ABSTRACT
Many researchers working on the development of dye-sensitized solar cells (DSCs) continue to focus on the synthesis of photoanode materials with high surface area, along with high light scattering ability to enhance light harvesting efficiency (LHE). Meanwhile, dye packing density, which also impacts the LHE significantly, is often overlooked. Solvothermally synthesized anatase TiO2 nanoparticles (SANP) were obtained by a new and simple approach using a mixed solvent, ethanol and acetic acid. SANP were applied in photoanodes of DSCs using either metal-free organic dye (D149) or organometallic (N719) dyes. Dye loading (packing density) was correlated with the isoelectric point (IEP) in addition to light scattering effects were shown to determine the devices photovoltaic efficiency (PCE); specifically when compared with ones employing commercially available TiO2 nanoparticles. SANP photoanodes sensitized with D149 dye were found to be optimised at 10 µm, yielding a PCE of 6.9%, superior to for transparent or transparent + scattering films from the commercial source (5.6% and 5.9%, respectively). Furthermore, a 7.7% PCE was achieved using a SANP photoanode sensitized with N719 dye, with 7.2% seen for the transparent photoanode and 7.9% with a scattering layer. The high PCEs of of SANP devices are attributed to the high dye loading capability in addition to light scattering. A further point of interest is that even with the increased reactivity of the surface towards dye adsorption, we did not observe any significant increase in recombination with the redox mediator, presumably due to the increased dye loading providing better shielding.
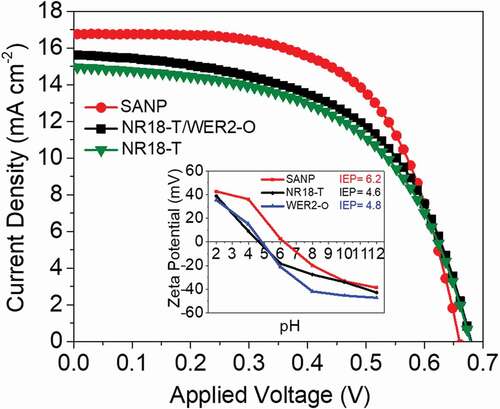
1. Introduction
TiO2 has a long history of use in photoanodes, including famous publications from Fujishima and Honda, as well as the group of Chen [Citation1,Citation2]. A significant increase in the use of this material followed the first DSCs in 1991, as reported by O’Regan and Gratzel in 1991 [Citation3,Citation4], where high surface area nanoparticle TiO2 films sensitized with a dye which injects electrons following photoexcitation [Citation3]. The highest photo-to-electric conversion efficiencies (PCE) under solar equivalent conditions are now over 14% [Citation5].
In spite of the advent of other technologies [Citation6,Citation7] and price decreases of maturing photovoltaic technologies, DSCs are still of interest to researchers, largely due to some of their distinct features, which provide promise for niche applications, such as flexible devices and/or indoor applications, where DSCs do not suffer as greatly from low light intensities, angle of incidence or partial shading as silicon does [Citation8–12].
It is well known that poor light harvesting from dye monolayers necessitate high surface areas and close packing of dyes on these surfaces [Citation3]. As such nanoscale TiO2 particles are standard in DSCs applications [Citation3]. Light scattering is another approach to increasing LHE, with the mean free path of a photon traversing the photoelectrode increased, thereby increasing the likelihood of it being absorbed by a dye [Citation13]. This poses a contradiction for photoanode development, as scattering is primarily controlled by particle (or void) size [Citation14,Citation15]. Bilayer photoanodes, with one layer of non-scattering TiO2 nanoparticles, and one composed of larger particles (400–500 nm) are commonly used, to scatter/reflect the incident light backward through the high surface area nanoparticle region to increase the probability of absorption [Citation16]. In general, thick TiO2 layers should be avoided as they lead to a lowering of the overall charge density in the film, which affects the quasi-Fermi level and subsequently the attainable device voltage [Citation17,Citation18].
The ruthenium complex N719 is still one of the most commonly employed dyes in DSC research, and as such serves as an important benchmark; however, high production/purification costs and low molar extinction coefficients limit widespread commercial viability [Citation19,Citation20]. Further to this, recent record device efficiencies (including the above mentioned 14%) have been attained using dyes employing more earth-abundant metals or metal-free organic compounds [Citation19,Citation21,Citation22]. D149 was one of the first organic dyes to rival the performance of N719 and is widely used in research today as it is commercially available. PCE of up to 9% has been reported [Citation23].
The isoelectric point IEP refers to the pH at which there is zero net surface charge on the material [Citation24]. With all other things being equal, the higher the IEP value a metal oxide surface has, the more negatively charged dye molecules, such as those with carboxylic functionality [Citation24,Citation25], can be adsorbed. This is a key factor in determining the total dye loading on photoanodes [Citation26,Citation27] and although not commonly addressed, has been exploited by several researchers. This includes, most simply, by the use of different semiconductors (i.e. not TiO2), which possess high IEP, such as ZnO or SnO2 [Citation26,Citation27]. This has also been achieved with surface post-treatments (such as TiCl4 or acid washes) [Citation26]. While enhanced LHE due to this increased dye loading may result from high IEP values [Citation26–28], this may also lead to dye aggregation, which can negatively affect overall device performance [Citation27].
Furthermore, dye loading is important outside the specific field of DSCs’ research. One recent report on the use of TiO2 particles for photocatalytic applications showed a significant dependence of the IEP of TiO2 nanoparticles synthesised at different pH values [Citation29]. In this, the use of higher acidic conditions was employed to produce TiO2 nanoparticles with a higher IEP [Citation29]. More details in this regard have been reported elsewhere [Citation30]. As mentioned above, acidic surface post-treatments have been expected to increase dye loading on TiO2 through changes to the IEP [Citation31,Citation32].
It has been widely reported that the dye adsorption capability is also strongly affected by exposed facets of TiO2 crystal structures [Citation33–35]. Increasing the surface energy of the exposed facets can enhance their reactivity to adsorb more dye molecules. Thus, nanostructured TiO2 with exposed facets can adsorb more dye compared to those without specific facets and then enhance DSC performance or photocatalytic activity [Citation35–39]. Many researchers synthesized anatase or rutile TiO2 particles with different morphologies (mostly by solvothermal reaction-based acidic solvents) with a high percentage of specific exposed facets to enhance the dye loading in DSCs [Citation33,Citation36,Citation38–42]. This was explained in term of surface reactivity, according to the theory of surface adsorption, the surface acidity is a function of charge affinity and density of adsorption sites which are mostly the cationic positive ones. These positive adsorption sites can bond strongly to the hydroxyl groups in solvents due to having high IEP [Citation42–45]. Increasing the IEP, such as with acid-based solvents can improve the dye loading, which also increases the LHE significantly [Citation31,Citation32]. While post-treatments of TiO2, like the ones described above, this adds an extra step in production [Citation26–28].
