ABSTRACT
Phosphorus is a ubiquitous and one of the most common elements found in living organisms. Almost all molecules containing phosphorus in our body exist as analogs of phosphate salts or phosphoesters. Their functions are versatile and important, being responsible for forming the genetic code, cell membrane, and mineral components of hard tissue. Several materials inspired from these phosphorus-containing biomolecules have been recently developed. These materials have shown unique properties at the biointerface, such as nonfouling ability, blood compatibility, lubricity, mineralization induction capability, and bone affinity. Several unfavorable events occur at the interface of materials and living organisms because most of these materials have not been designed while taking host responses into account. These unfavorable events are directly linked to reducing functions and shorten the usable periods of medical devices. Biomimetic phosphorus-containing polymers can improve the reliability of materials in biological systems. In addition, phosphorus-containing biomimetic polymers are useful not only for improving the biocompatibility of material surfaces but also for adding new functions due to the flexibility in molecular design. In this review, we describe the recent advances in the control of biointerfacial phenomena with phosphorus-containing polymers. We especially focus on zwitterioninc phosphorylcholine polymers and polyphosphoesters.
Graphical abstract
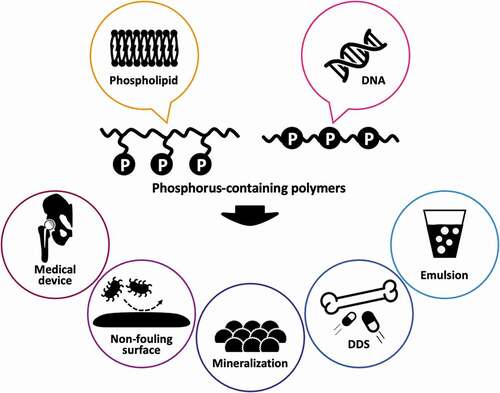
CLASSIFICATIONS:
1. Introduction
A biointerface is the interface between materials and living organisms or biological substances. Most reactions in biology are triggered at the interface when materials are in contact with biological environments. Therefore, controlling biointerfacial phenomena is important for the design of biomaterials. However, most materials used in medical and diagnostic applications are not selected with a consideration of the events occurring at the biointerface. Therefore, unfavorable host reactions with the biomaterials often occur, limiting the application of medical and diagnostic devices. One of the most typical biointerfacial events is biofouling. Protein adsorption is the first phenomenon when artificial materials come in contact with biological environments [Citation1]. The interactions recognized as occurring in protein adsorption are mostly noncovalent such as, H-bonding, electrostatic, and hydrophobic interactions [Citation2]. Therefore, the adsorption mode of proteins on a material surface is very complex phenomenon and affected by chemical and physical properties of the material surface. The adsorbed proteins subsequently trigger several host responses at the cellular level, such as thrombus formation, capsulation, inflammation, and infection [Citation3]. They also affect the physical and biochemical functions of medical and diagnostic devices. To improve the reliability of such devices, bioinertness, which can reduce nonspecific biofouling, is strongly required for the surfaces. In addition, biofunctional activity, which induces a specific biological reaction, sometimes becomes necessary, particularly in tissue engineering and bioadhesive applications. In other words, an appropriate surface function in a specific application is needed for materials.
Various surface modification processes of materials have been proposed physically and chemically [Citation4]. Biomimetic phosphorus-containing polymers are considered reliable candidates for improving the surface properties of materials [Citation5]. Phosphorus existing in the physiological environment forms analogs of phosphate salts or phosphoesters [Citation6]. Phosphorus-containing biomolecules have versatile and important functions, being responsible for forming the genetic code, cell membrane, and mineral components of hard tissue. Therefore, they are believed to have high potential for the development of biofunctional materials. In fact, zwitterionic phosphorylcholine (PC) polymers have been used to resolve the biointerfacial problems mentioned before and are also used in practical and clinical applications [Citation7]. In addition, pentavalent phosphorus of the phosphoester linkage allows versatile molecular design and immobilization [Citation8]. Thanks to the structural flexibility of these polymers, their alternative functions for biomedical applications have been actively studied [Citation5].
Reverse zwitterionic choline phosphate (CP)-substituted polymers [Citation9,Citation10] are interesting because they provide polymers bearing versatile functional zwitterions. In addition, polyphosphoesters (PPEs) are considered alternative biodegradable polymers [Citation11–13]. A certain kind of PPE shows similar properties as poly(ethylene glycol) (PEG) [Citation14]. The chemical structure and molecular weight of PPEs can be well tuned [Citation15,Citation16]. Therefore, the control of the physicochemical properties of PPEs and molecular conjugation are flexible. According to versatile molecular design capabilities, unique materials such as transdermal penetrating emulsions [Citation17] and bone-targeting macromolecular vehicles [Citation18] have been proposed.
Phosphorus-containing polymers are robust polymers with versatile functions of not only controlling biointerfacial phenomena but also generating alternative functional materials. In this review, we describe the recent advances in the control of biointerfacial phenomena with phosphorus-containing polymers. Typical phosphorus-containing polymers are surveyed, and their basic structure, properties, and advantageous aspects in the biomaterials field are introduced. In addition, the surface modification of materials with photoreactive zwitterionic PC polymers to reduce nonspecific biofouling is discussed.
2. Phosphorus-containing polymers
Phosphorus-containing polymers can be mainly divided into two groups (): polymers with phosphorus in their side chains and those with phosphorus in the main chain. Phospholipid-mimetic zwitterionic polymers are typical polymers in the former category. One of the most robust monomers in the biomedical field is 2-methacryloyloxyethyl phosphorylcholine (MPC). The synthesis route of highly purified MPC was established by Ishihara and coworkers in 1990 [Citation19], and numerous research publications on MPC polymers have been written since then. MPC polymers are applied in several biomedical devices such as soft contact lenses [Citation20], high-performance coronary stents [Citation21], artificial heart [Citation22], artificial joints [Citation23,Citation24], and biosensors [Citation25,Citation26] to improve their biointerfacial properties. The reduction in nonspecific biofouling is the most attractive surface phenomenon of MPC polymers. Some recent examples are shown in the next section.
Several MPC analogs with varying chain lengths and structures between PC and polymerizable groups have been synthesized [Citation27,Citation28]. The amphiphilic nature [Citation29] and mobility [Citation30] of the polymer chains could be controlled by a change in the structure of the spacers. In addition, CP-based polymers as reverse PC zwitterions have been recently designed to obtain polymers with versatile functional zwitterions. The CP monomer can be synthesized by the reaction of 2-dimethylaminoehyl methacrylate (DMAEMA) with cyclic phosphates having various functional groups. The functional CP polymers are converted to polymer prodrugs and hydrogels through photo- or click reactions [Citation10]. Recently, Emrick and coworkers reported that a competitive reaction occurs with 2-methoxy-2-oxo-1,3,2-dioxaphosphorane as a cyclic phosphate and a phosphate salt is easily formed with a zwitterionic CP compound [Citation9]. They successfully inhibited salt formation by using a cyclic phosphate with a butoxy group and clarified that the CP polymer resembles an MPC polymer with the absence of any significant cytotoxicity, hemolytic activity, red blood cell aggregation, and immunogenicity, opening up opportunities for these polymers in biology. When an undesired phosphate salt is formed as a by-product of a CP monomer, separation with an anion-exchange resin is effective [Citation31].
As mentioned before, phosphatidylcholine-mimetic polymers show biological inertness. In contrast, new zwitterionic polymers that can regulate immune reaction have been identified. Translocation of phosphatidylserine to the exposed membrane surface is an early event in apoptosis. In addition, phosphatidylserine, an ‘eat me’ signal present in apoptotic cells, is effectively recognized by specific receptors on professional phagocytes, facilitating the clearance of apoptotic cells [Citation32]. Phosphatidylserine also downregulates pro-inflammatory cytokine production [Citation33]. Ebara and coworkers synthesized a methacrylate-bearing phosphoryl serine (MPS) and reported that the MPS polymer with MPC (P(MPS/MPC)) can suppress macrophage activation [Citation34]. Interestingly, the suppression effect of P(MPS/MPC) was more remarkable than that of MPS homopolymer because the polar group composition of P(MPS/MPC) might be similar to that of the apoptotic cell membrane. They also suggested a possible beneficial effect of polymeric nanoparticles bearing phosphatidylserine groups for anti-inflammatory therapies [Citation35].
