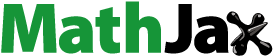
ABSTRACT
Thermo-, pH- and glucose-responsive polymeric nanoparticles are of great interest in developing a self-regulated drug delivery system. The novel core-shell nanoparticles were synthesized by self-assembly of a phenylboronic acid-based block copolymer poly-(N-isopropylacrylamide)-block-poly(3-acrylamidophenylboronic acid) (PNIPAM136-b-PAPBA16) and a fluorescent complex glucosamine-poly(N-isopropylacrylamide)/Eu(III) (GA-PNIPAM)/Eu(III) based on the cross-linking between PBA- and GA-containing blocks in this work. The nanoparticles can be tuned via thermo-induced collapse or glucose-induced swelling at appropriate pH and temperatures; they had an average kinetic radius was about 80nm, and which showed excellent fluorescence. MTT assays revealed the nanocarriers had no significant cytotoxic response of the micelle when it was observed in the cell line over the concentration range from 0.1 to 1000 μg/ml at any exposure times.
GRAPHICAL ABSTRACT
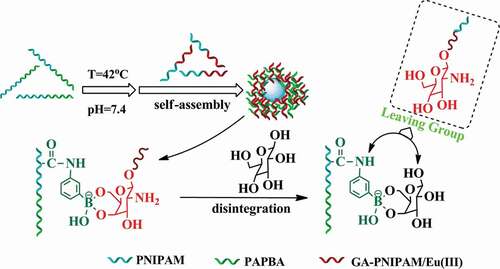
1. Introduction
Glucose-sensitive drug delivery system based on phenylboronic acid (PBA) has been a growing interest due to it can control the release of drugs automatically and continuously according to the change of glucose concentration [Citation1,Citation2]. PBA and its derivatives can be applied to glucose-sensitive drug delivery system for diabetes treatment in the future because of which can specific bind with 1.2-diols or polyols to formate reversible covalent PBA/diol complexes [Citation3]. This specific binding property enables the PBA functionalized carrier to have good glucose sensitivity and makes PBA-based glucose-sensitive drug delivery from materials to medicine in the future [Citation4,Citation5]. The system may be a self-regulated drug control release system, it can reduce the number of insulin injections and improve the patient’s compliance. So PBA-based glucose-sensitive hydrogels, microgels and nano-carriers have made great progress [Citation6–Citation9]. However, there are many difficulties of PBA and its derivatives as the glucose-sensitive drug delivery system is used for diabetes treatment. For example, the pH of glucose response is higher than the physiological pH and glucose response concentration is much higher than the blood glucose level of diabetic patients [Citation10]. Therefore, boronic acid-modified polymers can be coupled with other environment-sensitive monomers or polymers to synthesize multiple-responsive nano-carriers to be used in drug delivery, because these nano-carriers can undergo dissolution or self-assembly to achieve the drug release rate in response to the variational local environment through their functional groups. [Citation11–Citation13]. According to different response factors, PBA-based glucose-sensitive nano-carriers can be divided into pH response, temperature response, light response and other types.
Among these nano-carriers, the temperature response nano-carriers with special structure by using the temperature sensitivity of poly(N-isopropylacrylamide)(PNIPAM) can control the release of insulin by self-adjusting ‘on/off ’ mode and protect drugs from the degradation of enzymes, which have great potential application value in the treatment of diabetes [Citation14–Citation18]. Shi and co-workers prepared complex polymeric micelle (CPM) through the self-assembly of two types of diblock copolymers, poly(ethylene glycol)-b-poly(aspartic acid-co-aspartamidophenylboronic acid) (PEG-b-P(Asp-co-AspPBA)) and poly(N-isopropylacrylamide)-b-poly(aspartic acid-co- aspartamidophenylboronic acid) (PNIPAM-b-P(Asp-co-AspPBA)) [Citation15]. The CPM enabled the repeated on–off release of insulin regulated by glucose level. To enhance the glucose sensitivity and self-regulated release of insulin, the group fabricated glucose-responsive polymer based on the complexation between a glucosamine (GA)-containing block copolymer PEG45-b-P(Asp-co-AspGA) and a phenylboronic acid (PBA)-containing block copolymer PEG114-b-P(Asp-co-AspPBA) with α-CD/PEG45 inclusion complex as the sacrificial template [Citation15]. The cross-linking between PBA- and GA-containing blocks can enhance the stability of the shell. Therefore, the addition of a GA-containing block copolymer PEG-b-P (Asp-co-AspGA) resulted in the formation of core-shell-corona (CSC) complex micelles had a hydrophilic vesicular membrane which was favorable for the penetration of water-soluble substances and exhibited prominent glucose-responsiveness at physiological pH 7.4 [Citation17,Citation18]. With the development of nanotechnology, nanoscale drug carriers are attracting more and more attention [Citation19,Citation20].