As organic dyes relax the requirements in TiO2 photoanode design few reports, have been published regarding optimization of TiO2 photoanodes sensitized with D149 for DSCs [Citation23,Citation46–48]. In spite of there being a number of publications seeking to optimize devices for D149, these often focused on improving the electrolyte or the LHE of photoanode using a scattering layer. Therefore, increasing of dye loading along with reducing the film thickness and suppressing the recombination losses are required for efficient DSC based on free-metal organic dyes.
In this work, we investigate the effect of surface area, porosity, and the IEP on the dye loading, as well as the dye packing density of N719 and D149 on TiO2, using a newly synthesised material, produced in a solvothermal reaction with a mixture of ethanol and acetic acid. Mixed solvents, including acids, are commonly used in the hydrothermal reaction to control TiO2 morphologies with specific exposed crystal facets that exhibit different dye loading [Citation33,Citation39,Citation49]. Also, if acetic acid can be used to control the exposed crystal facets, it would be much more scalable than using other solvents such as HF or NH3F [Citation50,Citation51].
To the best of our knowledge, the effect of solvothermal reaction conditions on product IEP and subsequently on dye packing in such mixed solvent reactions has not been investigated. As such, we report here a new and facile solvothermal approach to synthesize high surface area anatase TiO2 nanoparticles (20 nm) along with their performances in DSCs using either organometallic N719 dye or organic D149 dye. To more fully understand the differences as compared to using commercially available TiO2, light scattering and dye packing density are investigated, with dye packing density seen to be dependent on IEP.
2. Experimental details
2.1 SANP production
SANP were synthesized by a new and facile one-step solvothermal process. Briefly, Titanium isopropoxide (TTIP) (1.5 ml) was added dropwise to an acetic acid-ethanol mixed solvent (1:2 v/v) under vigorous stirring for (1 h) at room temperature. A clear solution was formed which was transferred into a Teflon-lined stainless steel autoclave, 45 mL (Parr Instrument Company) heated to 200°C (ramp time of 1°C/min) for 9 h, after cooling down to room temperature the resulting white precipitate was collected and washed with distilled water and ethanol three times and then dried overnight at 90°C. Finally, the samples were calcined at 500°C (ramp time of 1°C/min) in the air for three hours (in this procedure the calcination step can be eliminated from the synthesis procedure as the sample showed higher crystallinity even prior to calcination).
2.2 DSC device assembly
A screen printing paste was prepared from SANP, using our previously reported method [Citation52–54]. In brief, organic binders (ethyl cellulose), ethanol, terpineol, acetic acid and water were added to the SANP, mixed and then the low boiling point solvents were evaporated using a rotary evaporator (leaving terpineol) [Citation52]. Using this paste, 10 µm films of SANP were screen printed onto cleaned FTO glass for use as photoanodes, along with commercial transparent TiO2 (Dyesol NR18-T) and in some cases also a scattering layer (Dyesol-WER2-O). Prior to this porous film, a dense layer was formed through the spray pyrolysis of titanium (IV) diisoproxide-bis-acetylacetonate (75 wt.% in isopropanol, Aldrich) solution (1:9 v/v in ethanol) at 450°C). After that, the printed photoanodes were sintered using a multi-step program (up to 500°C). Finally, the photoanodes were surface treated by soaking the photoanodes in a (20 mM) solution of TiCl4 (aq., Sigma) for 30 min at 70°C, then washed and re-sintered at (500°C for 30 min). Subsequently photoanodes were immersed in either a 0.5 mM N719 (Solaronix) dye solution of a mixture of tert-butanol (LR, Ajax Chemicals) and acetonitrile (HPLC, Lab-scan) [1:1 v/v], or 0.5 mM D149 (1-material, Canada) in a 1:1 (v/v) mixture of acetonitrile and tert-butanol. The photoanodes were taken out from the dye solution after 24 h and washed with acetonitrile and then dried.
Counter electrodes were prepared by first drilling holes in a separate piece of FTO glass, to be used as a filling port for the electrolyte solution. One drop of H2PtCl6 solution (10 mM in ethanol) was smeared on the cleaned pre-FTO counter electrode and heating to 400°C for 20 min. The counter electrodes are cooled before being sandwiched together with the photoanode, using a 25 μm Surlyn (Solaronix) spacer, by a hot press. The electrolyte solutions [acetonitrile/valeronitrile (85:15 vol %), 0.03 M iodine (I2), 0.5 M 4-tertbutyl pyridine (4-tBP), 0.6 M 1-butyl-3-methylimidazolium iodide (BMII), and 0.1 M guanidinium thiocyanate (GuSCN)] for N719 or [0.05 M iodine (Sigma), 0.6 M 1,2-dimethyl-3-propylimidazelium iodide (Solaronix), 0.1 M lithium iodide (Sigma) in methoxypropionitrile (Sigma)] for D149 were introduced into the filling port by a vacuum back-filling technique, and the filling port was then closed with a piece of Surlyn laminated to aluminium foil.
2.3 Characterization
The crystalline structure of SANP and commercial transparent titania NR18-T were examined using X-ray diffractometer (MMA, GBC Scientific Equipment LLC, Hampshire, Illinois, USA, 40 kV, 30 mA, Cu Kα radiation, λ = 1.5406 Å) in the range of 2θ = 20–80°, with a scan rate of 1°/min. The morphology and internal structure were examined by transmittance electron microscopy (TEM, JEOL JEM-2010). Microtrac Belsorp-mini nitrogen adsorption-desorption equipment was used to obtain specific surface area and pore volume values. The amount of N719 and D149 dyes on the different films was calculated by measuring the absorbance of dyes desorbed from the films (thickness = 5 µm, area = 1 cm2) in 4 ml of 0.1 M NaOH solution (distilled water: ethanol 1:1 v/v) for N719 or in 4 ml of 0.4 M NaOH in methanol for D149 [Citation35,Citation52] using a Shimadzu UV-1800 spectrophotometer. The diffuse reflection and the optical energy gap were conducted using an integrating sphere attachment (ISR-3100) and a Shimadzu UV-3600 spectrophotometer. The isoelectric points of the materials (0.01 M aqueous dispersions with 2 < pH < 12, adjusted using either 0.25 M NaOH or 37% v/v HCl) were measured using Zetasizer Nano-ZS (Malvern). A Shimadzu IR prestige-21 was used to measure fourier transform infrared spectroscopy (FTIR) spectra of undyed NR18-T and SANP films (3 µm thicknesses). The transmittance spectra of materials in FTIR measurements were normalized to make a reasonable comparison. A Horiba Jobin Yvon HR800 confocal Raman spectrometer equipped with a 632.8 nm laser was used to measure Raman shift of NR18-T and SANP films (3 μm). The Raman spectra were measured in the range of 100–800 cm−1 wavenumber range. A SPECS PHOIBOS 100 X-ray photoelectron spectroscopy (XPS) analyser was used with a high-vacuum chamber at the pressure below 10–8 mbar. X-ray beam was produced using Al Kα radiation source with energy 1486.6 eV, a voltage of 12 kV, and a power of 144 W. The XPS binding energy spectra of SANP and NR18-T samples were recorded at the pass energies of 20 eV, with a step of 0.05–0.1 eV in the fixed analyser transmission mode. The full survey scans of SANP and NR18-T samples were measured at the pass energies of 60 eV and a step of 0.5 eV. Casa XPS software package was used for analysis of XPS data. Thermogravimetric analysis (TGA) (STAR system, Mettler Toledo) was used to measure the weight loss of SANP and NR18-T powders (35 mg) in the temperature range (25–900°C) under air. A Veeco Dektak 150 Surface Profiler was used to determine the film thicknesses. Photocurrent density-voltage (J-V) measurements were measured using a solar simulator with an AM1.5 filter; set to 1 sun (100 mW/cm2, PV Measurements, Colorado, USA). A QEX10 system from (PV Measurements) was used for the incident to photocurrent conversion efficiency (IPCE) measurements in 5 nm steps. A Reference 600 Potentiostat (GAMRY instrument) was used for electrochemical impedance spectroscopy measurements (EIS) which were carried out for DSCs based on different photoanodes under 1 sun illumination at Voc in a frequency range (0.1–106 Hz) and AC voltage 10 mV.
