′ = Re, Sb, Nb, Os) [Citation31–37].
2.2. Electronic properties
Electronic properties of quadruple perovskite oxides have been widely investigated. CaCu3Ti4O12 is the best-known quadruple perovskite and is investigated as a dielectric material owing to its large permittivity [Citation38,39]. (Ca/La)Cu3Mn4O12 exhibits a room-temperature magnetoresistance in low magnetic fields [Citation40,41]. CaCu3Ru4O12 and CaCu3Ir4O12 show enhanced electron mass, like a heavy fermion [Citation42,43]. NaMn7O12 displays sequential charge/spin/orbital orderings [Citation44]. These properties are summarized in the review [Citation45].
The above-mentioned properties are mainly attributed to electronic interactions between A′- and B-site metals. A striking feature of A′–B site electronic interactions is intermetallic charge transfer reported for the ACu3Fe4O12 system. A temperature-induced A′-to-B electron charge transfer from Cu to Fe (3Cu2+ + 4Fe3.75+ → 3Cu3+ +4Fe3+) occurs at ~400 K for LaCu3Fe4O12 [Citation13], although a charge disproportionation (2Fe4+ → Fe3+ +Fe5+) below 210 K was reported for Ca-analogue CaCu3Fe4O12 previously [Citation17]. Later, an inverse B-to-A′ electron charge transfer from Fe to Cu simultaneously with the charge disproportionation was demonstrated for CaCu3Fe4O12 [Citation46], ensuring the bidirectionality of A′-B intersite charge transfer. The charge-disproportionation/transfer transitions are abruptly switched by bond strains on R–O (R: rare-earth metals) and Fe–O bonds for RCu3Fe4O12 [Citation47,48], evidencing multiple competing electronic states in ACu3Fe4O12 series [Citation49].
SrCu3Fe4O12 displays a second-order continuous Cu-to-Fe electron charge transfer associated with a negative thermal expansion in the temperature range between ~200 and ~270 K [Citation50–52]. More recently, adjustment of thermal expansion properties, that is, relaxations from first- to second-order charge transfer resulting in negative or near-zero thermal expansions in moderate temperature ranges were found for Mn-doped LaCu3Fe4O12 (Figure ) [Citation53]. Further, precise control of the thermal expansion coefficient and operation temperature range of negative/zero thermal expansion is achieved for Mn-doped SrCu3Fe4O12 (Figure ) [Citation54].
Figure 2. Temperature dependence of the unit cell volume (a) and differential scanning calorimetry curves (b) of LaCu3Fe4–xMnxO12 (x = 0, 0.5, 0.75, 1, and 1.5). Reproduced from [Citation53] with permission from AIP Publishing LLC.
![Figure 2. Temperature dependence of the unit cell volume (a) and differential scanning calorimetry curves (b) of LaCu3Fe4–xMnxO12 (x = 0, 0.5, 0.75, 1, and 1.5). Reproduced from [Citation53] with permission from AIP Publishing LLC.](/cms/asset/afc7881b-95d1-40c4-99e4-3dd137d44ce8/tsta_a_1350557_f0002_oc.gif)
Figure 3. Temperature dependence of the cubic a-axis length for SrCu3Fe4–xMnxO12 (x = 0, 0.5, 1, 1.25, 1.5, and 1.75). Reprinted from [Citation54] with permission from AIP Publishing LLC.
