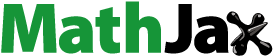
Abstract
Composite molecular sieves, FAU/SBA-15, having core-shell structure were synthesized. The synthesized composite sieves were characterized by X-ray diffractometry (XRD), scanning electron microscopy (SEM), transmission electron microscopy (TEM), energy dispersive X-ray spectroscopy (EDS), pyrolysis fourier transform infrared (Py-FTIR) spectroscopy, temperature programmed desorption spectra (NH3-TPD), UV Raman spectroscopy, nuclear magnetic resonance (NMR) and other techniques. XRD, SEM, TEM, N2 adsorption-desorption, mass spectrometry, NMR and EDS results showed that the composite molecular sieve contained two pore channels. Py-FTIR results showed that the addition of HY molecular sieves improved the acidity of the composite zeolite. The crystallization mechanism during the growth of FAU/SBA-15 shell was deduced from the influence of crystallization time on the synthesis of FAU/SBA-15 core-shell structured composite molecular sieve. HY dissociated partially in H2SO4 solution, and consisted of secondary structural units. This framework structure was more stable than its presence in the isolated form on the same ring or in the absence of Al. Thus it played a guiding role and connected with SBA-15 closely through the Si-O bond. This resulted in the gradual covering of the exterior surface of FAU phase by SBA-15 molecular sieves. The presence of SBA-15 restricted the formation of the other high mass components and increased the selectivity towards ethylbenzene.
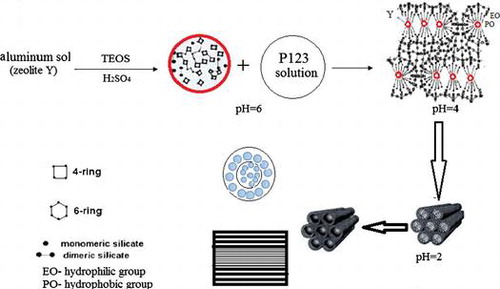
Classification:
1. Introduction
Y-type molecular sieves (FAU type) have unique pore structures and good thermal stabilities [Citation1]. However, the strong acidic nature of Y molecular sieves lowers their performances in shape-selective catalytic reactions. The acidity on the surface and in the pores should be manipulated to enhance their catalytic effects. Ordinary methods of modification not only mask the acidic sites on the surface of the Y-type molecular sieves but also cause acidic sites inside the pores to lose their activity, thereby reducing the catalytic activity [Citation2].
Recent years have seen the emergence of a new type of composite molecular sieves with core–shell structure [Citation3–10]. The advantage is that a pure-silica molecular sieve (e.g. Silicalite-1, SBA-15) is grown on the external surface of an active core (e.g. ZSM-5) [Citation11]. This regulates the acidity on external surface of the molecular sieve and provides entry to large molecules and brings about their initial degradation [Citation12]. The high acidity on the internal surface of the nuclear phase of molecular sieves favors further degradation of the primary products and the diffusion of the final products (relatively small molecules) into the smaller pores of the nuclear phase. This prevents blocking of the pores and thus solves problems [Citation13], such as easy deactivation of catalysts and low yields of high value-added liquid products (too many gaseous products), etc., compared to traditional microporous catalysts used in the catalytic cracking of macromolecules.
Some methods can be used to synthesize core–shell composite molecular sieves [Citation14,15]. Compared to the isomorphous method, the epitaxial method involves a phase comprising of grains on the surface [Citation16], and results in the formation of composite molecular sieve catalyst with core–shell structure. EMT/FAU composite core–shell molecular sieves were successfully synthesized by Goosens et al. [Citation17]. In this synthesis, the zeolite films having FAU structure were oriented on compositionally different micro-sized EMT zeolite crystals. In this synthesis, the zeolite films having FAU structure were oriented on compositionally different micro-sized EMT zeolite crystals.
Composite molecular sieves with core–shell microporous and mesoporous structure [Citation18–20] showed good conversion and selectivity for the catalytic degradation reactions of some macromolecules Zhou et al. [Citation21,22] synthesized c-TUD-1c-Ti-TUD-1 using dry gel conversion method, in which the porous structure of the composites showed better catalytic performance in organic reactions involving macromolecules containing benzene rings compared to traditional zeolites, in addition to good reusability of the catalysts. The cracking of the benzene ring-containing macromolecules, such as 1,3,5-tripropyl benzene does not require the catalyst to be highly acidic, but instead must possess good porosity. The composite porous core–shell material ZSM-5 obtained by base treatment [Citation23], showed relatively high conversion and good stability in the catalytic cracking of 1,3,5-tripropyl benzene. Zhao’s research group prepared ZSM-5@β core–shell molecular sieves [Citation24], in which the porosities and the multilevel acid centers of the composites were beneficial for improving the yield of propylene in the catalytic cracking of macromolecular byproducts (C4-C10 hydrocarbons) [Citation25].