Vinyl monomers bearing phosphoric and phosphonic acids are also essential in the biomedical field, especially for dental applications [Citation5,Citation36]. These monomers show high affinity for mineral components of teeth and have mild self-etching ability, which is required for dental adhesion. In addition, these monomers have a normally hydrophobic part, such as a long alkyl chain or a phenyl group, to represent their amphiphilic nature. The amphiphilicity encourages penetration of methyl methacrylate (MMA), which is a typical resin monomer used in dental adhesives, in the dental tissue [Citation37,Citation38]. Phosphoric and phosphonic acids containing polymers are also favorable for nucleation in biomineralization [Citation39]. To improve the compatibility of biomaterials with hard tissues, the surface modification of biomaterials with these polymers would be effective.
The second category of phosphorus-containing polymers () includes polymers with phosphorus in the main chain. Polyphosphazene is one of the most historical phosphorus-containing polymers and was originally synthesized by Stokes [Citation40,Citation41]. Polyphosphazene is known as inorganic rubber, which is a type of organic-inorganic hybrid high-molecular polymer whose main chain contains alternating single and double bonds of phosphorus and nitrogen and is endowed with excellent flame-retardant synergies. In recent years, polyphosphazene has attracted interest not only in the industrial field but also in the biomedical field, such as matrices for drug delivery [Citation42], tissue engineering [Citation43], and immune adjuvant [Citation44].
PPEs are also attracting interest as polymers that have phosphorus in the main chain. The chemical structure of PPEs resembles that of the nucleic acid backbone, and PPEs show biodegradability and biocompatibility. The phosphorus in PPEs can form three stable and divergent bonds, in addition to the P = O bond, which offers advantages over conventional biodegradable polymers such as aliphatic polyesters and polycarbonates and means that side-chain functionalization of PPEs is possible even if two bonds are used in the formation of the polymer backbone [Citation12]. In addition, end functionalization of PPEs is also easy by changing the initiator [Citation45] or by performing postpolymerization modification [Citation46]. Although there are several synthesis routes for PPEs [Citation47], ring-opening polymerization (ROP) is the most reliable route to obtain PPEs with an ideal structure. Previously, we established a synthesis route for PPEs by ROP with an organocatalyst [Citation15]. Due to mild reactivity, the catalyst can be easily handled compared with organometallic catalysts. In addition, ROP proceeds in a living manner, resulting in a very narrow molecular weight distribution. Clément et al. subsequently improved the polymerization condition by adding thiourea to the reaction system, allowing a narrow molecular weight distribution to be maintained with a high yield (>60%) [Citation16]. Today, most studies use organocatalysts for the synthesis of PPEs by ROP.
Polyphosphonates have a similar structure as PPEs, and the alkyl-phosphonic acid linkage of phosphonate makes it more stable than the corresponding phosphoester with three ester groups [Citation48]. Wurm and coworkers synthesized polyphosphonates from phostones in a single step without toxic reagents [Citation49]. The phosphoester linkage of PPEs and polyphosphonates is easily broken under basic conditions [Citation11,Citation49,Citation50] but is relatively stable under physiological and acidic conditions. To prepare acid-labile phosphorus-containing polymers, polyphosphoramidates were developed. Cleavage of phosphoramidate bonds in the side chains of polyphosphoramidates in an acidic environment generates phosphodiester PPEs [Citation51]. In contrast, polyphosphoramidates that can be quickly degraded under acidic conditions are obtained by inserting a phosphoramidate linkage in the polymer backbone [Citation52].
Poly(phosphate) (polyP) is the only physiologically observed phosphorus-containing polymer discussed in this review and plays diverse roles in nature. PolyP can be obtained from not only a biological process but also chemical synthesis [Citation53]. Müller et al. reported that polyP has the potential to encourage bone regeneration [Citation54], and Wang et al. reported that polyP can be a metabolic fuel for bone mineralization [Citation55]. As polyP is physiologically synthesized in bone-forming osteoblast cells and blood platelets, it strongly affects the coagulation pathway and enhances fibrin formation [Citation56]. Although it is useful for bone tissue regeneration, polyP may not be suitable for injection into blood circulation.
3. Antibiofouling and antimicrobial properties
Biofouling is the most considerable event when biomaterials come in contact with physiological environments, because it causes undesired host reactions such as thrombus formation, inflammation, and infection. These reactions normally occur due to nonspecific protein adsorption. Therefore, the design of biomaterial surfaces that can control protein adsorption is essential in order to obtain reliable biomaterials. Zwitterionic MPC polymers are most effective for satisfying this demand. Preservation of the high content of free water in the hydrated polymer chains and zero zeta potential of the polar unit are considered dominant factors for nonfouling behavior of MPC polymers [Citation57]. MPC polymers are used in cardiovascular and orthopedic medical devices to solve biointerfacial problems [Citation7]. Various surface modification techniques are proposed for MPC polymers. Recently, surface modification with photoreactive MPC polymers has become an attractive trend because of universalness, control of the reaction area, reaction at low temperature, and low cost. shows several photoreactive MPC polymers that are used to generate nonfouling surfaces. Methacrylate-bearing phenyl azide ()) and benzophenone are suitable candidates as comonomers for the preparation of photoreactive polymers. Ishihara and coworkers synthesized MPC polymers with 2-methacryloyloxyethyl-4-azidobenzoate (MPAz) by conventional radical polymerization. The polymers were modified by photoreaction on the surface of various polymer base materials, such as polyethylene and polystyrene, and alkylated glass and titanium. The surface wettability after modification significantly improved in all MPC polymers. In particular, in the case of a binary copolymer, an MPC polymer containing about 20%–30% of MPAz unit was effective for surface modification. By addition of n-butyl methacrylate (BMA) unit as the third comonomer, the efficacy of the MPAz unit improved. The nonfouling ability of P(MPC/MPAz) (MPC:MPAz = 70:30 [mol%]) and cell adhesion was effectively inhibited on the surface modified with the polymer ()) [Citation58]. A similar trend was observed after using MPC polymers having benzophenone moieties.
Figure 2. (a) Chemical structure of P(MPC/BMA/MPAz). (b) Cell adhesion on pattern-modified polyethylene with P(MPC/MPAz). Reprinted with permission from [Citation58]. Copyright (2013) American Chemical Society. MPC, 2-methacryloyloxyethyl phosphorylcholine; BMA, n-butyl methacrylate; MPAz, 2-methacryloyloxyethyl-4-azidobenzoate
![Figure 2. (a) Chemical structure of P(MPC/BMA/MPAz). (b) Cell adhesion on pattern-modified polyethylene with P(MPC/MPAz). Reprinted with permission from [Citation58]. Copyright (2013) American Chemical Society. MPC, 2-methacryloyloxyethyl phosphorylcholine; BMA, n-butyl methacrylate; MPAz, 2-methacryloyloxyethyl-4-azidobenzoate](/cms/asset/c6968ddf-fc1f-45cd-b323-5a373a132e82/tsta_a_1908095_f0002_oc.jpg)
Tyrosine is another candidate with a photoreactive moiety. MPC was copolymerized with N-methacryloyl-(l)-tyrosinemethylester (MAT) (P(MPC/MAT) ()) and spin-coated on silicon (Si) wafers [Citation59]. Photo-assisted surface modification of Si wafers with MPC polymers was performed and affected not only polymer adhesion to a solid surface but also protein conjugation. The conjugation of regiospecific protein immobilization could be achieved through the use of a photomask, while nonspecific protein adsorption was reduced on non-irradiated regions. In addition, mammalian cell adhesion was regulated by the types of proteins coated on the P(MPC/MAT) surface [Citation60].