![Figure 3. Temperature dependence of the cubic a-axis length for SrCu3Fe4–xMnxO12 (x = 0, 0.5, 1, 1.25, 1.5, and 1.75). Reprinted from [Citation54] with permission from AIP Publishing LLC.](/cms/asset/1bf84085-28d6-496e-801d-46bb5f7ebd4a/tsta_a_1350557_f0003_oc.gif)
2.3. Catalytic properties
Recently, catalytic properties of quadruple perovskite oxides synthesized under high pressure were reported. Fe4+-containing perovskites, CaFeO3, SrFeO3 and CaCu3Fe4O12 display catalytic activities for the oxygen evolution reaction (OER; 4OH– → O2+2H2O+4e– in alkaline conditions). These are comparable to or exceed the activities of state-of-the-art OER catalysts such as Ba0.5Sr0.5Co0.8Fe0.2O3–δ (BSCF) [Citation55] and RuO2 (Figure ). OER plays a crucial role in energy conversions such as water splitting and recharge of metal–air batteries, as well as the importance of the oxygen reduction reaction (ORR; O2+2H2O+4e– → 4OH– in alkaline conditions) for fuel cell operations [Citation56–58]. CaCu3Fe4O12 is a promising OER catalyst because of the earth-abundant constituent elements (Ca, Cu and Fe).
Figure 4. Linear sweep voltammograms in OER conditions for perovskite oxide and RuO2 catalysts. Reproduced from [Citation55].
![Figure 4. Linear sweep voltammograms in OER conditions for perovskite oxide and RuO2 catalysts. Reproduced from [Citation55].](/cms/asset/2027af4e-626c-4a95-ada3-58764b037ae1/tsta_a_1350557_f0004_oc.gif)
The origin of highly active OER catalysis of Fe4+-perovskites could be explained by two factors: metal–oxygen covalency and electronic states. Suntivich et al. [Citation59] exhibited a volcano-shaped plot of OER catalytic activity for ABO3-type perovskites, in which the activity is highest in the vicinity of the eg~1.2 orbital occupancy of B-site ions. Furthermore, Grimaud et al. [Citation60] reported that OER activities of Co-containing double perovskites LnBaCo2O6–δ (Ln = lanthanide metals) are related to the energy levels of oxygen 2p band center; the OER activity is enhanced when oxygen 2p band center is moderately close to the Fermi level. These tendencies are explained by the OER reaction model in which charge transfer from adsorbed reactants and intermediates to transition metal ions (active sites) of a catalyst is facilitated to decrease the energy barrier of a rate-determining step when the metal–oxygen covalency becomes strong [Citation61]. The unusual high valence ions (Fe4+) may form strong covalency with oxygen ions [Citation62], thus the high OER activity is expected for Fe4+-perovskites.
More interestingly, CaCu3Fe4O12 is more active for OER than simple perovskite counterparts. Since high OER activity is not known for cupric oxides, the Cu ions incorporated into A′-sites are not expected to contribute to the OER activity. In addition, synergistic effects between Cu and Fe do not seem to be achieved because another Cu-Fe complex oxide (spinel-type CuFe2O4) has a lower activity. Crystal structure analysis suggests that heavily tilted Fe–O–Fe bonds make the neighboring adsorbates close enough to interact, possibly enabling two active-site reaction mechanisms (Figure ). This mechanism is different from conventional single-active-site reactions for simple perovskite catalysts and is expected to avoid rate-determining steps of conventional mechanisms [Citation59].
Figure 5. (Top) OH− adsorbates on FeO2-terminated (100) planes of CaCu3Fe4O12. The interatomic distance between the nearest neighboring OH adsorbates is ∼2.6 A. (Bottom) Proposed OER reaction mechanism for CaCu3Fe4O12. Reproduced from [Citation55].