3. Results and discussion
3.1 Electron microscopy
TEM images ( and ) show the morphologies of solvothermally prepared nanoparticles (SANP) and commercial transparent titania nanoparticles (NR18-T). As can be seen, SANP have a more uniform, if aggregated, structure, consisting of 20 ± 5 nm nanoparticles. Meanwhile, NR18-T are comprised of two particle shapes, including spindle-like particles 32 ± 6 nm long and 10 ± 2 nm diameter, as well as 10 ± 3 nm spherical nanoparticles. The insets in and b show the shape morphologies of SANP and NR18-T. The shape of SANP is mainly attributed to the different degree of truncation of the octahedral structure during the solvothermal reaction [Citation55,Citation56]. While NR18-T samples have two different shape morphologies, those being spindle and spherical particles, the percentage of high energy exposed (001) facets is expected to be higher for the truncated SANP shape compared to spindle NR18-T shape. These differences in SANP and NR18-T morphologies are mainly result from the difference in synthesis conditions.
3.2 X-Ray diffraction (XRD)
XRD patterns () confirmed that SANP nanoparticles are phase pure anatase TiO2 (JSPD.21–1272) with good crystallinity, which is quite similar to NR18-T. However, all the peaks of SANP are narrower than those of NR18-T indicating smaller particle size of the former. In addition to being phase pure, neither sample shows any significant broad hump, which could be associated with the presence of amorphous material. This is further explored using the reference intensity ratio method (see Figure S1), with around 3% amorphous content determined to be present in SANP and 4% for NR18-T [Citation57] [Citation58] It is therefore assumed this amorphous content would only slightly affect the dye loading capability on SANP or NR18-T [Citation59].
Meanwhile, the crystallinity of the anatase phase was further confirmed with high-resolution TEM images (HRTEM) and selected area electron diffraction patterns (SAED, Figure S2). It is noteworthy that SANP showed high crystallinity even prior to calcination (as shown in Figure S3), suggesting it to be particularly useful in low-temperature manufactures, such as flexible DSCs. Uniform morphology of SANP with high surface area and appropriate pore size distribution are expected to enhance the dye loading compared to NR18-T [Citation60,Citation61]. shows the nitrogen adsorption/desorption isotherms measurements and the inset the pore size distributions of SANP, NR18-T and commercial reflector particles WER2-O (used as a scattering layer). SANP samples have a higher surface area and larger mesoporous structure, observed from the larger isotherm loop compared to both NR18-T and WER2-O materials, while the total porosity of SANP is comparable to that of NR18-T, and these are both much higher than that of WER2-O (). The average pore size distribution of SANP is centred at 20 nm which is smaller than for NR18-T at 37 nm, while for WER2-O it is >120 nm which is not seen here due to equipment limitations. HRTEM images (Figure S3b) also show that SANP contains a significant number of micropores prior to calcination. It can be observed that SANP have more uniform particle distribution compared to NR18-T, resulting in the formation of a uniform porous structure compared to NR18-T as shown in Figure S4.
Figure 1. TEM images of (a) SANP and (b) NR18-T; the insets are the shape morphologies of SANP and NR18-T; (c) XRD patterns; and (d) Nitrogen adsorption-desorption isotherm measurements and the inset is the pore size distribution calculated by the Barrett-Joyner-Halenda (BJH) method
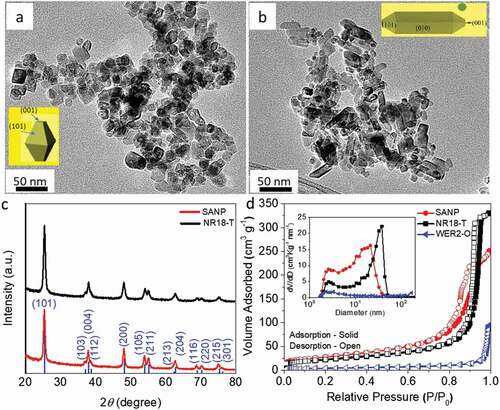
Table 1. The porosity and specific surface area of SANP, 18NR-T and WER2-O materials. a Porosity calculated as P = PV/(ρ−1 + PV), where PV is the cumulative pore volume (cm3·g−1), and ρ−1 is the inverse of the density of anatase (0.257 cm3·g−1) [Citation54]
3.3 Solar performance for SANP sensitized with D149 dye
Photovoltaic performance of DSCs based on SANP photoanodes (10.0 ± 0.5 µm) was compared with those based on only NR18-T (also 10.0 ± 0.5 µm) or NR18-T (6.0 ± 0.5 µm)/WER2-O (5.0 ± 0.5 µm). We selected the 10 µm film thickness as it resulted in the highest PCE performance in DSCs using D149 (Table S1, Table S2 and Figure S5). a, b and and S3 show the higher PCE and IPCE maxima of DSCs’ devices based on SANP compared to those utilising NR18-T or NR18-T/WER2-O photoanodes. From ~400 nm to ~650 nm IPCE of SANP is consistently above the other two, in this region, the LHE will be close to 100%. All devices here display similar open circuit voltages (Voc). However, the fill factors (FF) and current densities (Jsc, also seen in IPCE responses) are appreciably higher with the SANP photoanodes than with the other two systems tested here.
Table 2. J-V parameters of devices based on SANP, 18NR-Tand 18NR-T/WER2-O sensitized with D149
Jsc is determined by the combination of three factors, specifically, the charge injection efficiency (Φinj), charge collection efficiency (Φcc) and LHE, which is itself dependent on dye absorption coefficients, dye loading and light scattering ability, as this affects the pathlength of light. It seems reasonable to assume that the Φinj will be similar between dyes on SANP and NR18-T (of course there may be reasons for this not to be the case), while the Φcc is assumed to be slightly higher for SANP photoanode based on EIS results below.