Detailed study on the synthesis of core–shell composite molecular sieves using the microporous molecular sieves Y, with high acidity and high catalytic activity, equivalent to that of ZSM, has been rarely reported. Therefore, in this work, a secondary method has been employed to synthesize FAU/SBA-15 type core–shell composite molecular sieves, to extend the types of core–shell composite molecular sieves and their applications in the macromolecular catalysis. The crystallization mechanism of the shell growth in Y/SBA-15 was deduced, based on the characterization results. Moreover, its effect on the catalytic cracking of polystyrene has also been investigated.
2. Material and methods
2.1. Reagents and raw materials
Sulfuric acid (H2SO4), sodium silicate (water glass), and tetraethyl orthosilicate (TEOS) (chemically pure) were procured from Shenyang Chemical Reagent Factory. (PEO20-PPO70-PEO20) block copolymer (P123) was obtained from Sigma-Aldrich Shanghai. N-hexane (analytically pure) was purchased from Shenyang Chemical Reagent Factory, whereas polystyrene (PS) particles were purchased from Fushun Catalyst Factory.
2.2. Experimental methods
2.2.1. Synthesis of Y molecular sieves
Mix some amount of sodium aluminate, sodium hydroxide, and deionized water in a 100 ml beaker, at 5–20 °C until complete dissolution. Slowly add the sodium silicate (water glass) solution into the fully dissolved sodium aluminate solution, and make the molar composition of the whole: 16 Na2O:Al2O3:15 SiO2:320 H2O. After forming the seed solution, it was stirred for 30 min and aged at room temperature for 2–4 days.
To make alumino silica gel, mix a certain amount of sodium aluminate, sodium hydroxide, and deionized water in a 500 ml beaker, and stir until complete dissolution. Add aged seed solution at room temperature, and make the final molar composition of the reactants: 4.3 Na2O:Al2O3:10 SiO2:180 H2O. After some further stirring, crystallize the mixture in stainless steel crystallization kettle at 90 °C. Filter and wash at 110 °C, and the NaY-type molecular sieves were obtained after drying. The ratio of Si to Al of NaY was 2.44, which was determined by X-ray fluorescence spectroscopy (XRF) [Citation26].
The NH4Cl solution (0.5 mol/L) was exchanged with 10 g NaY zeolite for 24 h. The exchanged NaY zeolite was dried in an oven at 110 °C for 12 h, and then calcined in a muffle furnace at 450 °C for 6 h to obtain HY. The reason for using HY instead of NaY is that the activity of HY is higher than that of NaY, which is favorable for the degradation of polystyrene.
2.2.2. Synthesis of core–shell FAU/SBA-15 composite molecular sieves
The tri-block copolymer P123, H2O, and TEOS were mixed together in a three-necked flask and 10 mol/L sulfuric acid (H2SO4) was dropped slowly to adjust the pH values to 7, 6, 4, 2, or 1. Meanwhile small amounts of Y crystals were added multiple times. The mass ratios of reactants were: m(P123): m(H2O): m(TEOS): m(HY) = 4:30:9.5:4. The mixture was aged at 38 °C for 12 h and crystallized in an oven at 100 °C for 24 h. The hydrothermally synthesized sample was obtained after centrifugation, washing, drying, and calcination at 550 °C. The prepared core–shell material was denoted as SYSp, where p represented the pH of the solution, and p = 7, 6, 4, 2, or 1.
2.3. Characterization methods
X-ray diffraction (XRD) analysis of the powdered samples were conducted using an X-ray diffractometer (XRD-6000, Shimadzu, Japan) using CuKα1 source, with tube voltage of 40 kV and tube current of 80 mA. The step length in small-angle XRD (SXRD) tests was 0.01°, with a scanning rate of 2°/min in the range of 0.4–0.8°. The step length in wide-angle XRD (WXRD) tests was 0.1°, with scanning rate of 8°/min in the range of 10–70°. Surface morphology of the samples was studied by scanning electron microscopy (SEM) at a magnification of 250,000–1,000,000 microscope (SEM) with a magnification of 250,000–1,000,000 X at an accelerating voltage of 0.1–30 kV. The N2 adsorption–desorption isotherms were measured at –196 °C with an Auto Chemistry 2010 instrument. Transmission electron microscopy (TEM) images were obtained using a JEM-2100 TEM from JEOL (Japan) that operated at an accelerating voltage of 200 kV.
The quality scanning range of a LTQ XL linear ion mass spectrometer (Thermo, USA) in negative ion detection mode, equipped with ESI electrospray ion source, is m/z 100–900 Da, spray voltage 4 kV, and capillary voltage –20 V.