![Figure 5. (Top) OH− adsorbates on FeO2-terminated (100) planes of CaCu3Fe4O12. The interatomic distance between the nearest neighboring OH adsorbates is ∼2.6 A. (Bottom) Proposed OER reaction mechanism for CaCu3Fe4O12. Reproduced from [Citation55].](/cms/asset/17635599-8e78-4f7f-8353-cc6916f0524f/tsta_a_1350557_f0005_oc.gif)
Another remarkable feature of CaCu3Fe4O12 catalyst is durability in OER conditions. The simple perovskites CaFeO3 and SrFeO3 were readily degraded during 100 sequential OER measurements because of progressive surface amorphization (Figure ). In contrast, CaCu3Fe4O12 retained the initial OER activity after 100 OER measurements. The enhancement of stability for CaCu3Fe4O12 is attributed to the widespread covalent bonding network consisting of Cu–O and Fe–O bonds. Figure illustrates the electron density distributions of SrFeO3 and CaCu3Fe4O12 obtained from Rietveld refinement and maximum entropy method analysis of synchrotron X-ray diffraction data. It is expected that highly ionic Sr ions with electronic isolation from coordinating oxygen ions are easily dissolved in the electrolyte for SrFeO3. On the other hand, CaCu3Fe4O12 has a widespread covalent bonding network in which pseudosquare-planar CuO4 and octahedral FeO6 units are electronically connected. The degradation of perovskite oxide OER catalysts is an essential issue [Citation60,63,64] but efficient strategies have not been established yet. This finding provides a new design principle for highly active and robust catalysts for OER.
Figure 6. Cyclic voltammograms of SrFeO3, CaFeO3, and CaCu3Fe4O12 for 100 sequential OER measurements. Reproduced from [Citation55].
![Figure 6. Cyclic voltammograms of SrFeO3, CaFeO3, and CaCu3Fe4O12 for 100 sequential OER measurements. Reproduced from [Citation55].](/cms/asset/e0705641-844b-400e-af7d-1cbce75054b5/tsta_a_1350557_f0006_oc.gif)
Figure 7. (Left) Crystal structures and (right) electron density maps of SrFeO3 (equal-density level: 0.4 Å−3) and CaCu3Fe4O12 (equal-density level: 0.5 Å−3). Reproduced from [Citation55].
![Figure 7. (Left) Crystal structures and (right) electron density maps of SrFeO3 (equal-density level: 0.4 Å−3) and CaCu3Fe4O12 (equal-density level: 0.5 Å−3). Reproduced from [Citation55].](/cms/asset/f93fcf05-5b71-4e13-a8d7-7bb6ad8d3945/tsta_a_1350557_f0007_oc.gif)
To elucidate A′-site cation contributions to a reaction mechanism, comparison of simple and quadruple perovskite catalysts with identical elements/ions was conducted [Citation65]. Since Mn-based perovskites AMnO3 and AMn7O12 (A = Ca, La) consist of identical elements (Ca, Mn, O) for A = Ca or identical ions (La3+, Mn3+, O2–), compositional differences are suppressed, compared with the above-described couple (CaFeO3 and CaCu3Fe4O12). Figure shows linear sweep voltammograms of Mn-perovskites in OER conditions. There are clear differences in catalytic activity between AMnO3 and AMn7O12 series, namely, overpotentials are lower by 0.1 V and specific activities at 1.7 V versus reversible hydrogen electrode (RHE) are about thirtyfold for AMn7O12. This observation implies higher catalytic activities of quadruple perovskite oxides are derived not from electronic factors but structural effects altering reaction mechanisms. Indeed, first-principle calculations do not explain the origin of increased OER activity for quadruple manganese perovskites. In contrast, structure analysis based on Rietveld refinement of synchrotron X-ray diffraction data suggests a structure-activity relationship for Mn-oxide catalysts. Figure shows the specific activity versus average Mn–Mn distances for various manganese oxide catalysts including corner/edge-shared Mn–O polyhedral units, in analogy with the Mn–Mn distance dependence of photocatalytic turnover frequency for various manganese oxides [Citation66]. The specific activity is enhanced as the Mn–Mn distance increases from ~3 to ~3.2 Å, although poorly active for longer Mn–Mn distances (~3.8 Å). This feature is explained by possible O–O bond formation for a two-site reaction mechanism. Figure illustrates the proposed OER mechanism for quadruple perovskite catalysts, in which the two neighboring active sites (A′- and B-site Mn atoms) are close enough to connect their adsorbates. Rate-determining steps including O–O bond formation are avoided in this mechanism [Citation59]. AMn7O12 catalysts also catalytically active for ORR, as well as most manganese oxides (Figure ). Thus, AMn7O12 perovskites are bifunctional catalysts for oxygen reactions (OER and ORR).