Figure 3. Surface modification of a Si wafer with photoreactive PC polymers. (a) Chemical structure of P(MPC/BMA/MAT). (b) Schematic of the visible light–assisted surface modification process. (c) Schematic of the visible light–assisted multiprotein patterning process. (d) Fluorescence micrographs of an Rh-BSA- and FITC-BSA-patterned surface. Reproduced with permission from [Citation61]. Copyright (2020) The Royal Society of Chemistry. MPC, 2-methacryloyloxyethyl phosphorylcholine; BMA, n-butyl methacrylate; MAT, N-methacryloyl-(L)-tyrosinemethylester; PC, phosphorylcholine; Rh-BSA, rhodamine-labeled bovine serum albumin; FITC, fluorescein isothiocyanate
![Figure 3. Surface modification of a Si wafer with photoreactive PC polymers. (a) Chemical structure of P(MPC/BMA/MAT). (b) Schematic of the visible light–assisted surface modification process. (c) Schematic of the visible light–assisted multiprotein patterning process. (d) Fluorescence micrographs of an Rh-BSA- and FITC-BSA-patterned surface. Reproduced with permission from [Citation61]. Copyright (2020) The Royal Society of Chemistry. MPC, 2-methacryloyloxyethyl phosphorylcholine; BMA, n-butyl methacrylate; MAT, N-methacryloyl-(L)-tyrosinemethylester; PC, phosphorylcholine; Rh-BSA, rhodamine-labeled bovine serum albumin; FITC, fluorescein isothiocyanate](/cms/asset/5044236a-f7a8-41d5-a871-6e2e3baaa9d7/tsta_a_1908095_f0003_oc.jpg)
For all these surface modifications, ultraviolet (UV) light was used. Recently, however, surface modification with P(MPC/MAT) using visible light has also been reported [Citation61]. For this technique, a ruthenium complex (Ru(II)bpy32+) and ammonium persulfate (APS) were used as a catalyst. A schematic of the visible light–assisted surface modification is shown in ). Two MPC polymers, namely P(MPC/MAT) (MPC:MAT = 70:30 [mol%]) and P(MPC/BMA/MAT) (MPC:BMA:MAT = 30:40:30 [mol%]), were used. P(MPC/BMA/MAT) is insoluble in water and so was used to form the base layer. A P(MPC/BMA/MAT) ethanol solution was spin-coated onto a Si wafer, with the thickness of the resultant P(MPC/BMA/MAT) layer being ~15 nm, which was determined using ellipsometry. A P(MPC/MAT) aqueous solution containing appropriate concentrations of Ru(II)bpy32+ and APS was subsequently spin-coated on the P(MPC/BMA/MAT)-coated Si wafer. Visible light was irradiated on the surface for 15 s. Finally, the surface was rinsed with distilled water. ) shows a schematic of multiprotein patterning on a solid surface. On a P(MPC/BMA/MAT) base layer, P(MPC/MAT) in a phosphate-buffered saline (PBS) solution containing rhodamine-labeled bovine serum albumin (Rh-BSA), Ru(II)bpy32+, and APS was spin-coated. Visible light was irradiated through a transmission electron microscopy (TEM) mesh for 15 s. To remove unreacted substances, the surface was rinsed with PBS. Next, a P(MPC/MAT) solution containing fluorescein isothiocyanate (FITC)-labeled BSA (FITC-BSA), rather than Rh-BSA, was spin-coated on the surface, and the surface was again exposed to visible light through the TEM mesh for 15 s. Then surface was then rinsed with PBS and water and observed under a fluorescence microscope ()). A well-defined feature of multiprotein immobilization was observed. The protein immobilization in P(MPC/MAT) layer would be performed through the dimerization of tyrosine residues between P(MPC/MAT) and the protein. The Because of the lowermost P(MPC/BMA/MAT) layer, nonspecific protein immobilization was completely reduced on the non-irradiated regions of the surface. Surface modification with an antibody instead of albumin was also demonstrated. In addition, antibody-immobilized surfaces can be applicable for immunoassay platforms. Streptavidin immobilized in the P(MPC/MAT) layer well captured biotinylated primary antibody.
The blood compatibility of MPC polymers is one of their attractive surface properties [Citation7,Citation62]. The mechanism for nonthrombogenicity of MPC polymers was well characterized with attention to the interaction between the polymers and blood components, including blood cells [Citation63,Citation64], proteins [Citation57,Citation65], and water [Citation57]. The concrete applications of MPC polymers for cardiovascular devices are well summarized elsewhere [Citation7,Citation62,Citation66]. The antimicrobial adhesive property of MPC polymers is also important for the surface modification of biomedical materials. Long-term use of biomaterials in the human body is threatened by the adhesion and proliferation of bacteria on the implant substrate, which secrete their own matrix on the architectural structure of the film hosted by multiple types of microorganisms. The primary biofilms are problematic because the associated fungal and bacterial cells evolve rapidly and become significantly more resistant to antimicrobial treatment. Therefore, the biofilms give rise to a wide range of bacterial infections on human tissue (lungs, wounds, or the middle ear) and those related to medical devices (vascular catheters, orthopedic prostheses, or dental carries), which are difficult to eradicate and negatively affect the patient’s quality of life and mortality rate [Citation67,Citation68].
Here, recent research reports on antimicrobial adhesion for dental applications are introduced. The development of an MPC-based polymer containing Ca2+-binding moieties for stronger binding with hydroxyapatite (HA) of the tooth surface has been reported [Citation69], and 2-methacryloyloxyethylphosphate (MOEP) was copolymerized with MPC (PMP) by free-radical polymerization. The authors found that HA surface-coated with PMP containing around 50% MPC unit showed the best performance in preventing protein adsorption and downstream cell and bacterial adhesion ()). Susita et al. [Citation70] first synthesized PPEs with pendant PC moieties via thio-yne click chemistry of thiol-terminated PC with a phosphodiester PPE polymer (PEP-PC) ()). PEP-PC showed high affinity for the HA surface, preventing acid erosion of the HA at pH 5. In addition, PEP-PC shows efficient performance in preventing the adhesion of Streptococccus mutans and inhibiting biofilm formation on the HA surface. We believe PEP-PC can be used in dental applications. Thongthai et al. [Citation71] synthesized MPC polymers with 12-methacryloyloxydodecylpyridinium bromide (MDPB) and BMA and coated them on the surface of a resin for controlling bacterial growth in the oral environment (). This coating provided a more hydrophilic surface than that coated by MDPB and reduced the adsorption of BSA and salivary protein. In addition, a sparse biofilm and dead bacteria in the biofilm on the surface coated with P(MPC/BMA/MDPB) (MPC:BMA:MDPB = 15:70:15 [mol%]) were also observed after 48 h incubation in a S. mutans suspension ()).