Measured by a Bruker MSL-300 nuclear magnetic resonance spectrometer (Germany), the resonant frequency of 27Al magic-angle spinning nuclear magnetic resonance (MAS NMR) is 78.205 MHz, with the rotor rotating frequency 3 kHz, the RF field frequency 51 kHz, the reverse angle 18°, and the cycle time 500s; the resonant frequency of 29Si MAS NMR is 59.592 MHz, the rotor rotation frequency 4 kHz, the RF field frequency 37 kHz, the reverse angle 60°, and the cycle time 2s.
Pyrolysis Fourier transform infrared (Py-FTIR) spectra were recorded on a Bio-Rad FTS15/C spectrometer (Shimadzu, Japan) to determine the surface acid site types (B acid, L acid). UV Raman spectroscopy was carried out on a RM2000 Raman spectrometer (Renishaw, UK). Excitation was provided by a helium–cadmium laser at 325 nm and the laser power at the sample position was about 4.5 mW.
2.4. Catalytic cracking of polystyrene
A certain amount of polystyrene (PS) particulate sample was placed in the homemade hydrocracking micro-reactor device and a certain amount of catalyst (m(SYS2):m(PS) = 1:2) was added to it and mixed homogeneously. Then the device was made airtight. The device was slowly purged with N2 for 5 min and heated up to the decomposition temperature at a heating rate of 20 °C/min for the catalytic degradation to occur. The final temperature for catalyst degradation was 650 °C. The liquid product obtained during degradation was analyzed by liquid chromatography, after condensation.
3. Results and discussions
3.1. Influence of pH on the formation of core–shell composite molecular sieves
3.1.1. XRD and SEM analysis
SYS6, SYS4, SYS2, and SYS1 samples were filtered, dried (not calcined), and then characterized by XRD and SEM to analyze the changes in morphologies and structures of the products in solutions.
Figures and show the XRD patterns and SEM images, respectively, of the continuous tubular core–shell composite molecular sieves during synthesis under different pH conditions. It can be seen from Figures and that with decrease in pH, the crystalline phase underwent gradual compositional changes, until the core–shell structure of the molecular sieve was formed. In case of SYS6 (Figure (a)), octagon-like and spherical-like agglomerations, with diameters more than 200 nm, were seen in the SEM images. No characteristic peaks of (1 0 0), (1 1 0), or (2 0 0) crystal planes appeared, suggesting that this sample did not possess a typical mesoporous structure. Very weak peaks appeared in the wide-angle region, which were characteristic of a microporous structure. These were probably due to the primary tetra-ring structural units formed due to the corrosion of Y-type microporous molecular sieves by H2SO4 and the disappearance of some of the characteristic surface microporous structures. In case of SYS4 sample (Figure (b)), multiple octagon-like and sphere-like agglomerations were present, which showed more rod-like structures compared to the SYS6 sample. By combining this result with the SYS4 pattern in Figure , it was evident that weak characteristic peaks of (1 1 0) and (2 0 0) crystal planes appeared in the small-angle region, which indicated that the characteristic mesoporous structure became more obvious. The SXRD of SYS4 showed some characteristic peaks of microporous structure, but their intensities were low. Hence, it could be deduced that Y was coated with some amount of SBA-15 and was protected from corrosion from H2SO4. Hence, some peaks characteristic of microporous structure could be seen. In case of SYS2 sample (Figure (c)), the magnified image at the lower left corner showed continuous tubular morphology. Combining the morphology of SYS1 sample in Figure (d) with the SYS1 pattern in Figure (b), it was evident that due to highly acidic environment, the Y-type zeolite was corroded completely and showed only the mesoporous form of the composite.
Figure 1. XRD patterns of composite molecular sieves synthesized under different pH conditions: (a) SXRD and (b) WXRD patterns.
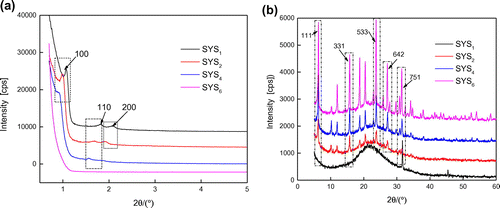
As the pH was lowered, the acid not only provided the required H+ ions for the formation of mesopores, but also partially removed Al from the Y-type zeolite framework. When the Y-type microporous molecular sieve was mixed with P123 solution and the pH value was adjusted from 6 to 2, the original microporous structure was coated with mesoporous molecular sieve. The adjustment of pH had influence on two aspects of the synthesis of the core–shell structure of the molecular sieve products. The first aspect was promotion of agglomeration of amorphous silicon and aluminum species in the system and the subsequent formation of mesoporous phase structure. The second aspect was breaking of the chemical equilibrium between the amorphous silicon and aluminum species. The Y-type microporous zeolite crystals in the system resulted in the partial destruction of the zeolite structure. This destruction did not degrade the structure completely to the amorphous Si–O or Al–O tetrahedral structure, but instead degraded it to the microporous structural units.