Figure 8. Linear sweep voltammograms in OER conditions for AMnO3, AMn7O12 (A = Ca, La), and RuO2. The inset illustrates the enlarged data in the vicinity of the current density onset. Reproduced from [Citation65] with permission from John Wiley & Sons.
![Figure 8. Linear sweep voltammograms in OER conditions for AMnO3, AMn7O12 (A = Ca, La), and RuO2. The inset illustrates the enlarged data in the vicinity of the current density onset. Reproduced from [Citation65] with permission from John Wiley & Sons.](/cms/asset/9ceec3bd-2738-4cd6-b170-7ee515867e3d/tsta_a_1350557_f0008_oc.gif)
Figure 9. The specific activities versus average Mn–Mn intermetallic distances calculated for edge-shared MnO6 octahedra (Mn2O3, Mn3O4), corner-shared MnO4 pseudosquare plane–MnO6 octahedron (CaMn7O12, LaMn7O12), and corner-shared MnO6 octahedra (CaMnO3, LaMnO3). Reproduced from [Citation65] with permission from John Wiley & Sons.
![Figure 9. The specific activities versus average Mn–Mn intermetallic distances calculated for edge-shared MnO6 octahedra (Mn2O3, Mn3O4), corner-shared MnO4 pseudosquare plane–MnO6 octahedron (CaMn7O12, LaMn7O12), and corner-shared MnO6 octahedra (CaMnO3, LaMnO3). Reproduced from [Citation65] with permission from John Wiley & Sons.](/cms/asset/001b2ae3-d692-46fd-b759-4c85684d12ea/tsta_a_1350557_f0009_oc.gif)
Figure 10. Proposed OER mechanism for LaMn7O12 via direct O–O bond formation between the unsaturated MnO4 plane (green, right triangle) and the unsaturated MnO6 octahedron (orange, left triangle). Reproduced from [Citation65] with permission from John Wiley & Sons.
![Figure 10. Proposed OER mechanism for LaMn7O12 via direct O–O bond formation between the unsaturated MnO4 plane (green, right triangle) and the unsaturated MnO6 octahedron (orange, left triangle). Reproduced from [Citation65] with permission from John Wiley & Sons.](/cms/asset/a67d29ea-964e-4162-aac5-1c151ecf70d2/tsta_a_1350557_f0010_oc.gif)
Figure 11. Catalytic activity of manganese perovskites obtained by using a rotating ring/disk electrode equipment. Disk/ring current densities are plotted as a function of applied disk potential in ORR conditions for AMnO3, AMn7O12 (A = Ca, La), and reference catalysts (acetlylene black (AB), Platinum-carbon composite (Pt/C)). Reproduced from [Citation65] with permission from John Wiley & Sons.
![Figure 11. Catalytic activity of manganese perovskites obtained by using a rotating ring/disk electrode equipment. Disk/ring current densities are plotted as a function of applied disk potential in ORR conditions for AMnO3, AMn7O12 (A = Ca, La), and reference catalysts (acetlylene black (AB), Platinum-carbon composite (Pt/C)). Reproduced from [Citation65] with permission from John Wiley & Sons.](/cms/asset/62206d8c-2149-4756-bb18-a35c3a5ff628/tsta_a_1350557_f0011_oc.gif)
3. Conclusions
Recent advances on novel functional properties for quadruple perovskite oxides are reviewed. Highly active oxygen reaction catalysis for quadruple perovskite oxides consisting of earth-abundant elements demonstrates that utilization of ultra-high-pressure synthesis facilitates development of novel functional materials. A great number of still-unexplored compounds synthesized under high pressure also remain promising candidates for functional materials. Thus, further investigation of high-pressure-synthesized materials can give new impetus to materials science.
Disclosure statement
No potential conflict of interest was reported by the author.