The LHE of SANP, compared to NR18-T and WER2-O, sensitized with D149 was investigated by using the quantified amount of desorbed dye from the photoanodes films (area = 1 cm2, thickness = 5 μm, which was desorbed in 4 ml of 0.4 M NaOH in methanol), as shown in the dye loading data (, ) (overnight dyeing time was employed to ensure the equilibrium was attained, c.f. Kim et al. [Citation62]). Figures S6 a, b show a lower-bound estimate of the LHE, based on the dye loading results. According to this, the LHE of the dyed SANP films is higher than those of NR18-T or WER2-O (with more broadening toward 700 nm) which enable it to harvest more light in this range. SANP films show higher dye loading compared to NR18-T and NR18-T/WER2-O photoanodes when normalised against the surface area to give dye packing (dyes nm−2). SANP again was shown to have superior dye hosting abilities, compared to the other TiO2 materials (). This is attributed to the higher IEP, which favours the binding of negatively charged dyes, as the surface is itself positively charged [Citation26,Citation27]. IEP values obtained from Zeta potential measurements are shown in . In addition to this, undyed SANP films showed higher diffuse reflection than that of NR18-T although lower than that of WER2-O films (), indicating good light scattering, and extended pathlength of light though SANP films compared to NRA8-T. shows the photographs of the three undyed photoanode configurations. This data was transposed to form Tauc plots (Figure S7), which shows no significant differences in terms of the optical bandgap of these materials.
Figure 2. (a) J-V characteristics measured under 1 sun illumination with an area of 0.16 cm2 of SANP, NR18-T- and NR18/WER2-O-based D149 devices; (b) IPCE; and (c) the absorbance spectra of desorbed D149 dye from films using 4 ml of 0.4 M NaOH in methanol, film thickness = 5.0 ± 0.3 µm and film area = 1 cm2
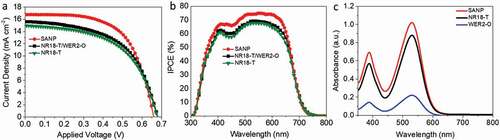
Figure 3. (a) IEP measurements of SANP, NR18-T and WER2-O in aqueous solutions (0.01 M) using different pH values; (b) diffuse reflections of SANP, NR18-T and WER2-O films (10.0 ± 0.3 µm thick); and (c) the visual image of photoanode films
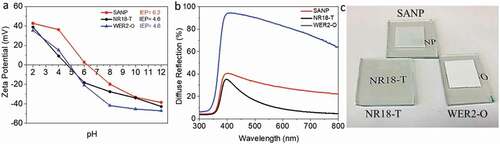
Table 3. The amount of D149 dye loading on SANP, 18NR-Tand films (thickness = 5.0 ± 0.3 µm; Area = 1 cm2) calculated using desorption method [Citation63]
In addition to examining the phase and crystallinity of the materials, Raman spectroscopy can also provide information about the percentage of specific exposed (001) facets of anatase as shown in Figure S8. The percentage of exposed (001) facets of SANP and NR18-T are quantified to be 8% and 7% respectively. While the slightly higher percentage of exposed facets (001) of SANP may slightly affect the IEP (and the number of available binding sites), this is not enough of a difference to explain the ~40% difference in dye packing density [Citation42].
3.4 FTIR analysis
Attenuated total reflectance-fourier transform infrared spectroscopy (ATR-FTIR) spectroscopy was employed to examine the active surface binding sites on undyed SANP and NR18-T films.
shows the ATR-FTIR spectra of SANP and NR18-T. The band at 3330 cm−1 is the broadest in each sample (slightly broader in SANP than NR18-T) and corresponds to the stretching vibration of the hydroxyl group (OH). The band at 1637 cm−1 corresponds to bending modes of Ti-OH, while the two peaks at 1550 cm−1 and 1417 cm−1 observed in SANP originate from the asymmetric and symmetric stretching modes of carboxylic groups, which are typically seen for TiO2 synthesised using acidic precursor solutions and calcined at only relatively low temperatures (100–250°C) [Citation64–66]. The asymmetric carboxylic group coordinated with Ti (1417 cm−1), as claimed, can be observed even after high sintering temperature [Citation67]. On the other hand, the bands 1637 cm−1 and 1417 cm−1, corresponding to Ti-OH and symmetric COOH were also observed in NR18-T while the asymmetric COOH was significantly smaller.
As acetic acid was involved in SANP synthesis, it is possible that this could remain, adsorbed on TiO2 surface by a strong bidentate mode, resulting in Ti-COOH bond [Citation68,Citation69]. During the dye adsorption process, these would presumably be replaced by the carboxylic acid groups on the dye itself, however. The specific mechanism and its relation to IEP and charge injection are however still not fully understood and will be the subject of further investigation. There also arose a degree of ambiguity from FTIR results, as to whether the observed species are residual groups following synthesis (and a 500°C sintering process) or adsorbed from the atmosphere due to the high IEP providing a favourable substrate for the binding of CO2/H2O. In order to address this, mass losses in SANP and NR18-T were measured up to 900°C using thermogravimetric analysis (TGA), as shown in . At lower temperatures (100–400°C) SANP shows more substantial mass loss than NR18-T, which is ascribed to adsorbed H2O. Above this, in the range of 400–600°C, the mass of SANP was more stable, while a gradual decrease in NR18-T was observed [Citation66]. The notable weight loss observed in SANP above 700°C, well above the temperatures experienced during device production, indicates the removal of some strongly bound residuals. Unfortunately, we are unable to confirm the identity of this with our present setup, although it seems most likely to be COOH, in line with observations from FTIR analysis. This residual organic components, which exists in SANP after synthesis, but is not observed in NR18-T, appear to play a role in enhancing the SANP surface reactivity and hence the dye packing density.
The surface compositions and the chemical states of SANP and NR18-T were further examined by XPS to investigate the surface functionality of SANP and NR18-T as shown in Figures S9 and S10 a-c. It can be noted that the relative amount of OH to O in SANP (64% vs 36%) is higher than that of NR18-T (52% vs 48%) as shown in Figures S10 c, d. These results are consistent with FTIR; however, these differences are also not sufficient to explain the dye packing differences.
From FTIR, TGA and XPS analysis SANP have a higher amount of adsorbed OH along with the presence of asymmetric carboxylic group compared to NR18-T probably due to the use of acetic acid and ethanol in the solvothermal reaction. This can increase the IEP resulting in a more positive surface charge on SANP; and thus can promote more dye adsorption leading to higher dye packing density.