Figure 4. (a) MPC-based polymer coating on HA disks to prevent the attachment of proteins and oral bacteria and SEM images of noncoated, PMP50-coated, and PMPC-coated surfaces of HA disks. Reprinted with permission from [Citation69]. Copyright (2016) Elsevier. (b) Bacterial adhesion effect of HA surface with and without immobilization of zwitterionic PEP: the proposed mechanism of PEP-PC. The CLSM biofilm structure in top and side views of Streptococcus mutans on HA and PEP-PC-immobilized HA (scale bar = 10 µm). Reprinted with permission from [Citation70]. Copyright (2019) CSJ. MPC, 2-methacryloyloxyethyl phosphorylcholine; HA, hydroxyapatite; PEP-PC, phosphorylcholine with a polyphosphoester copolymer; SEM, scanning electron microscopy; CLSM, confocal laser scanning microscopy
![Figure 4. (a) MPC-based polymer coating on HA disks to prevent the attachment of proteins and oral bacteria and SEM images of noncoated, PMP50-coated, and PMPC-coated surfaces of HA disks. Reprinted with permission from [Citation69]. Copyright (2016) Elsevier. (b) Bacterial adhesion effect of HA surface with and without immobilization of zwitterionic PEP: the proposed mechanism of PEP-PC. The CLSM biofilm structure in top and side views of Streptococcus mutans on HA and PEP-PC-immobilized HA (scale bar = 10 µm). Reprinted with permission from [Citation70]. Copyright (2019) CSJ. MPC, 2-methacryloyloxyethyl phosphorylcholine; HA, hydroxyapatite; PEP-PC, phosphorylcholine with a polyphosphoester copolymer; SEM, scanning electron microscopy; CLSM, confocal laser scanning microscopy](/cms/asset/4fc9ccd3-187a-487b-83d0-5697880e49b4/tsta_a_1908095_f0004_oc.jpg)
Figure 5. (a) Chemical structure of P(MPC/BMA/MDPB). (b) Dual functions of protein repellent property and antibacterial effect of MDPB/MPC polymer against Streptococcus mutans and effective inhibition of biofilm formation. (c) CLSM of S. mutans biofilm formed after 48 h incubation on the surface of the coated specimen. The biofilm was stained with LIVE/DEAD® BacLight bacterial viability kits. The percentage of live and dead bacteria is shown under the images. (i) Control, (ii) MPC/BMA, (iii) MDPB/MPC/BMA, and (iv) MDPB/BMA. Scale bars = 30 μm. Reprinted with permission from [Citation71]. Copyright (2020) Wiley. MPC, 2-methacryloyloxyethyl phosphorylcholine; BMA, n-butyl methacrylate; MDPB, 12-methacryloyloxydodecylpyridinium bromide; CLSM, confocal laser scanning microscopy
![Figure 5. (a) Chemical structure of P(MPC/BMA/MDPB). (b) Dual functions of protein repellent property and antibacterial effect of MDPB/MPC polymer against Streptococcus mutans and effective inhibition of biofilm formation. (c) CLSM of S. mutans biofilm formed after 48 h incubation on the surface of the coated specimen. The biofilm was stained with LIVE/DEAD® BacLight bacterial viability kits. The percentage of live and dead bacteria is shown under the images. (i) Control, (ii) MPC/BMA, (iii) MDPB/MPC/BMA, and (iv) MDPB/BMA. Scale bars = 30 μm. Reprinted with permission from [Citation71]. Copyright (2020) Wiley. MPC, 2-methacryloyloxyethyl phosphorylcholine; BMA, n-butyl methacrylate; MDPB, 12-methacryloyloxydodecylpyridinium bromide; CLSM, confocal laser scanning microscopy](/cms/asset/2ae3ae01-b6e9-4be0-ba6d-64233d11fc88/tsta_a_1908095_f0005_oc.jpg)
4. Lubrication
In addition to the antifouling property, lubricity is another interesting property of surfaces modified with zwitterionic MPC polymers [Citation72]. To achieve super-low-friction phenomena, comb-like graft chains of the MPC homopolymer (P(MPC)) should be formed on the surface [Citation73,Citation74]. Therefore, surface-initiated graft polymerization of MPC is the most effective way for surface modification [Citation75]. Moro et al. and Ishihara et al. were pioneers of improving the lubricity of the surface of cross-linked ultra-high-molecular weight polyethylene (CLPE) to prolong the lifetime of the artificial hip joint [Citation23,Citation76]. For graft polymerization, the photo-initiator benzophenone (BP) was coated on CLPE from the acetone solution. BP-coated CLPE was soaked in an aqueous solution containing MPC. Finally, graft polymerization of MPC was performed under UV (≈350 nm) photo-irradiation. Surface modification with P(MPC) significantly reduced the surface friction of CLPE. Lubrication of the modified surface was due to hydration lubrication [Citation77]. A P(MPC) graft layer of ~100 nm thickness accumulated water molecules, which play an important role in lubrication. Even under load, the water molecules might be preserved in the polymer layer and superior lubrication could be observed. The molecular motion of P(MPC) chains is also an important factor in high lubricity. Surface-initiated graft polymerization is a suitable way to generate a polymer brush structure having flexible free chain ends. Currently, an innovative hip joint system with a P(MPC)-grafted CLPE liner, Aquala® (Kyocera Co, Kyoto, Japan), was used in Japan; the hip joint is expected to last longer than conventional artificial hip joints [Citation24].
Poly(ether ether ketone) (PEEK) is gaining attention as an orthopedic implant material because of suitable mechanical properties compared with other metals [Citation78,Citation79]. Although the bulk properties of PEEK are sufficient for medical application, its surface property is not because PEEK has not been designed considering biocompatibility [Citation80]. Furthermore, high surface friction is one of the limitations of PEEK and may induce wear, device lifetime shortening, and tissue irritation. Nonspecific biofouling phenomena on neat PEEK should be diminished because they induce unfavorable bioreactions and infections at the surface of PEEK-based implants. To improve the interfacial properties of PEEK, surface modification with P(MPC) has been studied. Due to the structure of the PEEK backbone, which contains an alkyl ketone (a benzophenone unit), self-initiated graft polymerization of an acrylic (methacrylic) monomer can be performed without any photo-initiator. Kyomoto et al. first reported the surface modification of PEEK with MPC via self-initiated graft polymerization without any additional photo-initiator [Citation81]. PEEK specimens were soaked in an aqueous solution containing MPC and irradiated with UV light for appropriate periods. This method is simple, clean, and reliable. Surface properties, such as lubricity and antifouling, significantly improved. Recently, Nakano et al. successfully improved the wear resistance of P(MPC) brush layers generated on PEEK [Citation82]. A photoreactive zwitterionic monomer, 2-[2-(methacryloyloxy)ethyldimethylanmmonium]ethyl benzophenoxy phosphate (MBPP; )), was newly synthesized. MBPP is water soluble even though it has a hydrophobic BP unit. By adding only 0.5–0.75 mol% of MBPP to an MPC aqueous solution for polymerization, the stability of polymer brush layers formed on the PEEK surface significantly improved ()). As mentioned before, the superior lubrication of the P(MPC) brush layer is due to hydration of the polymer chains. Therefore, the dehydration of P(MPC) brushes under compression was monitored by Fourier transform infrared spectroscopy with attenuated total reflection. Addition of a small amount of MBPP and the formation of a cross-linked structure in polymer brushes effectively preserved the hydration state under compressive loading.
Figure 6. (a) Chemical structure of MBPP and (b) continuous friction test for PEEK and that grafted with P(MPC) with or without MBPP. Reprinted with permission from [Citation82]. Copyright (2019) American Chemical Society. MBPP, 2-[2(methacryloyloxy)ethyldimethylammonium]ethyl benzophenoxy phosphate; PEEK, poly(ether ether ketone)
![Figure 6. (a) Chemical structure of MBPP and (b) continuous friction test for PEEK and that grafted with P(MPC) with or without MBPP. Reprinted with permission from [Citation82]. Copyright (2019) American Chemical Society. MBPP, 2-[2(methacryloyloxy)ethyldimethylammonium]ethyl benzophenoxy phosphate; PEEK, poly(ether ether ketone)](/cms/asset/0daba9e1-7387-4e83-a0d1-3240b45c1064/tsta_a_1908095_f0006_oc.jpg)
5. Mineralization
Mineralization is one of the promising ways to improve the hard-tissue compatibility of materials. Several surface modification processes have been proposed for polymer and metal implants [Citation83–85]. The introduction of anionic groups on material surfaces is an attractive approach to inducing mineralization due to the affinity to calcium ions dissolved in media. Especially, phosphorus-containing polymers are recognized to be a trigger of nucleation of calcium salts, such as calcium phosphates and calcium carbonates.
Wentrup-Byrne and coworkers immobilized an MOEP polymer (P[MEOP]) on the surface of an expanded polytetrafluoroethylene (ePTFE) membrane for optimum mineralization [Citation86] and observed the induction of mineralization on the surface-modified ePTFE membrane. However, the characterization of P(MEOP) grafted was unclear. So they synthesized well-defined, non-crosslinked and crosslinked P(MOEP) via reversible addition-fragmentation chain transfer (RAFT)-mediated polymerization [Citation87] and demonstrated that the amount of phosphate groups and the degree of cross-linking, as well as accessibility of the phosphates themselves, play an important role in both the amount and the type of mineral formed. They also highlighted the importance of accessible ionic phosphate groups for calcium ion chelation and subsequent calcium phosphate nucleation.
Penczek et al. synthesized amphiphilic phosphorylated polyethylene and studied the effect of polymer additives on the crystallization of inorganic salts, such as the frequently studied CaCO3 and Ca3(PO4)2, crystallized in water solutions from the soluble salts, bearing Ca2+ cations and the corresponding soluble salts with required anions [Citation88]. They found that phosphorylated polyethylene attaches to inorganic salt crystals and forms a polymer–salt hybrid under appropriate conditions. They also used triblock copolymers consisting of PEG and poly(alkylene phosphate) to modify CaCO3 crystallization [Citation89]. The size and structure of crystals varied with a change in the concentration of the triblock copolymers. Moreover, unusual semi-open empty spheres were obtained when the crystallization was performed under specific conditions [Citation90]. These results indicate that phosphate groups of polymers intervene in the process of crystallization, and they have been summarized in several review articles [Citation91,Citation92].