Funding
This work was supported by Grants-in-Aid for Scientific Research [16H04220; 16H02393] from the Japan Society for the Promotion of Science, Toray Science Foundation, and Kyoto Technoscience Center.
References
- Mitchell RH. Perovskites: modern and ancient. Thunder Bay: Almaz Press; 2002.
- Tilley RJD. Perovskites: structure-property relationships. New York, NY: John Wiley & Sons; 2016.10.1002/9781118935651
- Labhasetwar N, Saravanan G, Kumar Megarajan S, et al. Perovskite-type catalytic materials for environmental applications. Sci Technol Adv Mater. 2015;16:036002.10.1088/1468-6996/16/3/036002
- Bednorz JG, Muller KA. Possible high-Tc superconductivity in the Ba-La-Cu-O system. Z Phys B. 1986;64:189–193.10.1007/BF01303701
- Urushibara A, Moritomo Y, Arima T, et al. Insulator-metal transition and giant magnetoresistance in La1-xSrxMnO3. Phys Rev B. 1995;51:14103–14109.10.1103/PhysRevB.51.14103
- Kobayashi KI, Kimura T, Sawada H, et al. Room-temperature magnetoresistance in an oxide material with an ordered double-Perovskite structure. Nature. 1998;395:677–680.
- Nakajima T, Kageyama H, Yoshizawa H, et al. Structures and electromagnetic properties of new metal-ordered manganites: RBaMn2O6 (R=Y and Rare-Earth Elements). J Phys Soc Jpn. 2002;71:2843–2846.10.1143/JPSJ.71.2843
- Bochu B, Chenavas J, Joubert JC, et al. High pressure synthesis and crystal structure of a new series of Perovskite-like compounds CMn7O12 (C=Na, Ca, Cd, Sr, La, Nd). J Solid State Chem. 1974;11:88–93.10.1016/0022-4596(74)90102-9
- Chenavas J, Joubert JC, Marezio M, et al. The synthesis and crystal structure of CaCu3Mn4O12: A new Ferromagnetic-Perovskite-like compound. J Solid State Chem. 1975;14:25–32.10.1016/0022-4596(75)90358-8
- Leinenweber K, Linton J, Navrotsky A, et al. High-pressure Perovskites on the join CaTiO3-FeTiO3. Phys Chem Miner. 1995;22:251–258.
- Shiro K, Yamada I, Ikeda N, et al. Pd2+-incorporated Perovskite CaPd3B4O12 (B=Ti, V). Inorg Chem. 2013;52:1604–1609.10.1021/ic3025155
- Ovsyannikov SV, Zainulin YG, Kadyrova NI, et al. New Antiferromagnetic Perovskite CaCo3V4O12 prepared at high-pressure and high-temperature conditions. Inorg Chem. 2013;52:11703–11710.10.1021/ic400649 h
- Long YW, Hayashi N, Saito T, et al. Temperature-induced A-B intersite charge transfer in an A-site-ordered LaCu3Fe4O12 Perovskite. Nature. 2009;458:60–63.10.1038/nature07816
- Long Y, Saito T, Mizumaki M, et al. Various valence states of square-coordinated Mn in A-site-ordered Perovskites. J Am Chem Soc. 2009;131:16244–16247.10.1021/ja906668c
- Kadyrova NI, Zakharova GS, Zainulin YG, et al. Synthesis and properties of the new compounds NaCu3V4O12 and CaCu3V4O12 obtained under uniform compression. Doklady Chemistry. 2003;392:251–253.10.1023/A:1026126110138
- Subramanian MA, Marshall WJ, Calvarese TG, et al. Valence degeneracy in CaCu3Cr4O12. J Phys Chem Solids. 2003;64:1569–1571.10.1016/S0022-3697(03)00095-7
- Yamada I, Takata K, Hayashi N, et al. Perovskite containing quadrivalent iron as a charge-disproportionated ferrimagnet. Angew Chem Int Ed. 2008;47:7032–7035.10.1002/anie.v47:37
- Yamada I, Ishiwata S, Terasaki I, et al. Synthesis, structure, and physical properties of A-site ordered Perovskites ACu3Co4O12 (A=Ca and Y). Chem Mater. 2010;22:5328–5332.10.1021/cm101671j
- Shiraki H, Saito T, Yamada T, et al. Ferromagnetic cuprates CaCu3Ge4O12 and CaCu3Sn4O12 with A-site ordered Perovskite structure. Phys Rev B. 2007;76:140403.