In the previous research, most of the polymer preparation of GA used amino group for reaction, and most of the polymers were synthesized by free radical polymerization. However, in this study, we retained the amino of GA, which is better for retaining the activity of GA. We synthesized polymer by atom transfer radical polymerization (ATRP) and reversible addition-fragmentation chain transfer polymerization (RAFT), so the reaction is more controllable. Meanwhile, europium fluorescence system is adopted in this study, which can be directly used for determination in the future. In a previous work of our team, a series of thermo-sensitive GA terminated-PNIPAM polymers (GA-PNIPAM) had been developed in our team by ATRP [Citation21,Citation22], and which could coordinate with Eu(III) ions to form the (GA-PNIPAM)/Eu(III) complexes with amino. To explore the novel PBA-based glucose-sensitive drug delivery, in this work, the thermo-sensitive phenylboronic acid PBA-containing block copolymer poly(N-isopropylacrylamide)-block-poly(3-acrylamidophenylboronic acid) (PNIPAM136-b-PAPBA16) were fabricated by RAFT. Then, the addition of a GA-containing complexes (GA-PNIPAM)/Eu(III) resulted in the formation of core-shell complex micelles based on the cross-linking between PBA- and GA-containing blocks as shown in Scheme 1. The results of the transmission electron microscopy (TEM) and dynamic light scattering (DLS) revealed the complex micelles were excellent nanoparticles which may be a promising candidate for glucose-responsive drug delivery for diabetes treatment.
2. Materials and methods
2.1. Materials and instrumentation
N-Isopropylacrylamide (Aldrich, 98%) was recrystallized twice from a hexane/benzene mixture (3/2, v/v). Tris(2-(dimethylamino)ethyl)amine (Me6TREN) was synthesized from tris(2-amino-ethylamine (TREN, Aldrich, 99%) according to the literature [Citation23]. CuBr (Aldrich, 99%) was washed successively with acetic acid and ether and then dried and stored under nitrogen. 3-Aminobenzeneboronic acid (Aladdin, 97%), acryloyl chloride (J&K Chemical, AR), 2,2ʹ-azobis(2-methylpropionitrile)(AIBN; J&K Chemical, 98%), 1,3,5-Trioxane (Aladdin, 99%), 1-dodecanethiol (Aladdin), tetrabutylammonium bromide (Aladdin), carbon disulfide (Aladdin), 2-bromo-2-methylpropionic acid (Acros, 98%), 2-bromopropionyl bromide(Aldrich, 97%), D(+)-glucosamine hydrochloride (Aladdin, 99%) and other chemical reagents were obtained commercially and were used as received unless otherwise stated. 3-[4,5-dimethylthiazol-2-yl]-2,5- diphenyltetrazolium (MTT) was purchased from Acros. Human hepatocellular carcinoma cells HepG2 in culture and NCTC clone 929 cells (L-929) were purchased from the Type Culture Collection of the Chinese Academy of Sciences, Shanghai.
The 1H nuclear magnetic resonance (NMR) spectra of monomers and polymers in CDCl3 were obtained on a Varian Unity 400 NMR spectrometer. Molecular weights (Mn) and polydispersity (Mw/M n) were measured using a gel permeation chromatograph (GPC), a Waters 510 pump and a Model 410 differential refractometer at 25°C. Tetrahydrofuran (THF) was used as the mobile phase at a flow rate of 1.0 ml·min−1. The lower critical solution temperatures (LCSTs) of the polymer solutions were determined by absorbance at 500 nm, using a Shimadzu-2600 UV-Vis spectrophotometer with a heating rate of 0.1°C·min−1. The LCST was defined as the temperatures corresponding to 10% decrease of transmittance. Polymer concentration was 1 mg/ml. The average particle size and size distribution of the nanoparticles were characterized by DLS with an Malvern470 instrument at a fixed scattering angle of 90°, after applying 0.45 μm Millipore filters. The morphologies of the nanoparticles were studied by TEM using a JEOL JEM-2100 microscope. The cell viability was evaluated by MTT, the optical density (OD) was measured at 490 nm with a microplate reader (Bio-Rad, USA). Cell viability was determined as a percentage of the negative control (untreated cells).