3.1.2. Changes in silicon species
With lowering of pH, the silicon species in the mesoporous system mainly underwent the following two reaction steps.
The first step was carried out under weakly acidic conditions:
The second step was carried out by gradually increasing the acidity (Figure ):
It could be seen that the introduction of acid into the weakly acidic medium, provided H+ continuously (pH = 6). This shifted the chemical equilibrium of the reaction in the first step to the right side. This reversible reaction medium showed some buffer-like properties in maintaining the pH value and had protective effect on the Y-type microporous zeolite structure. As the acidity increased gradually (<pH 6 < 2), a rapid polymerization of the silicates occurred in the second step, which promoted the formation of the mesoporous phase (pH = 2). Increase in the amount of acid (pH = 1) introduced led to an increase in amount of aluminum removed from the framework and consequently the degradation of FAU type crystals.
Hence, the structure of the synthesized FAU/SBA-15 composite molecular sieves was dependent on pH. Decrease in pH during the synthesis caused the destruction of the crystalline structure of FAU, and meanwhile this structure was coated with the newly formed SBA-15. Thus, the core–shell structure of the composite molecular sieve with FAU as core and SBA-15 as shell was obtained with both, microporous and mesoporous structures.
3.2. Characterization of the core–shell structure of composite molecular sieves
3.2.1. TEM
It could be further seen from TEM images (Figure ) that the shell phase SBA-15 coated on the external surface of the core phase FAU was highly dense with no cavities or vacancy defects (Figure (b)). The boundary between micropores and mesopores could be clearly seen (Figure (a)) and the whole shape was continuously tubular (Figure (c)). The middle region was darker. The structure with smaller pore size was microporous (core phase) while the exterior structure with a larger pore size was the mesopore (the thickness of shell phase was 100–200 nm).
Figure 4. TEM images of core–shell structure SYS2: the boundary between micropores and mesopores (a), cross section (b), overall morphology (c).
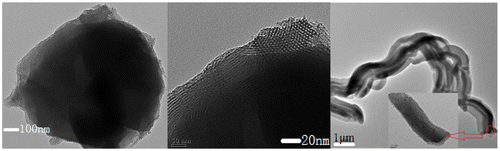
Figure further demonstrated the coexistence of microporous and mesoporous structures in the core–shell composite molecular sieves (sample SYS2).
Figure 5. The overall morphology of core–shell composite sieves (a), enlarged image of a section of the core–shell composite sieve (b), diffraction patterns of Y molecular sieves (c), and diffraction patterns of core–shell composite molecular sieves (d).
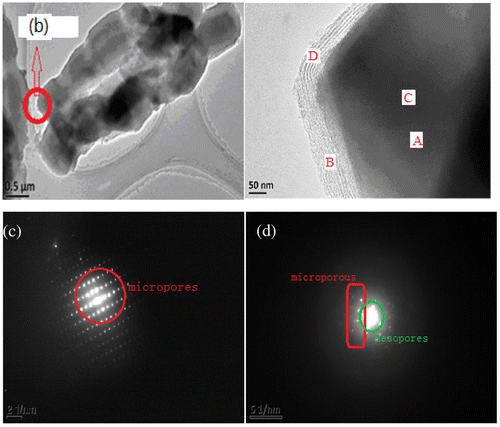
Figure (a) shows the overall surface topography of the sample (SYS2). Figure (b) shows the magnified image of a section of Figure (a), in which the boundary between the core microporous and shell mesoporous phases was clear. Figure (c) shows the diffraction pattern of Y-type microporous molecular sieves, which has uniform spots. Figure (d) shows the coexistence of large spots and small spots in the diffraction patterns of the composite molecular sieve, indicating that the core–shell composite molecular sieve contained two pore channels of micropores and mesopores, instead of the microporous molecular sieves with single pore size.
3.2.2. Elemental analysis
In order to further prove the core–shell structure of SYS2, energy-dispersive spectroscopy (EDS) measurements were performed at four points, sequentially from the interior to the exterior, as shown in Figure (b) and the analytic results are presented in Table .
Table 1. Elemental analysis of SYS2 by TEM/EDS.
Elements such as Si, Al, O, and Na were found to be present in the composite molecular sieve. This was consistent with the different elements present in the raw materials, used for synthesizing the molecular sieves. Moreover, their intensities were also in accordance with the amounts of the raw materials added. More specifically, the atomic ratios of Si:Al at A and C points were 5 and 4.6 (close to the atomic ratios of Si:Al in Y molecular sieves), and the atomic ratios of Si:Al at B and D points were 81.25 and 91 (close to the atomic ratios of Si:Al in SBA-15 molecular sieves). This was a further indication that the composite molecular sieve had a core–shell structure.