- Labeau M, Bochu B, Joubert JC, et al. Synthèse et caractérisation cristallographique et physique d'une série de composés ACu3Ru4O12 de type perovskite [Synthesis and crystallographic and physical characterization of a series of perovskite type ACu3Ru4O12 compounds]. J Solid State Chem. 1980;33:257–261.10.1016/0022-4596(80)90127-9
- Yamada I, Ochi M, Mizumaki M, et al. High-pressure synthesis, crystal structure, and unusual valence state of Novel Perovskite oxide CaCu3Rh4O12. Inorg Chem. 2014;53:7089–7091.10.1021/ic501341x
- Cheng JG, Zhou JS, Yang YF, et al. Possible Kondo physics near a metal-insulator crossover in the A-Site ordered Perovskite CaCu3Ir4O12. Phys Rev Lett. 2013;111:176403.10.1103/PhysRevLett.111.176403
- Tohyama T, Saito T, Mizumaki M, et al. Antiferromagnetic interaction between A'-Site Mn Spins in A-site-ordered Perovskite YMn3Al4O12. Inorg Chem. 2010;49:2492–2495.10.1021/ic9024876
- A Collomb, D Samaras, B Bochu, et al. Magnetic structures of some [AC3](B4)O12 compounds with a Perovskite-like structure. Physica B+C 1977;86–88, Part 2:927-928.
- Takata K, Yamada I, Azuma M, et al. Magnetoresistance and electronic structure of the half-metallic ferrimagnet BiCu3Mn4O12. Phys Rev B. 2007;76:024429.
- Akizuki Y, Yamada I, Fujita K, et al. AgCu3V4O12: A Novel Perovskite containing mixed-valence silver ions. Inorg Chem. 2013;52:13824–13826.10.1021/ic402579v
- Akizuki Y, Yamada I, Fujita K, et al. A-site-ordered Perovskite MnCu3V4O12 with a 12-coordinated manganese(II). Inorg Chem. 2013;52:11538–11543.10.1021/ic401855j
- Akizuki Y, Yamada I, Fujita K, et al. Rattling in the quadruple Perovskite CuCu3V4O12. Angew Chem Int Ed. 2015;54:10870–10874.10.1002/anie.201504784
- Ovsyannikov SV, Abakumov AM, Tsirlin AA, et al. Perovskite-like Mn2O3: a path to new manganites. Angew Chem Int Ed. 2013;52:1494–1498.10.1002/anie.201208553
- Sakai Y, Yang J, Yu R, et al. A-Site and B-Site charge orderings in an s-d level controlled Perovskite oxide PbCoO3. J Am Chem Soc. 2017;139:4574–4581.10.1021/jacs.7b01851
- Chen WT, Mizumaki M, Seki H, et al. A half-metallic A- and B-site-ordered quadruple Perovskite oxide CaCu3Fe2Re2O12 with large magnetization and a high transition temperature. Nat Commun. 2014;5:3909.