3.5 EIS analysis
EIS was carried out on D149 sensitized devices under 1 sun illumination, to compare electron transfer rates and lifetimes. The results are shown as both Nyquist and Bode plots in a, b, respectively, with fitting shown in Figure S11. These fits utilized the model proposed by Bisquert and co-workers [Citation70,Citation71], with parameters shown in . Here, Rs is the series resistance, Rct1 and Rct2 are the charge transfer resistance at the counter and the working electrode, respectively, while CPE1 and CPE2 are the constant phase elements associated with the counter and working electrodes, respectively. In all Nyquist plots, two semicircles were observed, with the first, corresponding to high frequencies, representing charge transfer resistance at the counter electrode/electrolyte interface (Rct1). As expected these were similar for all devices tested. The second semicircle (mid-range and low frequencies) represents the impedance of the interface between the photoanode material and liquid electrolyte in competition with the transfer of injected electrons through the photoanode material (charge transfer resistance, Rct2) [Citation70,Citation72]. Devices based on SANP have a slightly lower electrochemical impedance to charge transport through photoanodes compared to those of NR18-T and NR18-T/WER2-O photoanodes (). Bode plots, in , reveal that the local impedance maxima (ƒmax, low frequencies) of SANP-based devices occurs at a lower frequency (16 Hz) compared to those using either NR18-T and NR18-T/WER2-O, which are at 25 Hz. Subsequently, electron lifetimes calculated as τn = 1/2πfmax [Citation73] are seen to be slightly longer, indicative of reduced electron recombination, in line with higher FF values. Devices based on SANP also have higher chemical capacitance (Cµ) values compared to NR18-T and NR18-T/WER2-O, indicating higher electron density (i.e. this should be expected due to the higher surface area of SANP) [Citation35]. The EIS measurements indicate that SANP devices have better Φcc compared to NR18-T and NR18-T/WER2-O devices.
Figure 5. EIS measurements (a) Nyquist and (b) Bode plots of devices based on SANP, 18NR-T and 18NR-T/WER2-O sensitized with a D149 dye
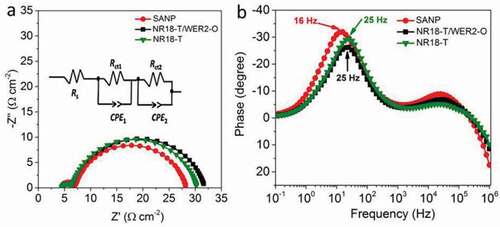
Table 4. EIS parameters of devices based on SANP, 18NR-T and 18NR-T/WER2-O sensitized with a D149 dye
As was discussed above, and shown in Figure S6, the LHE across a large portion of the spectrum (350 < 620 nm) is near unity for all the films presented here. Additionally, the Φcc of SANP devices is only slightly higher, while the peak IPCE values are significantly more for devices with SANP compared to NR18-T or bilayer films, suggesting that Φinj is higher on SANP and therefore, the main reasons for the improvement in SANP/D149 performance [Citation74,Citation75].
3.6 Solar performance for SANP sensitized with N719 dye
Devices based on the three photoanode configurations were also produced and characterised using N719 to investigate the effects of dye packing density, charge injection, charge collection on the DSC performance using such low extinction coefficient dye ( a, b, and and S4). Here the SANP again showed slightly higher Jsc as compared to devices based on transparent NR18-T photoanodes and comparable ones to those of devices based on NR18-T/WER2-O. It was also again observed that the use of SANP results in higher dye loading values, again attributed to higher surface area and packing density (c.f IEP, , and dye loading measurements below in , S12 a, b and ). For consistency with the above section, the same TiO2 film thicknesses (10 μm) were employed and also the overnight dyeing time was employed to ensure the equilibrium was attained. Figures S12 a, b show a lower-bound estimate of the LHE, based on N719 dye loading results. According to this, the LHE of the dyed SANP films is higher than that WER2-O (with more broadening toward 700 nm) which enable it to harvest more light in this range; however, it is similar to that of NR18-T with slightly increase in the range of 700–800 nm. As can be seen with devices based on SANP photoanodes showing average PCEs of 7.7%, higher than for devices based on transparent NR18-T photoanodes (7.2%) and the same (within one standard deviation) as devices employing the scattering layer (NR18-T/WER2-O, 7.9%).
Table 5. J-V parameters of devices based on SANP, 18NR-Tand 18NR-T/WER2-O sensitized with an N719 dye
Table 6. N719 dye loading measurements of SANP, 18NR-T and WER2-O films (thickness = 5.0 ± 0.3 µm; Area = 1 cm2)
The peak IPCE of SANP-based devices is higher than that of NR18-T and the same, within experimental uncertainty, to that of devices based on NR18-T/WER2-O. On the other hand, devices using the latter photoanodes showed a broadening of the response into the range of 600–750 nm, due to the superior scattering effect of large reflector particles (WER2-O, refer back to ). All devices show similar Voc while SANP-based devices showed slightly lower FF than others. The slightly lower FF of SANP/N719-based devices suggests possible higher charge recombination compared to others. This is investigated in more depth below, with EIS again being employed to probe charge transport properties.
Figure 6. (a) J-V characteristics of devices measured under 1 sun illumination, active area = 0.16 cm2, (b) IPCE, (c) the absorbance spectra of desorbed N719 dye from SANP, NR18-T and WER2-O films (5.0 ± 0.3 µm thick)
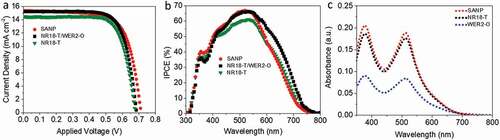
Nyquist plots for N719 sensitized DSCs ( and S13 and ) confirm SANP devices to have higher Rct2 compared to NR18-T and NR18-T/WER2-O-based devices. On the other hand, all N719 devices showed the same fmax of 25 Hz in Bode plots seen in , indicating that the τn,s are approximately the same. The higher recombination rate, related to lower FF in SANP photoanodes, may be attributable to the higher surface area of SANP nanoparticles [Citation15,Citation76], although there is no significant difference in the Cµ for any of the N719 devices, indicating similar charge density through photoanodes materials. It is worthy of mentioning that the trend is reverse in regards to the recombination rate in N719- and D149-based devices. The lower recombination rate (higher FF) observed in D149 devices may be attributed to the higher charge injection; however, more investigation is required as future work for further understanding.
Table 7. EIS parameters of devices based on SANP, 18NR-Tand 18NR-T/WER2-O sensitized with N719 dye
It has been shown that N719 is effective at blocking electron recombination, limiting the access of I3− to open space on the TiO2 surface. The same is not necessarily true normally with organic dyes such as D149, and as such, it can be conjectured that the higher dye loading of D149 on SANP may provide a more substantive benefit in terms of blocking possible recombination sites. In addition to this, while high dye loading has also been shown to lead to aggregation, with negative impacts upon device performance, this does not appear to be a major issue here.
Figure 7. (a) Nyquist and (b) Bode plots of devices based on SANP, NR18-T and NR18-T/WER2-O photoanodes sensitized with N719 dye
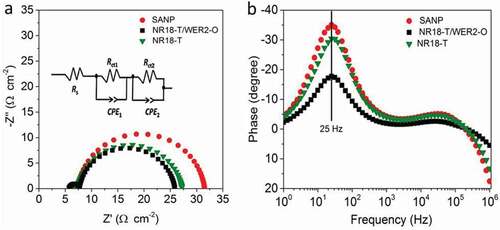
Devices based on SANP|D149 showed better photovoltaic performance (higher Jsc and FF with consistent Voc) compared to devices based on NR18-T/D149 and NR18-T/WER2-O/D149 due to higher LHE and lower recombination rate with longer τn. As for SANP|N719 devices, they showed higher performance compared to NR18-T|N719 while it is slightly lower than NR18-T/WER2-O|N719 due to the higher recombination (Φcc) even with similar τn, due to higher Rct2 at SANP/dye/electrolyte interfaces. In spite of the particle interfaces resulting from higher surface area of SANP can increase the Rct2. Also, more dye coverage seen on SANP can reduce the recombination with electrolyte as the dye layer works as a barrier between the semiconductor and the electrolyte [Citation59]. However, the slightly higher performance of NR18-T/WER2-O|N719 is mainly attributed to the lower charge recombination (slightly higher FF).