PPEs also encourage mineralization on material surfaces. We synthesized phosphodiester macromonomers and immobilized them onto a PEEK surface [Citation93]. As mentioned before, PEEK has been gaining interest in the orthopedic field due to its similar mechanical properties as natural bone tissue. However, the surface of pristine PEEK does not harmonize with bone tissue, and surface modification is required to enhance compatibility. A PPE macromonomer was synthesized via ROP using N-(2-hydroxypropyl) methacrylamide (HPMA) as an initiator. Phosphodeister macromonomers (PEPMA·Na, )) are grafted on PEEK by photo-induced self-initiated graft polymerization without any photo-initiator. To enhance calcium phosphate nucleation and biomineralization on a PEEK surface, PEPMA·Na was synthesized and immobilized on a PEEK substrate via self-initiated surface graft polymerization. Mineralization on the PEEK-g-poly(PEPMA·Na) substrate in x1.5 simulated body fluid (SBF) [Citation94] was significant compared to that on a non-modified PEEK surface ()).
Figure 7. (a) Schematic of the surface modification of PEEK specimens and (b) SEM images of bare PEEK and PEEK-g-poly(PEPMA·Na) after soaking in x1.5 SBF for 28 days. Reprinted with permission from [Citation93]. Copyright (2019) Taylor & Francis. PEEK, poly(ether ether ketone); SEM, scanning electron microscopy; PEPMA·Na, phosphodeister macromonomers; SBF, simulated body fluid
![Figure 7. (a) Schematic of the surface modification of PEEK specimens and (b) SEM images of bare PEEK and PEEK-g-poly(PEPMA·Na) after soaking in x1.5 SBF for 28 days. Reprinted with permission from [Citation93]. Copyright (2019) Taylor & Francis. PEEK, poly(ether ether ketone); SEM, scanning electron microscopy; PEPMA·Na, phosphodeister macromonomers; SBF, simulated body fluid](/cms/asset/21e1361a-8cf6-4f44-9f09-e1dc945109ad/tsta_a_1908095_f0007_oc.jpg)
6. Bone targeting
Bone targeting is one of the unique aims for phosphorus-containing polymers. It is well known that polymeric prodrugs are effective for prolonging the blood circulation of drugs due to the excluded volume. By adding bone-targeting moieties into prodrugs, bone-specific drug delivery can be performed. Previous studies have reported immobilization of bisphosphonates into water-soluble polymers as an advantageous approach. Kopeček and coworkers prepared an HPMA polymer bearing bisphosphonates [Citation95]. Drugs and imaging probes could be easily incorporated into this polymer through simple chemical ligations [Citation96]. An HPMA polymer bearing FITC and TNP-470 decreased the growth and vascularity of primary tumors and metastases in several human tumor xenografts [Citation97]. The authors targeted both tumor and endothelial compartments of bone metastases and calcified neoplasms with a single administration. The HPMA polymer substantially inhibited osteosarcoma growth.
PEG is commonly used as a water-soluble polymer to design polymeric drug carriers. Satchi-Fainaro et al. synthesized heterobifunctionalized PEG with alendronate and paclitaxel (PTX) for targeted treatment of breast cancer bone metastases [Citation98]. The heterobifunctionalized PEG formed self-assembled micelles, and the elimination half-life was longer than that of free PTX. The intravenously injected micelles accumulated in tumors well and displayed substantial antitumor effects in both murine syngeneic and human xenogeneic mouse models of bone metastases of mammary carcinomas.
In contrast, studies have reported accelerated blood clearance for some water-soluble polymers, including PEG, and lower antigenicity of zwitterionic polymers [Citation99]. Therefore, a bone-targeting drug carrier was produced by introducing osteotropic bisphosphonate alendronate (ALN) units into an amphiphilic MPC polymer ()) [Citation100]. This polymer, P(MPC/BMA/N-methacryloylalendronate [NMA]) (MPC:BMA:NMA = 40:55:5 [mol%]), could well solubilize hydrophobic anticancer drugs such as PTX and docetaxel (DTX) as shown in ). The nanosized particles generated from the amphiphilic polymers with DTX showed anticancer activities against several breast cancer cell lines, and particle formation did not hamper the pharmacological effect of DTX. Fluorescence observations evaluated by an in vivo imaging system and fluorescence microscopy showed that the addition of ALN to the Rho-P(MPC/BMA/NMA) enhanced bone accumulation ()). Bone-targeting phospholipid polymers are potential solubilizing excipients used to formulate DTX and deliver the hydrophobic drug to bone tissues by blood administration.
Figure 8. (a) Chemical structure of P(MPC/BMA/NMA)-Rho. (b) Solubility curve of DTX in the P(MPC/BMA/NMA) solution. The picture of DTX placed (i) in PBS and (ii) in the P(MPC/BMA/NMA) solution. The concentrations of DTX and P(MPC/BMA/NMA) are 5 and 200 mg/ml, respectively (n = 3, mean ± SD). (c) The biodistribution of P(MPC/BMA)-Rho or P(MPC/BMA/NMA)-Rho 3 days after intravenous injection. A sagittal section of the proximal end of the tibia was observed using confocal microscopy. Nuclei (blue) and Rho-labeled polymer (red) were excited at 405 nm (emission collected from 417 to 477 nm) and at 561 nm (emission collected from 571 to 1,000 nm), respectively. Magnification ×20. Bar = 100 µm. Reprinted with permission from [Citation100]. Copyright (2020) Wiley. MPC, 2-methacryloyloxyethyl phosphorylcholine; BMA, n-butyl methacrylate; NMA, N-methacryloylalendronate; Rho, rhodamine; DTX, docetaxel
![Figure 8. (a) Chemical structure of P(MPC/BMA/NMA)-Rho. (b) Solubility curve of DTX in the P(MPC/BMA/NMA) solution. The picture of DTX placed (i) in PBS and (ii) in the P(MPC/BMA/NMA) solution. The concentrations of DTX and P(MPC/BMA/NMA) are 5 and 200 mg/ml, respectively (n = 3, mean ± SD). (c) The biodistribution of P(MPC/BMA)-Rho or P(MPC/BMA/NMA)-Rho 3 days after intravenous injection. A sagittal section of the proximal end of the tibia was observed using confocal microscopy. Nuclei (blue) and Rho-labeled polymer (red) were excited at 405 nm (emission collected from 417 to 477 nm) and at 561 nm (emission collected from 571 to 1,000 nm), respectively. Magnification ×20. Bar = 100 µm. Reprinted with permission from [Citation100]. Copyright (2020) Wiley. MPC, 2-methacryloyloxyethyl phosphorylcholine; BMA, n-butyl methacrylate; NMA, N-methacryloylalendronate; Rho, rhodamine; DTX, docetaxel](/cms/asset/e11b5e18-a75b-45b4-80c4-0eec6975bd15/tsta_a_1908095_f0008_oc.jpg)
Methacrylic and acrylic polymers allow conjugation of multiple components because of the variety of functional monomers established. However, the main chain of these polymers is nondegradable. To avoid accumulation of carrier materials at the target site, the molecular design of polymers having a degradable backbone might be required. As described before, a PPE with phosphodiester linkages in the polymer backbone shows high mineral affinity. Therefore, studies investigated bone affinity in vivo [Citation18,Citation101]. To track the biodistribution of phosphodiester PPE (PEP•Na) in vivo, fluorescence-labeled PEP•Na (Cy5-PEP•Na) was synthesized and Cy5-PEP•Na or Cy5-azide (Cy5-Az) was injected into the tail veins of Institute of Cancer Research (ICR) mice. Immediately after intravenous injection, strong fluorescence signals were observed from the whole body of each mouse, suggesting that both Cy5-PEP•Na and Cy5-Az rapidly spread throughout the body via the bloodstream. However, after 30 h, the total radiant efficiencies of the spines of Cy5-Az-treated mice returned to the same levels, almost extinguished, as before the injection, whereas fluorescence signals from the spines of Cy5-PEP•Na-treated mice were still observed 75 h after injection [Citation101]. Because the observations were made in the dorsal position, fluorescence signals from the bone located near the surface were significant. Recently, studies have reported that PEP•Na affects the differentiation of bone cells, such as osteoblastic [Citation102] and osteoclastic [Citation103] cells. Phosphodiester PPEs may have the potential to control bone remodeling and be an alternative material for macromolecular bone therapeutics.