2.2. General procedure for synthesis of nanoparticles
2.2.1. Synthesis of PNIPAM-b-PAPBA
Poly(N-isopropylacrylamide)-block-poly(3-acrylamidophenylboronic acid) was synthesized using NIPAM and 3-acrylamidophenylboronic acid by RAFT as shown in Scheme 2. Synthesis of block copolymers required four steps. Firstly, we synthesized S-1-dodecyl-S´-(α,α´-dimethyl-α´´-acetic acid) trithiocarbonate (DMP) (1) as a chain transfer agent (CTA). DMP was synthesized by a method derived from Lai et al. [Citation24,Citation25]. 1-Dodecanethiol (4.04 g, 20 mmol), tetrabutylammonium bromide (0.26 g,0.8 mmol), and acetone (10 ml) were mixed in a jacketed reactor with nitrogen gas for 30 min at 0°C. Sodium hydroxide solution (50%) (1.68 g, 21mmol) was added over 15 min. After stirred for an additional 15 min, carbon disulfide (1.525 g, 20mmol) in acetone (2.015 g, 10mmol) was added during which time the color turned red. chloroform (30 ml) was added in one portion after 15 min, followed by drop-wise addition of sodium hydroxide solution (50%)(8 g, 100mmol) over 30 min at 0°C. The reaction was stirred overnight at 25°C. Deionized water (30 ml) was added, followed by concentrated HCl (5 mL) to acidify the aqueous solution. The reactor was stirred intensely under a nitrogen atmosphere to help evaporate off acetone. The solid was collected with a Buchner funnel and then stirred in 2-propanol (100 ml). The undissolved solid was filtered to obtain 1 as a yellow solid (3.62 g, yield 49.7%, melting point 62.5°C) (the results are shown in Figures S1 and S2).
Secondly, we synthesized the macromolecular chain transfer agents 2. A mixture of NIPAM (1.27 g, 11.2 mmol), DMP (40 mg, 0.112 mmol), 1,3,5-trioxane (50.44 mg, 0.56 mmol), AIBN (0.20 mg, 0.011 mmol) in anhydrous dimethylformamide (DMF, 5 ml) was sealed on middle side of an H-shaped ampoule glass and stirred, nitrogen was bubbled through both mixtures for 20 min to remove any oxygen. Three freeze-pump-thaw cycles were performed to degas the solutions. The ampoule was placed at 70°C. The samples were removed periodically by syringe to determine molecular weight. The polymerization was quenched by exposing the solution to air. The solution was concentrated under vacuum and the polymer was precipitated into cold ether three times and dried under a vacuum for 4 h to obtain 2 as a power (0.54 g, yield 42%, Mn = 7780g•mol−1) (the results are shown in Figures S3 and S4).
Thirdly, we synthesized 3-acrylamidophenylboronic acid(APBA) monomer 3. The method was derived from Shinkai et al. [Citation25,Citation26]. 3-Aminophenylboronic acid (3.90 g, 21.9 mmol) was dissolved in a mixture of THF:H2O (66 ml, 1:1, v/v) in a 250-ml round-bottom flask. Sodium bicarbonate (4.05 g, 48.2 mmol) was added to the flask, and the mixture was cooled below 5°C. The mixture of acryloyl chloride (4.36 g, 48.3 mmol) in anhydrous THF (7 ml) was added drop slowly over 1 h. The reaction mixture was stirred overnight and allowed to reach room temperature. After THF was removed under vacuum, ethyl acetate (48 ml) was added into the solution then stirred for 2 h. The organic layers were washed with water twice and brine twice. Ethyl acetate was removed under vacuum, the resulting solid residue was added deionized water (42 ml), and heated at 80°C for 1h until a clear yellow solution was obtained. The solution was vacuum-filtered and cooled to below 10°C to obtain white power. The power was recrystallized by deionized water and dried in a vacuum oven at 25°C for 2 days to obtain 3 (1.53 g, yield 36.7%) (the results are shown in Figures S5 and S6).