3.2.3. Porosity analysis
Analysis of N2 adsorption desorption Y, SBA-15, SYS2 adsorption desorption isotherms is shown in Figure .
It can be seen from Figure , the Y adsorption desorption isotherms for I, microporous molecular sieve is a typical line; adsorption–desorption isotherms for SBA-15 type V is mesoporous molecular sieve type typical line, and SYS2 IV line is typical at low relative pressure stage. The N2 adsorption–desorption isotherms of SYS2 composite molecular sieves showed obvious microporous and mesoporous characteristics, which demonstrated that it was composite molecular sieves with composite pore structures. The detailed properties are given in Table .
Table 2. Surface area and average desorption pore volume of SYS2 sample. BET stands for Brunauer–Emmett–Teller and BJH for Barrett–Joyner–Halenda.
The distribution of pore sizes is shown in Figure . The sizes of micropores and mesopores are approximately 1.85 and 6 nm, respectively.
3.3. Analysis of acid sites
Pyridine is absorbed on the acid sites of the composites (HY/SBA-15 and NaY/SBA-15). The peaks at 1542 and 1450 cm−1, due to chemisorbed pyridine, are used to determine the number of acid sites on the composite. The ratio of the peak (1442 cm−1) was weak that indicates that both Na+ and non-framework aluminum species in NaY/SBA-15 zeolite are weak L acid sites. The peaks at 1450 and 1542 cm–1 can be attributed to the C–C stretching vibrations of the pyridine complex with the Lewis acid and that of the pyridine complex with the Brønsted acid, respectively. The Brønsted acid of HY/SBA-15 is stronger than NaY/SBA-15.The infrared spectrum of pyridine showed that the addition of HY improved the acidity of the composite zeolite. The results of Py-FTIR showed that the addition of HY improved the acidity of the composite zeolite (see Figure ).
The results of acid content and distribution of HY, NaY/SBA-15, and HY/SBA-15 molecular sieves obtained from the temperature-programmed desorption (NH3-TPD) spectra [Citation26] are shown in Table . Compared to NaY/SBA-15, the proportion of medium and strong acids of HY/SBA-15 increased, while the proportion of weak acids decreased.
Table 3. The acidity and acidic distribution of three kinds of molecular sieves.
4. Mechanism of crystallization
4.1. UV Raman spectroscopic analysis
UV Raman spectroscopy can prevent disturbances due to fluorescence, generated by the intermediate species during the synthesis of molecular sieves and enhance the sensitivity of the measurement. Meanwhile, this characterization method showed much higher recognition sensitivity toward templates than XRD, which provided the possibility of studying the in situ mechanism of synthesis of molecular sieves using in situ UV Raman spectroscopy [Citation27–29].
Figure shows the UV Raman spectrograms of the liquid phase and solid phase during the crystallization process of the composite molecular sieves.
Characteristic bands at 380, 520, and 610 cm−1, attributed to P123, appeared in all the spectra except for spectrum 2. During the crystallization process of the core–shell of the composite molecular sieve, the template agent P123, which was initially in both the solid and liquid phases, was transformed completely to the solid phase. This indicated that when the pH of the system was reduced, the silicon species (positive charges) agglomerated, due to electrostatic interactions, around the P123 micelles (negative charges) in the liquid phase. This transformed the precipitates formed, by coating P123 in the liquid phase onto the exterior surface of Y to form the solid gel phase. The formed mesoporous phase showed a highly ordered two-dimensional hexagonal structure (the morphologies of the samples after crystallization in hot water can be seen in Figure ).
Compared to spectrum 4, spectrum 3 showed a new characteristic peak at 298 cm−1 and the initial peak at 520 cm−1 shifted to 514 cm−1, both indicating the long-range ordered environment of the structural unit of HY [Citation30]. In spectrum 1, the band at 778 cm−1 could be assigned to the oligomeric silicon species in the liquid phase [Citation31]. HY dissociated partially in H2SO4 solution and formed the secondary structural unit. The characteristic singlets at 1024 and 1310 cm−1 were attributed to the silicon species in the liquid phase. When the pH was reduced to 2, the gelation increased markedly and the system became very viscous. In spectrum 2, only one band at 985 cm−1, attributed to , could be observed. The appearance of this band was due to the addition of H2SO4 to adjust the pH of the system [Citation32]. In spectrum 5, the characteristic bands at 298 and 380 cm−1 were assigned to the breathing vibrations of dual-hexatomic rings, whereas the typical vibrational bands of SBA-15 appeared at around 500, 606, 808, and 976 cm−1. Among these, the broad band at around 500 cm−1 overlay the vibrational bands of the four-, five-, and six-membered rings in the mesoporous molecular sieves. The band at 606 cm−1 was probably due to the Si–O bridged bond in the mesoporous molecular sieve. The band at 808 cm−1 could be attributed to the Si–O–Si symmetric stretching vibration in the molecular sieve, and the band at 976 cm−1 was a result of the surface Si–OH groups [Citation33,34].