The comparable device performances of SANP|N719 against NR18-T/WER2-O|N719 is attributed to similar (IPCE max, LHE and Φcc) assuming similar Φinj.
The enhanced performance of DSCs based SANP|D149 compared to those using commercial TiO2 is mainly attributed to the higher Φinj, higher IEP and higher surface area which enables efficient dye loading/packing density. The IEP of SANP is probably enhanced as a result of the solvothermal synthesis process using a mixed solvent of acetic acid and ethanol. This approach can be used to increase the dye packing density and thus enhance the Φinj in DSC. Further investigation is required for more understanding to the reasons why D149 devices are expected to show higher Φinj in term of affecting the residual chemical or adsorbed species observed in FTIR, TGA and XPS on the dye loading/binding and thus Jsc. This could be investigated for devices based on very thin films as a future application.
4. Conclusions
TiO2 nanoparticles were synthesized by a new and facile solvothermal method, with large specific surface area and high IEP, both of which favour dye loading. SANP photoanodes showed reasonable light scattering due to aggregation. From LHE, EIS and IPCE, it appears that SANP devices showed higher Φinj which led to higher Jsc for devices based on only nanoparticles photoanode compared to devices based on commercial transparent NR18-T nanoparticles photoanode or bilayer photoanode consisted of transparent nanoparticles and large scattering particles NR18-T/WER2-O sensitized with D149 dye. In addition, SANP|D149 devices showed longer values of τn,s and lower Rct2 through the TiO2 network resulting in slight enhancement in the Φcc. As the IPCEs of SANP|D149 are higher than those of NR18-T or NR18-T/WER2-O across a fairly broad region, and by a consistent amount, this suggests differences in Φinj. It is however not clear why this would be the case, and why the same would not be true of N719. This is an area of continued research. Devices based on 10 µm SANP|N719 did show higher Jsc and PCEs compared to NR18-T|N719, in spite of slightly lower FF values. This was largely attributed to light scattering effects, which are more pronounced when using low extinction co-efficient dyes (D149 has a higher extension coefficient compared to N719).
The synthesis procedure using a mixed solvent ethanol and acetic acid (2:1 v/v) used here produced SANP with higher IEP along with higher surface area which enabled higher dye packing density and thereby enhanced the DSC performance. This work sheds light on the role of the IEP of synthesized TiO2 metal oxide on DSC performance which may also be useful for other applications required more reactive surface materials.
Supplemental Material
Download PDF (1 MB)Acknowledgments
The authors would like to acknowledge the Australian National Fabrication Facility (ANFF) and Electron Microscope Center (EMC) for access to equipment. Dr Andrew Nattestad would like to acknowledge the ARC for funds. Kadhim Al-Attafi would like to thank Dr. Patricia Hayes for assistance on running the FTIR and Raman measurements and the Higher Committee for Education Development in Iraq (HCED) for financial support.
Disclosure statement
No potential conflict of interest was reported by the authors.
Supplemental material
Supplemental data for this article can be accessed here.
Additional information
Funding
Notes on contributors
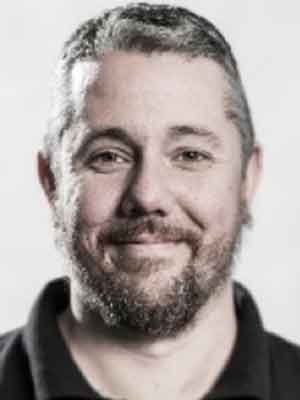
Andrew Nattestad
Dr. Andrew Nattestad is a Senior Research Fellow at the University of Wollongong (UOW), working in the Intelligent Polymer Research Institute (IPRI). He completed his PhD at Monash University with his thesis, “the development of photocathodes for tandem dye-sensitised solar cells”. At UOW he has continued to implement third generation photovoltaic concepts into dye-sensitized and organic solar cells, as well as expanding into other areas including a number of photocatalytic systems.
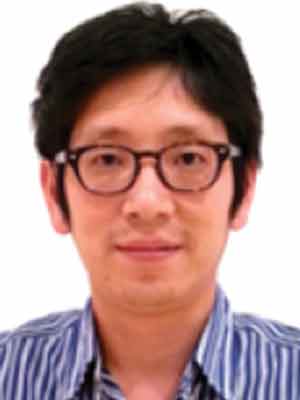
Jung Ho Kim
Prof. Jung Ho Kim is currently tenured Professor at the Institute for Superconducting and Electronic Materials (ISEM), Australian Institute for Innovative Materials (AIIM), University of Wollongong, Australia. He received his Bachelor’s (1998), Master’s (2000), and PhD (2005) degrees from Sungkyunkwan University, Korea. He is currently acting as an editorial board member for Scientific Reports (Nature Publishing Group) and Associate Editor for Science and Technology of Advanced Materials. His major research is the rational design of materials for energy storage and harvesting applications.
References
- Chen S, Russak MA, Witzke H, et al. Photoelectrochemical cell. United States Patent, Publication of US4172925A. 1979.
- Fujishima A, Honda K. Electrochemical photolysis of water at a semiconductor electrode. Nature. 1972;238(5358):37–38.
- O’Regan B, Grätzel M. A low-cost, high-efficiency solar cell based on dye-sensitized colloidal TiO2 films. nature. 1991;353(6346):737–740.
- Deb S, Ellingson R, Ferrere S, et al. Photochemical solar cells based on dye-sensitization of nanocrystalline TiO2. in AIP Conference Proceedings. AIP, United States; 1999.
- Kakiage K, Aoyama Y, Yano T, et al. Highly-efficient dye-sensitized solar cells with collaborative sensitization by silyl-anchor and carboxy-anchor dyes. Chem Comm. 2015;51(88):15894–15897.
- Tsai H, Asadpour R, Blancon J-C, et al. Light-induced lattice expansion leads to high-efficiency perovskite solar cells. Science. 2018;360(6384):67.
- Meng L, Zhang Y, Wan X, et al. Organic and solution-processed tandem solar cells with 17.3% efficiency. Science. 2018;361(6407):1094–1098.
- Rasheduzzaman M, Pillai PB, Mendoza ANC, et al. A study of the performance of solar cells for indoor autonomous wireless sensors. In 2016 10th International Symposium on Communication Systems, Networks and Digital Signal Processing (CSNDSP), IEEE, Prague, Czech, Republic. 2016.
- Yoon S, Tak S, Kim J, et al. Application of transparent dye-sensitized solar cells to building integrated photovoltaic systems, Building and Environment. V.46. 2011.