Finally, we synthesized PNIPAM-b-PAPBA 4. A mixture of APBA (0.383 g, 2.00 mmol), macro CTA (Mn = 7780 g•mol−1, 0.707 g, 0.091 mmol), 1,3,5-trioxane (50.44 mg, 0.56 mmol), AIBN (1.70 mg, 0.01 mmol) in anhydrous DMF:H2O (7.5 ml, 95:5, v/v) was sealed on middle side of an H-shaped ampoule glass and stirred, nitrogen was bubbled through both mixtures for 20 min to remove any oxygen. Three freeze-pump-thaw cycles were performed to degas the solutions. The ampoule was placed at 70°C for 1 h. The polymerization was quenched by exposing the solution to air. The solution was concentrated under vacuum and the polymer was precipitated into cold ether thrice. The product was dissolved in methyl alcohol and dialyzed for 48 h to purify it and was then dried under vacuum (0.76 g, yield 77%, Mn = 11100 g•mol−1)
2.2.2. Synthesis of GA-PNIPAM/Eu(III) complexes
The GA-PNIPAM/Eu(III) complexes had been synthesized previous work and the method derived from literature 19. The synthetic route of polymer GA-PNIPAM was shown in Scheme 3.
2.2.3. Synthesis of [GA-PNIPAM/Eu(III)]–(PNIPAM-b-PAPBA) complex micelles
The block copolymers PNIPAM-b-PAPBA and the (GA-PNIPAM)/Eu(III) complexes were used for the preparation of the polymer nanoparticles in this study. PNIPAM136-b-PAPBA16(0.16g, Mn = 19200 g•mol−1, 0.8 mmol) was firstly dissolved in DMSO (l ml) for preparation of molecularly dispersed polymer solution. Then, it was diluted with PBS 7.4 solution with (GA-PNIPAM)/Eu(III) complexes (8 mmol) concentration of 0.8 mmol•ml for further studies. To prepare the nanoparticles, the solution was kept in a water bath at 42°C overnight to ensure the formation of the core–shell micelles owing to the complexation between PBA and GA, and the micelles with PNIPAM as shell and cross-linking PBA- and GA-containing blocks as core. Then, a given volume of (GA-PNIPAM)/Eu(III) complexes solution was added into the micellar solution under vigorous stirring according to the desired feed ratio. The solution was immersed in a water bath at 42°C for 24 h.
2.3. Biocompatibility study
Cell viability was investigated using NCTC clone 929 cells (L-929) and Human Hepatocellular Carcinoma Cells HepG2 in culture. After incubation for 24 h in 96-wells plates (8 × 104 cells/ml per well) using Dulbecco’s modified Eagles medium (DMEM) in an incubator (37°C, 5% CO2), the culture medium was mixed with 200 μl of DMEM containing a sample of (GA-PNIPAM)/Eu(III) complexes, PNIPAM-b-PAPBA and [(GA-PNIPAM)/Eu(III)]–(PNIPAM-b-PAPBA) with a range of sample concentrations from 0.1 to 1000 μg/ml (according to the literature [Citation21]): the mixture was further incubated for 48 h. Each sample was tested in six replicates per plate. Then, 20 µl of MTT solutions was added to the mixture in each well, which was incubated for additional 4 h. Next, 200 μl of DMSO was added, and the mixtures were shaken at room temperature. Six replicate wells were used for the control and test concentrations for each microplate. In addition, HepG2 cell suspension concentrations of 1 × 105 cells/ml, 6 × 104 cells/ml, and 4 × 104 cells/ml were used the longer duration exposure experiments (24, 48, 72 and 96 h exposures, respectively) at each time-point of samples. The optical density was measured using a microplate reader at 570nm. The cell viability (%) was calculated according to the following EquationEq. (1)(1)
(1) :
where Asample was the absorbance of the cells incubated in DMEM and mixture and Acontrol was the absorbance of the cells incubated in DMEM.
3. Results and discussion
3.1. Analysis of PNIPAM-b-PAPBA
PNIPAM-b-PAPBA was synthesized by an APBA/macro-CTA/AIBN feed ratio of 20/1/0.1, which could obtain products having a narrow molecular weight distribution range of 1.22–1.39 as shown in Figure S7. The GPC traces of the block copolymer demonstrated a clean shift toward lower elution volume. The polymerization data of PNIPAM-b-PAPBA were shown in Table S1. The architecture of polymer was characterized by 1HNMR and IR spectroscopic analysis. shows the 1HNMR spectra of the PNIPAM-b-PAPBA, which showed all of the peaks expected for the PNIPAM and PAPBA blocks. Compared with macro-CTA (Figure S3), characteristic signals of PNIPAM representing methyl protons on isopropyl groups and protons adjacent to nitrogen atoms were shifted to 3.75 and 6.73 ppm, respectively. On the spectrum, the peaks at 7.93, 7.83, 7.56, 7.34, 8.00, 10.10 ppm confirmed the characteristic group of APBA.
shows an Fourier-infrared transmittance spectrum (FT-IR) of PNIPAM-b-PAPBA. Compared with macro-CTA (Figure S4), the peaks at 1367 cm−1 were ascribed to stretching vibration of PBA group, and 1478 cm−1 were due to stretching vibration of benzene in APBA.