4.2. Possible mechanism of the formation of FAU/SBA-15 composite core–shell structured molecular sieve
From the above results, the mechanism for the formation of the FAU/SBA-15 core–shell structured molecular sieve was proposed (Figure ).
After the addition of octagonal HY crystal particles into the SBA-15 synthetic crystallization environment, the gelation around the Y crystals (FAU phase) changed with variation of pH. HY dissociated partially in the H2SO4 solution (pH = 6) and consisted of secondary structural units.
The result of MAS NMR and Mass spectrometry are supportive in the analyses secondary structural units (see Figure ).
The peaks in the 29Si spectra of HY molecular sieves when δ = –88.48, –94.12, –98.14, and –103.81 are in turn attributed to Si (3Al), Si (2Al), Si (1Al), and Si (0Al) in their skeletons. The facts that the signals move toward high field and are obviously broadened after acid treatment indicate that the zeolite skeleton has been destroyed and become amorphous, producing a large amount of Si–OH. Meanwhile, the proportion of Si species with low Al content is increased, which indicates that Al is removed from the sample after acid treatment.
From the 27Al MAS NMR spectra, the acid treated HY molecular sieve appears a new peak at δ = 0, which shows that acid treatment turns the original HY molecular sieve with six-coordinated Al on the skeleton part to four-coordinated Al. It can be seen that the peak of the four-coordinated Al at δ = 55 is obviously broadened, which indicates that the environment uniformity of the four-coordinated Al has been improved, and the secondary structure unit with tetra-atomic ring structure has been obtained [Citation35].
Using electrospray ionization mass spectrometry, we studied the secondary structural units in which HY is dissolved in the liquid phase of H2SO4, as is shown in Figure .
As can be seen from Figure , the mass spectra values m/z = 30.7 and 75 correspond to C6H3 and , respectively. The major fragmentation peak of the secondary structure is
(m/z = 154), and we have speculated about the structure of the tetra-atomic ring.
It was proved by the self-consistent field molecular orbital calculation that, considering the energy aspect, Al preferably existed on the tetra-rings. This framework structure was more stable than its presence in the isolated form on the same ring or in the absence of Al [Citation36]. Since the bond length of Si–O (0.165 nm) was smaller than that of Al–O (0.175 nm), substitution of Si at the Al position led to irregular contraction of crystal cell and decrease in stability (pH = 4). Thus, it played a guiding role and connected with SBA-15 closely through the Si–O bond (pH = 2). Finally, this resulted in the gradual covering of the exterior surface of FAU phase by SBA-15 molecular sieves [Citation37,38].
5. Catalytic degradation of polystyrene by composite molecular sieve
The synthesized SYS2 core–shell structured molecular sieves were applied to the catalytic cracking reaction of PS and the analysis of the liquid products is presented in Table .
Table 4. Reaction products of catalytic degradation and thermal cracking of PS.
The thermal cracking of PS alone produced styrene monomer as the major product. After the introduction of catalyst, the selectivity for benzene, toluene, ethylbenzene, isopropylbenzene, etc. (all belonged to harmonic composition of gasoline with high octane number) increased, which was due to the attack of the proton at Brønsted acid site of the acidic catalyst surface, on the phenyl group of polystyrene. This led to the intermolecular or intramolecular hydrogen transfer, decrease in β-type chain scissions, increase in other modes of chain scission, transformation of more of PS to ethylbenzene, and so on. The selectivity toward ethylbenzene increased remarkably when catalyzed with the core–shell structured SYS2 molecular sieve, which increased from 6.46% by catalyzed HY with the non-core–shell structured (3.10% by thermal cracking) to 10.17%. Since the interior of the SYS2 core–shell structured molecular sieve still retained the Y strong acidic center, and the shell was completely covered with SBA-15, which was weakly acidic, side reaction such as isomerization on the exterior surface was prevented and the conversion of olefin and selectivity to arene showed improvement. Meanwhile, PS degraded initially when passing through the mesopores and the degradation products entering the micropores were smaller. The relatively strong acidic centers in the micropores facilitated the further degradation of the initial products. As a result, the yields of products with smaller molecular weights (e.g. benzene, toluene, ethylbenzene), when catalyzed by SYS2, were higher than that catalyzed by HY. Moreover, the yields of higher mass components were also lower than HY. The shell part of the SYS2 sample (SBA-15) covered almost the entire exterior surface of FAU forming core–shell structured system with connected pore channels, and enhanced the synergistic catalysis effect. In addition, the existence of the SBA-15 shell restricted the formation of other high mass components and increased the selectivity toward ethylbenzene.