7. Particle-stabilized emulsion for topical and transdermal drug delivery
Particle-stabilized emulsions (PEs) are surfactant-free emulsions stabilized with solid particles, which constitute an interesting strategy for the encapsulation and transport of drugs in pharmaceutical and cosmetic formulations [Citation104]. PEs show improved long-term stability, even without the addition of a conventional surfactant, are more environmentally friendly than other kinds of emulsions, and cause less toxicity to human bodies [Citation105,Citation106]. Such properties make PE systems promising candidates as drug carriers for controlled drug delivery [Citation107].
Solid emulsifiers decorated with different PPEs have been recently proposed as green particle emulsifiers to improve the properties of PE systems, including biocompatibility and biodegradability, which are often required in biomedical applications. For instance, Che et al. [Citation108] introduced a hydrophobic PPE containing an amino group at the end of the chain, poly[2-(but-3-yn-1-yloxy)-2-oxo-1,3,2-dioxaphospholane] (PBYP-NH2), onto the surface of cellulose nanocrystals (CNCs) via combining the 2,2,6,6-tetramethylpiperidine 1-oxyl (TEMPO) oxidation of CNCs, ROP of a BYP monomer, and amidation to improve the surface properties and amphipathic properties of CNCs and proposed using it as the sole particle stabilizer to prepare an oil-in-water (O/W) emulsion and to perform the emulsion polymerization of styrene. The styrene-in-water emulsion could be simply described as mixing the styrene monomer and a CNC-PBYP-COOH aqueous dispersion in a 30:70 (v/v) ratio. The PPE-modified CNCs (CNC-PBYP-COOH) had a higher ability to stabilize emulsions compared with pristine CNCs due to the introduction of hydrophobic PPE chains on the CNC surface. The formed emulsions were stable when the concentration of modified CNCs was >5.0 mg/mL, and the droplet sizes of the formed emulsions were ~500 nm. In addition, the emulsions could be polymerized to yield nanosized latexes and displayed good stability in the polymerization. Recently, we reported an O/W emulsion stabilized by CNCs modified with a thermoresponsive PPE, poly(2-isopropoxy-2-oxo-1,3,2-dioxaphospholane) (PIPP), to form (CNC-g-PIPP) via ROP to improve the emulsification efficacy ()). We found that CNC-g-PIPP displayed better surface activity and interfacial properties compared with pristine CNCs at 4°C and helped enhance emulsion stabilization by using a slight amount of grafted particles (~0.4 wt%). O/W emulsions stabilized by CNC-g-PIPP exhibit excellent stability against coalescence for at least 15 days. The emulsion stability could be easily controlled by changes in temperature due to the thermal-sensitive property of PIPP chains on CNCs surfaces ()). When the temperature was increased from 4°C to 45°C (i.e. above the lower critical solution temperature of the PIPP chain), complete phase separation of the emulsion occurred within 45 h. In addition, the emulsification/demulsification process was repeatable for several cycles, with only a slightly increased droplet size [Citation17]. Thus, these different kinds of PPE-modified CNCs can be used as green emulsifiers to form small-size emulsions, which can be applied in many fields, such as cosmetic products and drug delivery systems.
Figure 9. (a) Hypothesized schematic of possible mechanisms for the reversible emulsification/demulsification of CNC-g-PIPP-stabilized emulsion. (b) Creaming profiles for emulsion proportion of emulsion stabilized by 0.4 wt% of CNC-g-PIPP and CNCs at different temperatures. CNC, cellulose nanocrystal; PIPP, poly(2-isopropoxy-2-oxo-1,3,2-dioxaphospholane). Reprinted with permission from [Citation17]. Copyright (2019) American Chemical Society
![Figure 9. (a) Hypothesized schematic of possible mechanisms for the reversible emulsification/demulsification of CNC-g-PIPP-stabilized emulsion. (b) Creaming profiles for emulsion proportion of emulsion stabilized by 0.4 wt% of CNC-g-PIPP and CNCs at different temperatures. CNC, cellulose nanocrystal; PIPP, poly(2-isopropoxy-2-oxo-1,3,2-dioxaphospholane). Reprinted with permission from [Citation17]. Copyright (2019) American Chemical Society](/cms/asset/03e069d4-176e-4073-9692-235d2c786ac8/tsta_a_1908095_f0009_oc.jpg)
Most recently, we proposed a stable O/W emulsion loaded with bifonazole (BFZ) stabilized by CNC-g-PIPP (BFZ-loaded CPPE) as a platform for topical lipophilic drug delivery ()) [Citation109]. BFZ-loaded CPPE has an acceptable size and good interface properties, sustained drug release, and retention. It shows enhanced drug permeation and penetration compared with BFZ-loaded surfactant-stabilized emulsion and BFZ solution. Nile red (NR) fluorescence-labeling studies showed that NR/BFZ-loaded CPPE could well penetrate porcine skin layers ()). The ability of CPPE to enhance drug penetration was attributed to the excellent stability and amphiphilic property of CNC-g-PIPP particles, which are surrounded at an O/W interface. In addition, we also found that after 24 h, exposure of BFZ-loaded CPPE is likely to be tolerable in terms of histopathology because of the side-chain PPEs grafted on CNC surfaces ()), which are significantly biocompatible in vivo. These findings indicated that the proposed emulsion systems carrying PPE can be a preferred choice for improving topical drug delivery without causing skin irritation.
Figure 10. (a) Possible penetration of BFZ-loaded CPPE for topical delivery through the skin. (b) CLSM images revealing the penetration and distribution of Nile red within porcine skin after being exposed for 24 h to CPPE, CE, and SOL. Scale bar = 100 µm. (c) Histopathological analysis of skin structure after H&E staining of normal porcine skin, skin treated with BFZ-loaded CPPE, skin treated with BFZ-loaded CE, and skin treated with SOL (40× magnification). Scale bar = 20 μm. Reprinted with permission from [Citation109]. Copyright (2021) Elsevier. BFZ, bifonazole; CNC, cellulose nanocrystal; PIPP, poly(2-isopropoxy-2-oxo-1,3,2-dioxaphospholane); CPPE, CNC-g-PIPP particle-stabilized emulsion; CE, conventional surfactant-stabilized emulsion; SOL, drug-in-oil as the control solution; CLSM, confocal laser scanning microscopy; H&E, hematoxylin and eosin
![Figure 10. (a) Possible penetration of BFZ-loaded CPPE for topical delivery through the skin. (b) CLSM images revealing the penetration and distribution of Nile red within porcine skin after being exposed for 24 h to CPPE, CE, and SOL. Scale bar = 100 µm. (c) Histopathological analysis of skin structure after H&E staining of normal porcine skin, skin treated with BFZ-loaded CPPE, skin treated with BFZ-loaded CE, and skin treated with SOL (40× magnification). Scale bar = 20 μm. Reprinted with permission from [Citation109]. Copyright (2021) Elsevier. BFZ, bifonazole; CNC, cellulose nanocrystal; PIPP, poly(2-isopropoxy-2-oxo-1,3,2-dioxaphospholane); CPPE, CNC-g-PIPP particle-stabilized emulsion; CE, conventional surfactant-stabilized emulsion; SOL, drug-in-oil as the control solution; CLSM, confocal laser scanning microscopy; H&E, hematoxylin and eosin](/cms/asset/5516e914-831b-4c0c-aa16-745aa29ba407/tsta_a_1908095_f0010_oc.jpg)
8. Conclusions
We have reviewed recent advances in biointerfacial aspects of phosphorus-containing polymers. Biomimetic phosphorus-containing polymers can reduce nonspecific biofouling, which is the most serious issue occurring at the surface of biomaterials in biological environments. Biomimetic phosphorus-containing polymers can prolong implementation periods and enhance the reliability of biomedical devices. The flexibility in the molecular design of phosphorus-containing polymers is also advantageous compared with conventional biomedical polymers. Furthermore, phosphorus-containing polymers are useful to control specific biological interactions for diagnostics and targeted therapeutic applications in the biomedical field.