- Senn MS, Chen W-T, Saito T, et al. B-Cation order control of magnetism in the 1322 Perovskite CaCu3Fe2Nb2O12. Chem Mater. 2014;26:4832–4837.10.1021/cm502064b
- Byeon S-H, Lee S-S, Parise JB, et al. New Ferrimagnetic oxide CaCu3Cr2Sb2O12: high-pressure synthesis, structure, and magnetic properties. Chem Mater. 2005;17:3552–3557.10.1021/cm050397b
- Byeon S-H, Lufaso MW, Parise JB, et al. T high-pressure synthesis and characterization of Perovskites with simultaneous ordering of both the A- and B-Site cations, CaCu3Ga2M2O12 (M=Sb, Ta). Chem Mater. 2003;15:3798–3804.10.1021/cm034318c
- Yin Y-Y, Liu M, Dai J-H, et al. LaMn3Ni2Mn2O12: An A- and B-Site ordered Quadruple Perovskite with A-Site tuning orthogonal spin ordering. Chem Mater. 2016;28:8988–8996.10.1021/acs.chemmater.6b03785
- Larregola SA, Zhou J, Alonso JA, et al. New routes to synthesizing an ordered Perovskite CaCu3Fe2Sb2O12 and its magnetic structure by neutron powder diffraction. Inorg Chem. 2014;53:4281–4283.10.1021/ic500458 m
- Deng H, Liu M, Dai J, et al. Strong enhancement of spin ordering by A-site magnetic ions in the ferrimagnet CaCu3Fe2Os2O12. Phys Rev B. 2016;94:024414.10.1103/PhysRevB.94.024414
- Subramanian MA, Li D, Duan N, et al. High dielectric constant in ACu3Ti4O12 and ACu3Ti3FeO12 phases. J Solid State Chem. 2000;151:323–325.10.1006/jssc.2000.8703
- Ramirez AP, Subramanian MA, Gardel M, et al. Giant dielectric constant response in a copper-titanate. Solid State Commun. 2000;115:217–220.10.1016/S0038-1098(00)00182-4
- Zeng Z, Greenblatt M, Subramanian MA, et al. Large low-field magnetoresistance in perovskite-type CaCu3Mn4O12 without double exchange. Phys Rev Lett. 1999;82:3164–3167.10.1103/PhysRevLett.82.3164
- Alonso JA, Sanchez-Benitez J, De Andres A, et al. Enhanced magnetoresistance in the complex perovskite LaCu3Mn4O12. Appl Phys Lett. 2003;83:2623–2625.10.1063/1.1611647
- Kobayashi W, Terasaki I, Takeya J-I, et al. Novel heavy-fermion state in CaCu3Ru4O12. J Phys Soc Jpn. 2004;73:2373–2376.10.1143/JPSJ.73.2373
- Xin Y, Zhou HD, Cheng JG, et al. Study of atomic structure and electronic structure of an AA'3B4O12 double-perovskite CaCu3Ir4O12 using STEM imaging and EELS techniques. Ultramicroscopy. 2013;127:94–99.10.1016/j.ultramic.2012.07.019
- Prodi A, Gilioli E, Gauzzi A, et al. Charge, orbital and spin ordering phenomena in the mixed valence manganite (NaMn3+3)(Mn3+2Mn4+2)O12. Nature Mater. 2004;3:48–52.10.1038/nmat1038
- Vasil’ev AN, Volkova OS. New functional materials AC3B4O12. Low Temp Phys. 2007;33:895.10.1063/1.2747047
- Yamada I, Murakami M, Hayashi N, et al. Inverse charge transfer in the Quadruple Perovskite CaCu3Fe4O12. Inorg Chem. 2016;55:1715–1719.10.1021/acs.inorgchem.5b02623
- Yamada I, Etani H, Tsuchida K, et al. Control of bond-strain-induced electronic phase transitions in iron perovskites. Inorg Chem. 2013;52:13751–13761.10.1021/ic402344 m
- Etani H, Yamada I, Ohgushi K, et al. Suppression of intersite charge transfer in charge-disproportionated perovskite YCu3Fe4O12. J Am Chem Soc. 2013;135:6100–6106.10.1021/ja312015j