6. Conclusions
FAU/SBA-15 core–shell structured composite molecular sieves with two kinds of porous structures, unique selectivity, and active sites were synthesized. The further growth of the molecular sieve shell by crystallization was precisely controlled. The key to the synthesis was to use rational acidity to control the growth of the shell mesoporous phase crystals. The composite molecular sieve possessed two kinds of porous structures and exhibited unique selectivity and activity. During the catalytic cracking of polystyrene, the macromolecular products could pass through the pore channels connecting SBA-15 shell and HY core and were transferred to the active catalytic centers for the reaction. Then, the secondary cracking reaction took place and the molecules were transformed into arenes. Moreover, synergistic effects were shown by the two kinds of molecular sieves. Therefore, the core–shell synthesized composite porous structures showed improved the catalytic cracking of polystyrene, to obtain the harmonic components of gasoline with high octane number, such as ethylbenzene.
Disclosure statement
No potential conflict of interest was reported by the authors.
Funding
A part of this work was supported by the Research Fund for the Foundation of Liaoning Education Committee [L2017LQN002]; the Doctoral Program of Higher Education by Chinese Education Department [grant number 20100042110008]; Liaoning BaiQianWan Talents Program, and the Talent Scientific Research Fund of LSHU [grant number 2016XJJ-064].
References
- Caeiro G, Carvalho RH, Wang X. Activation of C2–C4 alkanes over acid bifunctional zeolites over and bifunctional zeolite catalysts. J Mol Catal A Chem. 2006;255:131–158.10.1016/j.molcata.2006.03.068
- Chen J, Wang Y, Wei HL. Catalytic performance of methanol alkylation of toluene with silicalite-1 HZSM-5/composite molecular sieve. Nat Gas Chem Ind. 2009;34:22–26.
- Fa-Kuen S, Wang SC, Chia-I Y, et al. Imparting functionality to biocatalysts via embedding enzymes into nanoporous materials by a de novo approach: size-selective sheltering of catalase in metal-organic framework microcrystals. J Am Chem Soc. 2015;137:4276–4279.
- Malgras V, Ji QM, Kamachi YC, et al. Templated synthesis for nanoarchitectured porous materials. Bull Chem Soc Jpn. 2015;88:1171–1200.10.1246/bcsj.20150143
- Ariga K, Yamauchi Y, Rydzek G, et al. Layer-by-layer nanoarchitectonics: invention, innovation, and evolution. Chem Lett. 2014;43:36–68.10.1246/cl.130987
- Malgras V, Ataee-Esfahani H, Wang HJ, et al. Nanoarchitectures for mesoporous metals. Adv Mater. 2016;28:993–1010.10.1002/adma.201502593
- Wang Y, Gu HC. Core–shell-type magnetic mesoporous silica nanocomposites for bioimaging and therapeutic agent delivery. Adv Mater. 2015;27:576–585.10.1002/adma.v27.3
- Wang L, Liu CH, Nemoto Y, et al. Rapid synthesis of biocompatible gold nanoflowers with tailored surface textures with the assistance of amino acid molecules. RSC Adv. 2012;2:4608–4611.10.1039/c2ra20348 k
- Wei Y, Li XM, Elzatahry AA, et al. A versatile in situ etching-growth strategy for synthesis of yolk-shell structured periodic mesoporous organosilica nanocomposites. RSC Adv. 2016;6:51470–51479.10.1039/C6RA08541E
- Yue Q, Zhang Y, Jiang YJ, et al. Nanoengineering of core–shell magnetic mesoporous microspheres with tunable surface roughness. J Am Chem Soc. 2017;139:4954–4961.10.1021/jacs.7b01464
- Kong DJ, Zou W, Zheng JL, et al. Influence factors and crystallization kinetics of the synthesis of MFI/MFI core shell molecular sieves. Acta Phys Chim Sin. 2009;25:1921–1928.