PBA-containing polymers have uniquely stimuli-responsive because their water solubility is determined by pH and solution diol concentration [Citation27]. The pKa value of PAPBA is about 9.3, and the value can be reduced by linking with diol [Citation28]. To investigate the micelles based on the cross-linking between PBA- and GA-containing blocks, the thermo-responsive property of PNIPAM-b-PAPBA was studied by turbidimetry in two conditions (pH value of 7.4 and 9.3) respectively. shows the LCSTs of the PNIPAM-b-PAPBA at pH value of 7.4 and 9.3. The LCSTs are shown in . All LCSTs of PNIPAM-b-PAPBA were below 32°C (PNIPAM’s LCST); this was attribute to that hydrophobic PBA group was introduced into blocks polymers. It is expected that the pH value of the media has a great effect on the volume phase transitions of both micelles. From , the LCST of the same polymer in solution (pH value of 9.3) was higher than in solution (pH value of 7.4). The reason is that the pH value of 9.3 is exactly equal to pKa value of APBA; therefore, the APBA groups in the polymer is formated borate anion, which can enhance the hydrophilicity of polymer. The reason can be explained that the LCST increased with the increase of phenylboric acid content of the polymer. The LCST of P5 was closest to body temperature of above polymer, so it was used to prepare complex micelles in this article.
Figure 3. Transmittance of PNIPAM-b-PAPBA with different molecular weights at λ = 500 nm and for pH = 7.4 and 9.3
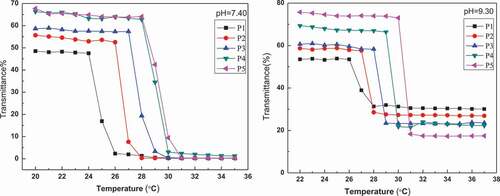
Table 1. LCST behavior of PNIPAM-b-PAPBA with different pH medium
3.2. Analysis of [GA-PNIPAM/Eu(III)]–(PNIPAM-b-PAPBA) complex micelles
The scattered light intensity of the complex micelles at different rates of (GA-PNIPAM)/Eu(III) and (PNIPAM-b-PAPBA) was used to study the dependence of complex micelles on substance concentration and the results are shown in . The complex micelles had the maximum scattering intensity while the molar ratio of the PBA in PNIPAM-b-PAPBA and the GA in (GA-PNIPAM)/Eu(III) was 1:1. This result indicated that cross-linking occurred between the two mixtures and the larger aggregation was formed.
presents the intensity-average hydrodynamic radius distributions f(Rh) of complex micelles. The Rh was about 80nm, and which was calculated by Stokes–Einstein relation. There are few larger complex micelles near the average radius of 180 nm, which may be caused by the formation of GA-bis(boronate) complex which is more favorable in the ‘core’ area [Citation29].
TEM images of the complex micelles provided information on size, shape, and distribution (). Since no macroscopic phase separation occurred in the mixed system, we thought that the composite micelle was formed. Its core was composed of PAPBA/PAGA complex, and the soluble PNIPAM block was a shell. It can be seen from that the diameter of the complex micelles was about 130 ~ 380nm, which was larger than that of DLS test. It may be caused by the extrusion of the sample.
In order to confirm the presence of complex micelles as a result of cross-linking between PBA- and GA-containing blocks, this study adopts a ternary system. The glucose was added to the complex micelles. Glucose will connect with PAPBA to form a new covalent bond because of competitive complexation as shown in Scheme S1. The cross-linking between PBA- and GA of (GA-PNIPAM)/Eu(III) will be broken when the external glucose concentration increases, and a new covalent bond will be formed between PAPBA and glucose in system. After the newly formed micelle was dialyzed, no fluorescence of the new substance was found under the ultraviolet light. However, red fluorescence is observed from the dialysate (freeze-dried) in Figure S7. This phenomenon reveals that the GA of (GA-PNIPAM)/Eu(III) was separated from the cross-linked complex micelles. Uv-vis absorption spectra were used to further confirm that micelles were formed by the reversible cross-linked between PBA- and GA-containing blocks (Figure S8). The characteristic absorption peak of Eu(III) at 355nm is clearly seen of complex micelles in Figure S8. The characteristic absorption at 267nm is the peak of PBA of micelles, which is red shifted to 262 nm in the PNIPAM-b-PAPBA and glucose.