- Yamada I. High-pressure synthesis, electronic states, and structure-property relationships of Perovskite oxides, ACu3Fe4O12 (A: divalent alkaline earth or trivalent rare-earth ion). J Ceram Soc Jpn. 2014;122:846–851.10.2109/jcersj2.122.846
- Yamada I, Tsuchida K, Ohgushi K, et al. Giant negative thermal expansion in the iron perovskite SrCu3Fe4O12. Angew Chem Int Ed. 2011;50:6579–6582.10.1002/anie.v50.29
- Yamada I, Shiro K, Oka K, et al. Direct observation of negative thermal expansion in SrCu3Fe4O12. J Ceram Soc Jpn. 2013;121:912–914.10.2109/jcersj2.121.912
- Yamada I, Shiro K, Etani H, et al. Valence transitions in negative thermal expansion material SrCu3Fe4O12. Inorg Chem. 2014;53:10563–10569.10.1021/ic501665c
- Yamada I, Marukawa S, Murakami M, et al. “True” negative thermal expansion in Mn-doped LaCu3Fe4O12 perovskite oxides. Appl Phys Lett. 2014;105:231906.10.1063/1.4903890
- Yamada I, Marukawa S, Hayashi N, et al. Room-temperature zero thermal expansion in a cubic Perovskite oxide SrCu3Fe4−xMnxO12. Appl Phys Lett. 2015;106:151901.10.1063/1.4918293
- Yagi S, Yamada I, Tsukasaki H, et al. Covalency-reinforced oxygen evolution reaction catalyst. Nat Commun. 2015;6:8249.10.1038/ncomms9249
- Wang ZL, Xu D, Xu JJ, et al. Oxygen electrocatalysts in metal-air batteries: from aqueous to nonaqueous electrolytes. Chem Soc Rev. 2014;43:7746–7786.10.1039/C3CS60248F
- Hong WT, Risch M, Stoerzinger KA, et al. Toward the rational design of non-precious transition metal oxides for oxygen electrocatalysis. Energy Environ Sci. 2015;8:1404–1427.10.1039/C4EE03869 J
- Fabbri E, Habereder A, Waltar K, et al. Developments and perspectives of oxide-based catalysts for the oxygen evolution reaction. Catal Sci Technol. 2014;4:3800–3821.10.1039/C4CY00669 K
- Suntivich J, May KJ, Gasteiger HA, et al. Perovskite Oxide optimized for oxygen evolution catalysis from molecular orbital principles. Science. 2011;334:1383–1385.10.1126/science.1212858
- Grimaud A, May KJ, Carlton CE, et al. Double perovskites as a family of highly active catalysts for oxygen evolution in alkaline solution. Nat Commun. 2013;4:2439.
- Suntivich J, Hong WT, Lee Y-L, et al. Estimating hybridization of transition metal and oxygen states in Perovskites from OK-edge X-ray absorption spectroscopy. J Phys Chem C. 2014;118:1856–1863.10.1021/jp410644j
- Bocquet AE, Fujimori A, Mizokawa T, et al. Electronic-structure of SrFe4+O3 and related Fe Perovskite oxides. Phys Rev B. 1992;45:1561–1570.10.1103/PhysRevB.45.1561
- May KJ, Carlton CE, Stoerzinger KA, et al. Influence of oxygen evolution during water oxidation on the surface of Perovskite Oxide catalysts. J Phys Chem Lett. 2012;3:3264–3270.10.1021/jz301414z
- Zhu Y, Zhou W, Chen ZG, et al. SrNb0.1Co0.7Fe0.2O3-δ perovskite as a next-generation electrocatalyst for oxygen evolution in alkaline solution. Angew Chem Int Ed. 2015;54:3897–3901.10.1002/anie.201408998
- Yamada I, Fujii H, Takamatsu A, et al. Bifunctional oxygen reaction catalysis of quadruple manganese Perovskites. Adv Mater. 2017;29:1603004.10.1002/adma.v29.4
- Robinson DM, Go YB, Mui M, et al. Photochemical water oxidation by crystalline polymorphs of manganese oxides: structural requirements for catalysis. J Am Chem Soc. 2013;135:3494–3501.10.1021/ja310286 h