- Dutta S, Bhaumik A, Wu KCW. Hierarchically porous carbon derived from polymers and biomass: effect of interconnected pores on energy applications. Energy Environ Sci. 2014;7:3574–3592.10.1039/C4EE01075B
- Zhao DY. China: a big player in a small world. ACS Cent Sci. 2016;2:577–578.10.1021/acscentsci.6b00275
- Zhu HW, Jing YK, Pal M, et al. Mesoporous TiO2@N-doped carbon composite nanospheres synthesized by the direct carbonization of surfactants after sol–gel process for superior lithium storage. Nanoscale. 2017;9:1539–1546.10.1039/C6NR08885F
- Goossens AM, Wouters BH, Grobet PJ, et al. Synthesis and characterization of epitaxial FAU-on-EMT zeolite overgrowth materials. Eur J Inorg Chem. 2001;2001:1167–1181.10.1002/(ISSN)1099-0682
- Kong D, Zheng J, Yuan X, et al. Fabrication of core/shell structure via overgrowth of ZSM-5 layers on mordenite crystals. Microporous Mesoporous Mater. 2009;119:91–96.10.1016/j.micromeso.2008.10.001
- Goossens AM, Wouters BH, Buschmann V, et al. Oriented FAU zeolite films on micrometer-sized EMT crystals. Adv Mater. 1999;11:561–564.10.1002/(ISSN)1521-4095
- Huang ZH, Che S. Fabrication of mesostructured silica materials through co-structure-directing route. Bull Chem Soc Jpn. 2015;88:617–632.10.1246/bcsj.20140416
- Yamamoto E, Kuroda K. Colloidal mesoporous silica nanoparticles. Bull Chem Soc Jpn. 2016;89:501–539.10.1246/bcsj.20150420
- Qian XF, Du JM, Li B, et al. Controllable fabrication of uniform core–shell structured zeolite@SBA-15 composites. Chem Sci. 2011;2:2006–2013.10.1039/c1sc00250c
- Zhou J, Hua ZL, Cui XZ. Hierarchical mesoporous TS-1 zeolite: a highly active and extraordinarily stable catalyst for the selective oxidation of 2,3,6-trimethylphenol. Chem Commun. 2010;46:4994–4997.10.1039/c0cc00499e
- Zhou J, Hua ZL, Zhao JJ. A micro/mesoporous aluminosilicate: key factors affecting framework crystallization during steam-assisted synthesis and its catalytic property. J Mater Chem. 2010;20:6764–6773.10.1039/c0jm00513d
- Lei Q, Zhao T, Li F. Catalytic cracking of large moleculed over hierarchical zeolites. Chem Commun. 2006;103:1769–1772.10.1039/b600547 k
- Liu M, Fan SM, Zhao TS. The eighteenth National Symposium on molecular sieves. 18th ed. Yinchuan: Yinchuan University Press; 2015.
- Tang YN, Zhou HG, Li T. Fe3O4/TiO2 composite application in photocatalytic degradation of RhB. Guangdong Chem Ind. 2016;43:64–68.
- Shi CW, Zhou WF, Sun XH, et al. Morphology control and aromatization of Y/SBA-15 composite zeolite. Appl Chem Ind. 2016;45:1085–1088.
- Ma Y, Wang XL, Li C. Charge separation promoted by phase junctions in photocatalysts. Chin J Catal. 2015;36:1519–1527.10.1016/S1872-2067(15)60874-9
- Dutta PK, Shieh DC, Puri M. Correlation of framework Raman bands of zeolites with structure. Zeolites. 1988;8:306–309.10.1016/S0144-2449(88)80127-1
- Dutta PK, Shieh DC. Crystallization of zeolite-A: a spectroscopic study. J Phys Chem. 1986;90:2331–2334.10.1021/j100402a017
- Fan FT, Li C. From molecular fragments to active sites: in situ, resonance UV Raman spectroscopy study on zeolitic catalyst. Sci Sin Chim. 2013;43:1818–1840.10.1360/N032013-00023
- Guo Q, Fan FT, Guo ML, et al. UV Raman spectroscopic studies on the mechanism of FeAlPO4−5 synthesis. Chin J Catal. 2012;33:106–113.10.1016/S1872-2067(10)60281-1
- Tepavitcharova S, Balarew F, Rull F, et al. Raman spectroscopic studies of ion association in the Na+, Mg2+/Cl−, SO42−/H2O system. J Raman Spectrosc. 2005;36:891–897.10.1002/(ISSN)1097-4555
- Zhang W, Lu J, Han B, et al. Direct synthesis and characterization of titanium-substituted mesoporous molecular sieve SBA-15. Chem Mater. 2002;14:3413–3421.10.1021/cm011686c
- Sung-Suh HM, Luan Z, Kevan L. Photoionization of porphyrins in mesoporous siliceous MCM-41, AIMCM-41, and TiMCM-41 molecular sieves. J Phys Chem B. 1997;101:10455–10463.10.1021/jp972772w
- Patricia PR, Héctor AH, Jaime SV, et al. Crystallization of faujasite Y from seeds dispersed on mesoporous materials. Microporous Mesoporous Mater. 2010;132:363–374.
- Sanhueza V, Kelm U, Cid R. Photoionization of porphyrins in mesoporous siliceous. J Chem Tech Biotech. 2003;78:485–488.10.1002/(ISSN)1097-4660
- Zhang L, Wang HY, Jiang ZX. Synthesis and catalytic activity of core–shell MFI/CHA zeolites. Acta Phys Chim Sin. 2016;32:745–754.
- Yin SH, Li SW, Zhang B, et al. Extraction kinetics of neodymium(III) from chloride medium in the presence of two complexing agents by D2EHPA using a constant interfacial area cell with laminar flow. Hydrometallurgy. 2016;161:160–165.10.1016/j.hydromet.2016.01.016