Acknowledgments
The authors would like to thank Dr. Akihisa Otaka for valuable discussion and technical support.
Disclosure statement
No potential conflict of interest was reported by the author(s).
Additional information
Funding
Notes on contributors
Suphatra Hiranphinyophat
Suphatra Hiranphinyophat was born in Thailand in 1992. She received her B.Eng., and M.Eng. degrees from Thammasat University, Thailand. Recently, she received her PhD in 2021 from Kansai University, Japan under the supervision of Prof. Yasuhiko Iwasaki. Her current interest is focused on biomaterials for medical technologies.
Yasuhiko Iwasaki
Yasuhiko Iwasaki was born in Tokyo, Japan in 1971. He obtained his MS degree in Department of Industrial Chemistry, Nihon University, Tokyo, Japan, in 1995. Since 1995, he joined the organic materials laboratory of Institute of Biomedical and Bioengineering, Tokyo Medical and Dental University (TMDU). He worked as assistant professor at TMDU for three years and he received his PhD from Nihon University in 1998. He was then promoted as an assistant professor of TMDU in 1998. At the TMDU, he studied about surface modification with zwitterionic phosphophoryl choline polymers to produce blood compatible biomedical devices. In 2000, he stayed at Polymer Science and Engineering Department (PS&E), University of Massachusetts (UMASS) for 7 months as a visiting scientist. At UMASS, he studied the modification of solid surfaces with organosilane compounds. In 2007, he moved to Department of Chemistry and Materials Engineering, Faculty of Chemistry, Materials and Bioengineering, Kansai University, Osaka, Japan Kansai University and he was promoted to the full professor in 2011. His present research interests are surface modification of polymeric biomaterials, blood compatible surface, biodegradable polyphosphoesters and surface engineering of living mammalian cells. Dr. Iwasaki received several awards related biomaterials and polymer science such as, Award for Encourage of Research in Polymer Science in 2005, Award of Japanese Society for Biomaterials in 2020, Fellows of Biomaterials Science and Engineering in 2020 etc.
References
- Horbett TA, Latour RA. Adsorbed proteins on biomaterials. In: Wagner WR, Sakiyama-Elbert SE, Zhang G, et al. editors. Biomaterials science. 4th ed. Cambridge:Academic Press; 2020. p.645–660.
- Horbett TA, Brash JL, editors. Proteins at interfaces II: fundamentals and applications. ACS symposium series 602. Washington DC: American Chemical Society; 1995.
- Szott LM, Horbett TA. Protein interactions with surfaces: cellular responses, complement activation, and newer methods. Curr Opin Chem Biol. 2011;15:677–682.
- Ratner BD, Hoffman AS, McArthur SL. Physicochemical surface modification of materials used in medicine. In: Wagner WR, Sakiyama-Elbert SE, Zhang G, et al. editors. Biomaterials science. 4th ed. Cambridge:Academic Press; 2020. p.487–505.
- Monge S, Canniccioni B, Graillot A, et al. Phosphorus-containing polymers: a great opportunity for the biomedical field. Biomacromolecules. 2011;12:1973–1982.
- Walsh CT. Chapter 1, Introduction to phosphorus chemical biology. In: Walsh CT, editor. The chemical biology of phosphorus. Cambridge: Royal Society of Chemistry; 2021. p. 3–26.
- Ishihara K. Revolutionary advances in 2-methacryloyloxyethyl phosphorylcholine polymers as biomaterials. J Biomed Mater Res A. 2019;107:933–943.
- Bauer KN, Tee HT, Velencoso MM, et al. Main-chain poly(phosphoester)s: history, syntheses, degradation, bio-and flame-retardant applications. Prog Polym Sci. 2017;73:61–122.
- Hu G, Parelkar SS, Emrick T. A facile approach to hydrophilic, reverse zwitterionic, choline phosphate polymers. Polym Chem. 2015;6:525–530.
- Hu G, Emrick T. Functional choline phosphate polymers. J Am Chem Soc. 2016;138:1828–1831.
- Baran J, Penczek S. Hydrolysis of polyesters of phosphoric acid. 1. Kinetics and the pH profile. Macromolecules 1995;28:5167–5176.
- Yilmaz ZE, Jérôme C. Polyphosphoesters: new trends in synthesis and drug delivery applications. Macromol Biosci. 2016;16:1745–1761.
- Bauer KN, Liu L, Wagner M, et al. Mechanistic study on the hydrolytic degradation of polyphosphates. Eur Polym J. 2018;108:286–294.
- Pelosi C, Tinè MR, Wurm FR. Main-chain water-soluble polyphosphoesters: multi-functional polymers as degradable PEG-alternatives for biomedical applications. Eur Polym J. 2020;141:110079.
- Iwasaki Y, Yamaguchi E. Synthesis of well-defined thermoresponsive polyphosphoester macroinitiators using organocatalysts. Macromolecules. 2010;43:2664–2666.
- Clément B, Grignard B, Koole L, et al. Metal-free strategies for the synthesis of functional and well-defined polyphosphoesters. Macromolecules. 2012;45:4476–4486.
- Hiranphinyophat S, Asaumi Y, Fujii S, et al. Surface grafting polyphosphoesters on cellulose nanocrystals to improve the emulsification efficacy. Langmuir. 2019;35:11443–11451.
- Iwasaki Y. Bone mineral affinity of polyphosphodiesters. Molecules. 2020;25:758.
- Ishihara K, Ueda T, Nakabayashi N. Preparation of phospholipid polymers and their properties as polymer hydrogel membranes. Polym J. 1990;22:355–360.
- Goda T, Ishihara K. Soft contact lens biomaterials from bioinspired phospholipid polymers. Expert Rev Med Devices. 2006;3:167–174.
- Lewis AL, Tolhurst LA, Stratford PW. Analysis of a phosphorylcholine-based polymer coating on a coronary stent pre- and post-implantation. Biomaterials. 2002;23:1697–1706.
- Yamazaki K, Kihara S, Akimoto T, et al. EVAHEARTTM: an implantable centrifugal blood pump for long-term circulatory support. Jpn J Thorac Cardiovasc Surg. 2002;50:461–465.
- Moro T, Takatori Y, Ishihara K, et al. Surface grafting of artificial joints with a biocompatible polymer for preventing periprosthetic osteolysis. Nat Mater. 2004;3:829–836.
- Moro T, Takatori Y, Tanaka S, et al. Clinical safety and wear resistance of the phospholipid polymer-grafted highly cross-linked polyethylene liner. J Orthop Res. 2017;35:2007–2016.
- Kudo H, Sawada T, Kazawa E, et al. A flexible and wearable glucose sensor based on functional polymers with soft-MEMS techniques. Biosens Bioelectron. 2006;22:558–562.
- Arakawa T, Aota T, Iitani K, et al. Skin ethanol gas measurement system with a biochemical gas sensor and gas concentrator toward monitoring of blood volatile compounds. Talanta. 2020;219:121187.
- Iwasaki Y, Kurita K, Ishihara K, et al. Effect of methylene chain length in phospholipid moiety on blood compatibility of phospholipid polymers. J Biomater Sci Polym Ed. 1995;6:447–461.
- Ishihara K, Fujiike A, Iwasaki Y, et al. Synthesis of polymers having a phospholipid polar group connected to a poly(oxyethylene) chain and their protein adsorption-resistance properties. J Polym Sci A Polym Chem. 1996;34:199–205.
- Iwasaki Y, Ijuin M, Mikami A, et al. Behavior of blood cells in contact with water-soluble phospholipid polymer. J Biomed Mater Res. 1999;46:360–367.
- Iwasaki Y, Yamasaki A, Ishihara K. Platelet compatible blood filtration fabrics using a phosphorylcholine polymer having high surface mobility. Biomaterials. 2003;24:3599–3604.