The fluorescence spectra of (GA-PNIPAM)/Eu(III) and complex micelle are shown in . The complex micelle displayed four strong, narrow emission peaks at 579, 591, 613 and 658 nm, corresponding to the 5D0→7FJ, (J = 0, 1, 2, 3) electronic transitions, respectively, which occurred from the excited D state to the multiplet F state. The most pronounced peak was at 613 nm (5D0→7F2). These are strong evidence for the formation of complex micelles which was the cross-linking between PBA- of PNIPAM-b-PAPBA and GA- of (GA-PNIPAM)/Eu(III). The fluorescence intensity of the composite micelle was lower than that of the (GA-PNIPAM)/Eu(III) with the same concentration, because of the lower content of europium.
Figure 7. Fluorescence spectra recorded for (GA-PNIPAM)/Eu(III) and complex micelles (excitation wavelength 355 nm)
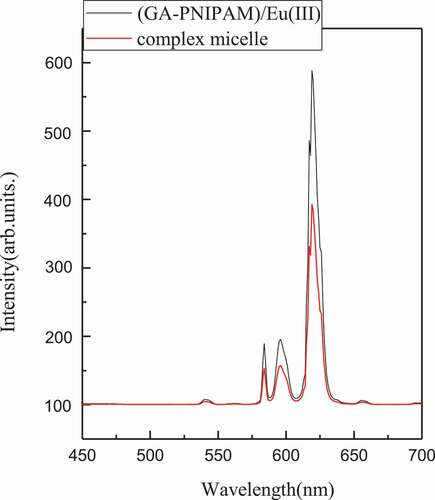
Thermo-responsive properties of the complex micelles will assist the carrier in drug release, so we studied the transmissivity of complex micelles with the change of temperature and the results are shown in . It can be seen that the transmittance depends on temperature, and there is basically no change of the transmittance in the range of 20 ~ 33°C. When the temperature above 33°C, the transmittance of the complex micelles rapidly drops from 86.72% to 77.65%, and continues to rise until the transmittance of the complex micelles at 33.6°C drops to 44.2% and finally reaches a plateau. Therefore, it can be deduced that the LCST of the complex micelles is 33.6°C.
The complex micelles are responsive not only to temperature but also to the pH and glucose concentration ( and ). From , the fluid dynamics diameter of complex micelles is about 80nm at pH = 7.4. When the pH value is between 6 and 7.4, the hydrodynamic diameter of the complex micelles with the decrease of pH. This is due to the cross-linking between PBA- and GA-containing blocks towards the direction of dissociation when the pH value is less than pKa. PNIPAM-b-PAPBA is hydrophobic and will form a micelle with PAPBA as the core and PNIPAM as the shell, the diameter rather than cross-linked between PBA- and GA-containing blocks.
Figure 9. Ph dependence of scattered intensity-average hydrodynamic radius <Rh> recorded for the aqueous mixture of complex micelles
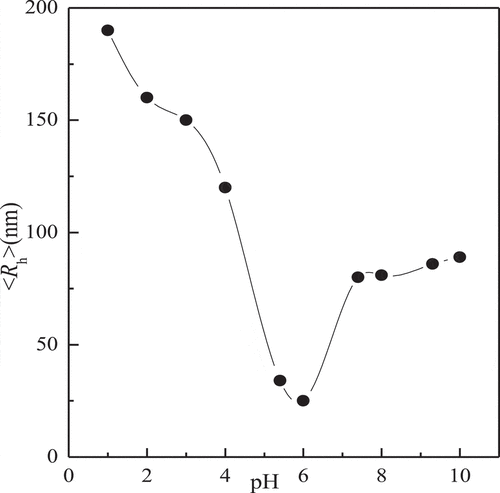
Figure 10. Glucose concentration dependence of scattered light intensity recorded for the aqueous mixture of complex micelles at pH = 7.4
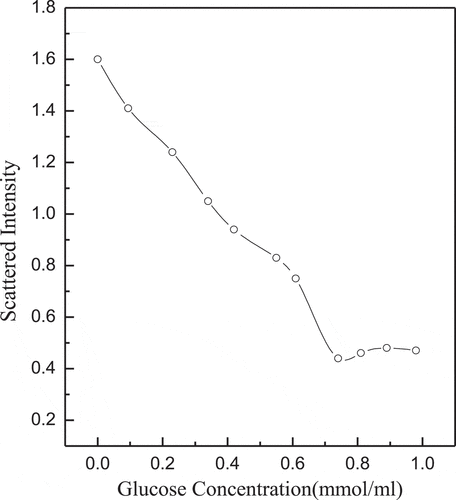
shows the scattering light intensity of complex micelles to glucose concentration. The pKd (dissociation constant) of boronic acid is about 9.3, so PNIPAM-b-PAPBA and (GA-PNIPAM)/Eu(III) exhibit the strongest complexation at pH value of 9.3. In order to study its biological properties, this study takes pH value of 7.4 as an example. The scattering light intensity was decreased with the increase of glucose concentration. The reason is the competition between GA-containing blocks and glucose, it can cause the complex micelles dissociation [Citation25]. There is no aggregation of large particles in solution, which may result in a stronger generation of phenylborates.