- Freitag M, Teuscher J, Saygili Y, et al. Dye-sensitized solar cells for efficient power generation under ambient lighting. Nat Photonics. 2017;11(6):372.
- Weerasinghe HC, Huang F, Cheng Y-B. Fabrication of flexible dye sensitized solar cells on plastic substrates. Nano Energy. 2013;2(2):174–189.
- Yoon S, Tak S, Kim J, et al. Application of transparent dye-sensitized solar cells to building integrated photovoltaic systems. Build Environ. 2011;46(10):1899–1904.
- Dembele KT, Selopal GS, Soldano C, et al. Hybrid carbon nanotubes–TiO 2 photoanodes for high efficiency dye-sensitized solar cells. J Phys Chem C. 2013;117(28):14510–14517.
- Koo H-J, Kim YJ, Lee YH, et al. Nano-embossed hollow spherical TiO2 as bifunctional material for high-efficiency dye-sensitized solar cells. Adv Mater. 2008;20(1):195–199.
- Sawatsuk T, Chindaduang A, Sae-Kung C, et al. Dye-sensitized solar cells based on TiO2–MWCNTs composite electrodes: performance improvement and their mechanisms. Diam Relat Mater. 2009;18(2–3):524–527.
- Wang Z-S, Kawauchi H, Kashima T, et al. Significant influence of TiO2 photoelectrode morphology on the energy conversion efficiency of N719 dye-sensitized solar cell. Coord Chem Rev. 2004;248(13–14): 1381–1389.
- Hore S, Vetter C, Kern R, et al. Influence of scattering layers on efficiency of dye-sensitized solar cells. Sol Energy Mater Sol Cells. 2006;90(9):1176–1188.
- Deepak TG, Anjusree GS, Thomas S, et al. A review on materials for light scattering in dye-sensitized solar cells. RSC Adv. 2014;4(34):17615–17638.
- Joly D, Pellejà L, Narbey S, et al. A robust organic dye for dye sensitized solar cells based on iodine/iodide electrolytes combining high efficiency and outstanding stability. Sci Rep. 2015;4(1):4033.
- Ito S, Murakami TN, Comte P, et al. Fabrication of thin film dye-sensitized solar cells with solar to electric power conversion efficiency over 10 %. Thin Solid Films. 2008;516(15): 4613–4619.
- Zeng W, Cao Y, Bai Y, et al. Efficient dye-sensitized solar cells with an organic photosensitizer featuring orderly conjugated ethylenedioxythiophene and dithienosilole blocks. Chem Mater. 2010;22(5):1915–1925.
- Kakiage K, Aoyama Y, Yano T, et al. An achievement of over 12 percent efficiency in an organic dye-sensitized solar cell. Chem Comm. 2014;50(48):6379–6381.
- Ito S, Zakeeruddin SM, Humphry-Baker R, et al. High-efficiency organic-dye-sensitized solar cells controlled by nanocrystalline-TiO2 electrode thickness. Adv Mater. 2006;18(9):1202–1205.
- Park H, Yang D-J, Yoo J-S, et al. Surface passivation of highly ordered TiO2 nanotube arrays and application to dye-sensitized solar cells using the concept of isoelectric point. J Ceram Soc Jpn. 2009;117(1365):596–599.
- Wang K, Shi Y, Guo W, et al. Zn2SnO4-based dye-sensitized solar cells: insight into dye-selectivity and photoelectric behaviors. Electrochim Acta. 2014;135:242–248.
- Son D-Y, Lee C-R, Shin H-W, et al. Understanding the role of the dye/oxide interface via SnO 2 -based MK-2 dye-sensitized solar cells. Phys Chem Chem Phys. 2015;17(23):15193–15200.
- Ulusoy TG, Ghobadi A, Okyay AK. Surface engineered angstrom thick ZnO-sheathed TiO 2 nanowires as photoanodes for performance enhanced dye-sensitized solar cells. J. Mater. Chem. A. 2014;2(40):16867–16876.
- Li X, Yu Q, Yu C, et al. Zinc-doped SnO 2 nanocrystals as photoanode materials for highly efficient dye-sensitized solar cells. ?J Mater Chem A. 2015;3(15):8076–8082.
- Azeez F, Al-Hetlani E, Arafa M, et al., The effect of surface charge on photocatalytic degradation of methylene blue dye using chargeable titania nanoparticles. Scientific reports, 2018. 8.
- Al-Attafi K. Optimisation of TiO2 and Zn2SnO4 photoanode materials for efficient dye-sensitized solar cells. Australia: University of Wollongong; 2019.
- Song L, Du P, Shao X, et al. Effects of hydrochloric acid treatment of TiO2 nanoparticles/nanofibers bilayer film on the photovoltaic properties of dye-sensitized solar cells. Mater Res Bull. 2013;48(3):978–982.
- Jeong H, Lee Y, Kim Y, et al. Enhanced photoelectric efficiency by surface modification of TiO2 thin film using various acidic species. Korean J Chem Eng. 2010;27(5):1462–1468.
- Liu G, Yang HG, Pan J, et al. Titanium dioxide crystals with tailored facets. Chem Rev. 2014;114(19):9559–9612.
- Lin J, Zhao L, Heo Y-U, et al. Mesoporous anatase single crystals for efficient Co(2+/3+)-based dye-sensitized solar cells. Nano Energy. 2015;11:557–567.
- Lin J, Heo Y-U, Nattestad A, et al. 3D hierarchical rutile TiO2 and metal-free organic sensitizer producing dye-sensitized solar cells 8.6% conversion efficiency. Sci Rep. 2014;4:5769.
- Chen FC, Tanaka Y, Yang X. Photocatalytic and dye-sensitized solar cell performances of {010}-faceted and [111]-faceted anatase TiO2 nanocrystals synthesized from tetratitanate nanoribbons. ACS Appl Mater Interfaces. 2014;6(18):16007–16019.
- Wu B, Guo C, Zheng N, et al. Nonaqueous production of nanostructured anatase with high-energy facets. J Am Chem Soc. 2008;130(51):17563–17567.
- Yu J, Fan J, Lv K. Anatase TiO2 nanosheets with exposed (001) facets: improved photoelectric conversion efficiency in dye-sensitized solar cells. Nanoscale. 2010;2(10):2144–2149.
- Chu L, Qin Z, Yang J. Anatase TiO2 nanoparticles with exposed {001} facets for efficient dye-sensitized solar cells. Sci Rep. 2015;5:12143.
- Peng J-D, Lin -H-H, Lee C-T, et al. Hierarchically assembled microspheres consisting of nanosheets of highly exposed (001)-facets TiO2 for dye-sensitized solar cells. RSC Adv. 2016;6(17):14178–14191.
- Maitani MM, Tanaka K, Shen Q, et al. Electron transport properties in dye-sensitized solar cells with {001} facet-dominant TiO2 nanoparticles. Phys Chem Chem Phys. 2017;19(33):22129–22140.
- Bullard JW, Cima MJ. Orientation dependence of the isoelectric point of TiO2 (rutile) surfaces. Langmuir. 2006;22(24):10264–10271.