3.3. Assessment of cell viability
The (GA-PNIPAM)/Eu(III), (PNIPAM-b-PAPBA) and complex micelles were used to evaluate the cytotoxicity on L-929 and MCF-7(). The percent of cell viability was determined by comparison with cells that were not exposed to samples, which were used as the control group. All three compounds were toxic in either cell line over a broad concentration range from 0.10 to 1000.00μg/ml. By introducing the hydrophilic monomer GA into the PNIPAM chain, the (GA-PNIPAM)/Eu(III) and complex micelles made the L-929 cells remained at a high level increasing over 100%, and the viability of the MCF-7 cells decreasing to approximately 80%.
4. Conclusions
The novel thermo-, pH- and glucose-responsive polymeric nanoparticles were fabricated by the cross-linking between PBA of PNIPAM-b-PAPBA and GA of GA-PNIPAM/Eu(III) to form complex micelles. The nanoparticles exhibited strong fluorescence and a fluid dynamics diameter approximately 80 nm at pH = 7.4. MTT assays revealed the nanocarriers had no significant cytotoxic response. The nanoparticles will have potential applications for glucose-responsive drug delivery for diabetes treatment.
Supplemental Material
Download PDF (633.3 KB)Acknowledgments
We are grateful to the Jilin science & technology department, the science and technology development project (Nos. 20180101194JC, 20180101187JC 20170520149JH, and 20180520009JH), Jilin Province Science and Technology project of traditional Chinese medicine (2017103), Jilin Province Education Department the Science and Technology development project (Nos. JJKH20181117KJ and JJKH 20191059KJ), Jilin Province Health and health Commission (No. 2016Q053, 2018J114), Jilin City Science and Technology Innovation development project (2019301119) and National College Student Innovation Training Program (201913706004).
Disclosure statement
No potential conflict of interest was reported by the authors.
Supplementary material
Supplemental data for this article can be accessed here.
Additional information
Funding
References
- Guan Y, Zhang Y. Boronic acid-containing hydrogels: synthesis and their applications. Chem Soc Rev. 2013;42(20):8106–8121.
- Ma R, Shi L. Phenylboronic acid-based glucose-responsive polymeric nanoparticles: synthesis and applications in drug delivery. Polym Chem. 2014;5(5):1503–1518.
- Kuivila HG, Keough AH, Soboczenski EJ. Areneboronates from diols and polyols1. J Org Chem. 1954;19(5):780–783.
- Brooks WLA, Sumerlin BS. Synthesis and applications of boronic acid-containing polymers: from materials to medicine. Chem Rev. 2016;116:1375–1397.
- Matsumoto A, Miyahara YJ. Borono-lectin based engineering as a versatile platform for biomedical applications. Sci Technol Adv Mater. 2018;19:18–30.
- Farooqi ZH, Wu W, Zhou S, et al. Engineering of phenylboronic acid based glucose-sensitive microgels with 4-vinylpyridine for working at physiological pH and temperature. Macromol Chem Phys. 2011;212(14):1510–1514.
- Sato K, Yoshida K, Takahashi S, et al. pH-and sugar-sensitive layer-by-layer films and microcapsules for drug delivery. Adv Drug Deliv Rev. 2011;63(9):809–821.