- Hiemstra T, Venema P, Riemsdijk WHV. Intrinsic proton affinity of reactive surface groups of metal (hydr)oxides: the bond valence principle. J Colloid Interface Sci. 1996;184(2):680–692.
- Griffiths DM, Rochester CH. Infrared study of the adsorption of water on to the surface of rutile. JChemi Soc Faraday Trans 1. 1977;73:1510–1529.
- Schaub R, Thostrup P, Lopez N, et al. Oxygen vacancies as active sites for water dissociation on rutile. Phys Rev Lett. 2001;87(26):266104.
- Kuang D-B, Uchida S, Humphry-Baker R, et al. Organic dye-sensitized ionic liquid based solar cells: remarkable enhancement in performance through molecular design of indoline sensitizers. Synthetic Metal. 2008;47:1923–1927.
- Li J, Kong F, Wu G, et al. Di-n-alkylphosphinic acids as coadsorbents for metal-free organic dye-sensitized solar cells. Synth Met. 2014;197:188–193.
- Selopal GS, Wu H-P, Lu J, et al. Metal-free organic dyes for TiO2 and ZnO dye-sensitized solar cells. Cell. 2016;6:18756.
- Dozzi M, Selli E. Specific facets-dominated anatase TiO2: fluorine-mediated synthesis and photoactivity. Catalysts. 2013;3(2):455–485.
- Sandhu S, Shahi SK, Singh V. Acidic ionic liquids: an alternative to HF for {001} reactive facet controlled synthesis of anatase titania. New J Chem. 2018;42(15):12762–12765.
- Cheng X-L, Hu M, Huang R, et al. HF-free synthesis of anatase TiO2 nanosheets with largely exposed and clean {001} facets and their enhanced rate performance as anodes of lithium-ion battery. ACS Appl Mater Interfaces. 2014;6(21):19176–19183.
- Al-Attafi K, Nattestad A, Yamauchi Y, et al. Aggregated mesoporous nanoparticles for high surface area light scattering layer TiO2 photoanodes in Dye-sensitized Solar Cells. Sci Rep. 2017;7(1):10341.
- Na J, Kim J, Park C, et al. TiO2 nanoparticulate-wire hybrids for highly efficient solid-state dye-sensitized solar cells using SSP-PEDOTs. RSC Adv. 2014;4(84):44555–44562.
- Lin J, Nattestad A, Yu H, et al. Highly connected hierarchical textured TiO2 spheres as photoanodes for dye-sensitized solar cells. ?J Mater Chem A. 2014;2(23):8902–8909.
- Zhu J, Wang S, Bian Z, et al. Solvothermally controllable synthesis of anatase TiO2 nanocrystals with dominant {001} facets and enhanced photocatalytic activity. CrystEngComm. 2010;12(7):2219–2224.
- Li J, Xu D. Tetragonal faceted-nanorods of anatase TiO2 single crystals with a large percentage of active {100} facets. Chem Comm. 2010;46(13):2301–2303.
- Kern A, Madsen IC, Scarlett NVY. Quantifying amorphous phases. Dordrecht: Springer Netherlands; 2012.
- Zhao P, Lu L, Liu X, et al. Error analysis and correction for quantitative phase analysis based on rietveld-internal standard method: whether the minor phases can be ignored? Crystals. 2018;8(3):110.
- Al-Attafi K, Nattestad A, Wu Q, et al. The effect of amorphous TiO2 in P25 on dye-sensitized solar cell performance. Chem Comm. 2018;54(4):381–384.
- Chou TP, Zhang Q, Russo B, et al. Titania particle size effect on the overall performance of dye-sensitized solar cells. J Phys Chem C. 2007;111(17):6296–6302.
- Bai Y, Mora-Seró I, De Angelis F, et al. Titanium dioxide nanomaterials for photovoltaic applications. Chem Rev. 2014;114(19):10095–10130.
- Kim B, Park SW, Kim J-Y, et al. Rapid dye adsorption via surface modification of TiO2 photoanodes for dye-sensitized solar cells. ACS Appl Mater Interfaces. 2013;5(11):5201–5207.
- Pazoki M, Lohse PW, Taghavinia N, et al. The effect of dye coverage on the performance of dye-sensitized solar cells with a cobalt-based electrolyte. Phys Chem Chem Phys. 2014;16(18):8503–8508.
- Zama I, Martelli C, Gorni G. Preparation of TiO2 paste starting from organic colloidal suspension for semi-transparent DSSC photo-anode application. Mater Sci Semicond Process. 2017;61:137–144.
- Parra R, Góes MS, Castro MS, et al. Reaction pathway to the synthesis of anatase via the chemical modification of titanium isopropoxide with acetic acid. Chem Mater. 2008;20(1):143–150.
- Qu Q, Geng H, Peng R, et al. Chemically binding carboxylic acids onto TiO2 nanoparticles with adjustable coverage by solvothermal strategy. Langmuir. 2010;26(12):9539–9546.
- Chellappa M, Anjaneyulu U, Manivasagam G, et al. Preparation and evaluation of the cytotoxic nature of TiO2 nanoparticles by direct contact method. Int J Nanomedicine. 2015;10(Suppl 1:31.
- Anselmi C, Mosconi E, Pastore M, et al. Adsorption of organic dyes on TiO2 surfaces in dye-sensitized solar cells: interplay of theory and experiment. Phys Chem Chem Phys. 2012;14(46):15963–15974.
- Chan M, Carrington T, Manzhos S. Anharmonic vibrations of the carboxyl group in acetic acid on TiO2: implications for adsorption mode assignment in dye-sensitized solar cells. Phys Chem Chem Phys. 2013;15(25):10028–10034.
- Bisquert J. Theory of the impedance of charge transfer via surface states in dye-sensitized solar cells. J Electroanal Chem. 2010;646(1–2):43–51.
- Bisquert J, Zaban A, Greenshtein M, et al. Determination of rate constants for charge transfer and the distribution of semiconductor and electrolyte electronic energy levels in dye-sensitized solar cells by open-circuit photovoltage decay method. J Am Chem Soc. 2004;126(41):13550–13559.
- Wang Q, Moser J-E, Grätzel M. Electrochemical impedance spectroscopic analysis of dye-sensitized solar cells. J Phys Chem A. 2005;109(31): 14945–14953.
- Kern R, Sastrawan R, Ferber J, et al. Modeling and interpretation of electrical impedance spectra of dye solar cells operated under open-circuit conditions. Electrochim Acta. 2002;47(26):4213–4225.
- Hu L, Dai S, Weng J, et al. Microstructure design of nanoporous TiO2 photoelectrodes for dye-sensitized solar cell modules. J Phys Chem A. 2007;111(2):358–362.
- Sánchez-García MA, Bokhimi X, Maldonado-Álvarez A, et al. Effect of anatase synthesis on the performance of dye-sensitized solar cells. Nanoscale Res Lett. 2015;10(1):306.
- Feng X, Zhu K, Frank AJ, et al. Rapid charge transport in dye-sensitized solar cells made from vertically aligned single-crystal rutile TiO2 nanowires. Angew Chem. 2012;124(11):2781–2784.