- Dong Y, Wang W, Veiseh O, et al. Injectable and glucose-responsive hydrogels based on boronic acid glucose complexation. Langmuir. 2016;32(34):8743–8747.
- Chai Z, Ma L, Wang Y, et al. Phenylboronic acid as a glucose-responsive trigger to tune the insulin release of glycopolymer nanoparticles. J Biomater Sci Polym Ed. 2016;27(7):599–610.
- Hoare T, Pelton R. Engineering glucose swelling responses in poly(N-isopropylacrylamide) -based microgels. Macromolecules. 2007;40(3):670–678.
- Wang D, Liu T, Yin J, et al. Stimuli-responsive fluorescent poly(N-isopropylacrylamide) microgels labeled with phenylboronic acid moieties as multifunctional ratiometric probes for glucose and temperatures. Macromolecules. 2011;44:2282–2290.
- Xing SY, Guan Y, Zhang YJ. Kinetics of glucose-induced swelling of P(NIPAM-AAPBA) microgels. Macromolecules. 2011;44:4479–4486.
- Roy D, Cambre JN, Sumerlin BS. Triply-responsive boronic acid block copolymers: solution self-assembly induced by changes in temperature, pH, or sugar concentration. Chem Commun. 2009;2106–2108.
- Shi DJ, Ran MS, Zhang L, et al. Fabrication of biobased polyelectrolyte capsules and their application for glucose-triggered insulin delivery. ACS Appl Mater Interfaces. 2016;22:13688–13697.
- Liu G, Ma RJ, Ren J, et al. A glucose-responsive complex polymeric micelle enabling repeated on-off release and insulin protection. Soft Matter. 2013;9(5):1636–1644.
- Yang H, Zhang C, Li C, et al. Glucose-responsive polymer vesicles templated by α-CD/PEG inclusion complex. Biomacromolecules. 2015;16(4):1372–1381.
- Yang H, Ma RJ, Yue J, et al. A facile strategy to fabricate glucose-responsive vesicles via a template of thermo-sensitive micelles. Poly.Chem. 2015;6(20):3837–3846.
- Kataoka K, Miyazaki H, Bunya M. Totally synthetic polymer gels responding to external glucose concentration: their preparation and application to on-off regulation of insulin release. J Am Chem Soc. 1998;120:12694–12695.
- Komiyama M, Mori T, Ariga K. Molecular imprinting: materials nanoarchitectonics with molecular information. Bull Chem Soc Jpn. 2018;91:1075–1111.
- Zhao LY, Zou QL, Yan XH. Self-assembling peptide-based nanoarchitectonics. Bull Chem Soc Jpn. 2019;92:70–79.
- Cui GH, Bai Y, Gao ZG, et al. Synthesis and characterization novel Eu(III) complexes by End-functionalized poly(N-isopropylacrylamide). Mater Sci Eng C. 2017;78:603–608.
- Cui GH, Gao ZG, Qiu NN, et al. Synthesis and characterization of Eu(III)-based coordination complexes of modified D-glucosamine and poly(N-isopropylacrylamide). Opt Mater. 2017;72:115–121.
- Ciampolini M, Nardi N. Five-coordinated high-spin complexes of bivalent cobalt, nickel, and copper with tris(2-dime thylaminoe thy1)amine. Inorg Chem. 1966;5:41–44.
- Lai JT, Filla D, Shea R. Functional polymers from novel carboxyl-terminated trithiocarbonates as highly efficient RAFT agents. Macromolecules. 2002;35:6754–6756.
- James TD, Shinkai S. Artificial receptors as chemosensors for carbohydrates. Top. Curr Chem. 2002;218:159–200.
- Chunwei S, Jingjing L, Wenyuan W, et al. Toward understanding of the effect of nucleation temperature on porous structure of micro-mesoporous composite molecular sieves and related crystallization mechanism. Catalysts. 2019;9:777. 1-10.
- Roy D, Cambre JN, Sumerlin BS. Triply-responsive boronic acid block copolymers: solution self-assembly induced by changes in temperature, pH, or sugar concentration. Chem Commun. 2009;2106–2108.
- Wang D, Liu SY. Fabrication of multi-responsive interpolymer complex micelles via reversible covalent bond formation between phenylboronic acid and glucose moieties. Scientia Sinica Chimica. 2011;41:351–358.
- Zhang YJ, Guan Y, Zhou SQ. Synthesis and volume phase transitions of glucose-sensitive microgels. Biomacromolecules. 2006;7:3196–3201.