- Mukai M, Higaki Y, Hirai T, et al. Separation of endo-cyclic 2-methacryloyloxyethyl choline phosphate by anion exchange approach. Chem Lett. 2018;47:1509–1511.
- Van Engeland M, Nieland LJW, Ramaekers FCS, et al. Annexin V-affinity assay: a review on an apoptosis detection system based on phosphatidylserine exposure. Cytometry. 1998;31:1–9.
- Ma HM, Wu Z, Nakanishi H. Phosphatidylserine-containing liposomes suppress inflammatory bone loss by ameliorating the cytokine imbalance provoked by infiltrated macrophages. Lab Investig. 2011;91:921–931.
- Nakagawa Y, Saitou A, Aoyagi T, et al. Apoptotic cell membrane-inspired polymer for immunosuppression. ACS Macro Lett. 2017;6:1020–1024.
- Nakagawa Y, Yano Y, Lee J, et al. Apoptotic cell-inspired polymeric particles for controlling microglial inflammation toward neurodegenerative disease treatment. ACS Biomater Sci Eng. 2019;5:5705–5713.
- Moszner N, Hirt T. New polymer-chemical developments in clinical dental polymer materials: enamel–dentin adhesives and restorative composites. J Polym Sci A Polym Chem. 2012;50:4369–4402.
- Watanabe I, Nakabayashi N, Pashley DH. Bonding to ground dentin by a phenyl-P self -etching primer. J Dent Res. 1994;73:1212–1220.
- Carrilho E, Cardoso M, Ferreira MM, et al. 10-MDP based dental adhesives: adhesive interface characterization and adhesive stability—A systematic review. Materials. 2019;12:790.
- Kepa K, Coleman R, Grøndahl L. In vitro mineralization of functional polymers. Biosurf Biotribol. 2015;1:214–227.
- Stokes AC. LXVII.—Some new forms of american rotifera.—II. Ann Mag Nat Hist. 1897;19:628–633.
- Allcock HR, Chen C. Polyphosphazenes: phosphorus in inorganic–organic polymers. J Org Chem. 2020;85:14286–14297.
- Teasdale I, Brüggemann O. Polyphosphazenes: multifunctional, biodegradable vehicles for drug and gene delivery. Polymers. 2013;5:161–187.
- Deng M, Kumbar SG, Wan Y, et al. Polyphosphazene polymers for tissue engineering: an analysis of material synthesis, characterization and applications. Soft Matter. 2010;6:3119–3132.
- Andrianov AK, Langer R. Polyphosphazene immunoadjuvants: historical perspective and recent advances. J Control Release. 2021;329:299–315.
- Ikeuchi R, Iwasaki Y. High mineral affinity of polyphosphoester ionomer-phospholipid vesicles. J Biomed Mater Res A. 2013;101:318–325.
- Wang YC, Liu XQ, Sun TM, et al. Functionalized micelles from block copolymer of polyphosphoester and poly(ε-Caprolactone) for receptor-mediated drug delivery. J Control Release. 2008;128:32–40.
- Steinbach T, Wurm FR. Poly(phosphoester)s: a new platform for degradable polymers. Angew Chem Int Ed. 2015;54:6098–6108.
- Steinbach T, Ritz S, Wurm FR. Water-soluble poly(phosphonate)s via living ring-opening polymerization. ACS Macro Lett. 2014;3:244–248.
- Bauer KN, Liu L, Andrienko D, et al. Polymerizing phostones: a fast way to in-chain poly(phosphonate)s with adjustable hydrophilicity. Macromolecules. 2018;51:1272–1279.
- Iwasaki Y, Akiyoshi K. Design of biodegradable amphiphilic polymers: well-defined amphiphilic polyphosphates with hydrophilic graft chains via ATRP. Macromolecules. 2004;37:7637–7642.
- Zhang S, Wang H, Shen Y, et al. A simple and efficient synthesis of an acid-labile polyphosphoramidate by organobase-catalyzed ring-opening polymerization and transformation to polyphosphoester ionomers by acid treatment. Macromolecules. 2013;46:5141–5149.
- Wang H, Su L, Li R, et al. Polyphosphoramidates that undergo acid-triggered backbone degradation. ACS Macro Lett. 2017;6:219–223.
- Momeni A, Filiaggi MJ. Synthesis and characterization of different chain length sodium polyphosphates. J Non Cryst Solids. 2013;382:11–17.
- Muller WE, Tolba E, Schroder HC, et al. Polyphosphate: a morphogenetically active implant material serving as metabolic fuel for bone regeneration. Macromol Biosci. 2015;15:1182–1197.
- Weg M, Tolba E, Feng Q, et al. Amorphous Ca2+ polyphosphate nanoparticles regulate the ATP level in bone-like SaOS-2 cells. J Cell Sci. 2015;128:2202–2207.
- Morrissey JH, Choi SH, Smith SA. Polyphosphate: an ancient molecule that links platelets, coagulation, and inflammation. Blood. 2012;119:5972–5979.
- Ishihara K, Nomura H, Mihara T, et al. Why do phospholipid polymers reduce protein adsorption? J Biomed Mater Res. 1998;39:323–330.
- Fukazawa K, Ishihara K. Synthesis of photoreactive phospholipid polymers for use in versatile surface modification of various materials to obtain extreme wettability. ACS Appl Mater Interfaces. 2013;5:6832–6836.
- Tanaka M, Iwasaki Y. Photo-assisted generation of phospholipid polymer substrates for regiospecific protein conjugation and control of cell adhesion. Acta Biomater. 2016;40:54–61.
- Tanaka M, Kawai S, Iwasaki Y. Well-defined protein immobilization on photo-responsive phosphorylcholine polymer surfaces. J Biomater Sci Polym Ed. 2017;28:2021–2033.
- Iwasaki Y, Bunuasunthon S, Hoven VP. Protein patterning with antifouling polymer gel platforms generated using visible light irradiation. Chem Commun. 2020;56:5472–5475.
- Iwasaki Y, Ishihara K. Cell membrane-inspired phospholipid polymers for developing medical devices with excellent biointerfaces. Sci Technol Adv Mater. 2012;13:064101.
- Ueda T, Oshida H, Kurita K, et al. Preparation of 2-methacryloyloxyethyl phosphorylcholine copolymers with alkyl methacrylates and their blood compatibility. Polym J. 1992;24:1259–1269.
- Iwasaki Y, Mikami A, Kurita K, et al. Reduction of surface-induced platelet activation on phospholipid polymer. J Biomed Mater Res. 1997;36:508–515.
- Ishihara K, Ziats NP, Tierney BP, et al. Protein adsorption from human plasma is reduced on phospholipid polymers. J Biomed Mater Res. 1991;25:1397–1407.
- Ishihara K. Blood-compatible surfaces with phosphorylcholine-based polymers for cardiovascular medical devices. Langmuir. 2019;35:1778–1787.
- Stoodley P, Sauer K, Davies DG, et al. Biofilms as complex differentiated communities. Annu Rev Microbiol. 2002;56:187–209.
- Zander ZK, Becker ML. Antimicrobial and antifouling strategies for polymeric medical devices. ACS Macro Lett. 2018;7:16–25.
- Kang S, Lee M, Kang M, et al. Development of anti-biofouling interface on hydroxyapatite surface by coating zwitterionic MPC polymer containing calcium-binding moieties to prevent oral bacterial adhesion. Acta Biomater. 2016;40:70–77.
- Noree S, Thongthai P, Kitagawa H, et al. Reduction of acidic erosion and oral bacterial adhesion through the immobilization of zwitterionic polyphosphoesters on mineral substrates. Chem Lett. 2019;48:1529–1532.
- Thongthai P, Kitagawa H, Kitagawa R, et al. Development of novel surface coating composed of MDPB and MPC with dual functionality of antibacterial activity and protein repellency. J Biomed Mater Res B. 2020;108:3241–3249.
- Ishihara K. Highly lubricated polymer interfaces for advanced artificial hip joints through biomimetic design. Polym J. 2015;47:585–597.
- Kyomoto M, Moro T, Miyaji F, et al. Effects of mobility/immobility of surface modification by 2-methacryloyloxyethyl phosphorylcholine polymer on the durability of polyethylene for artificial joints. J Biomed Mater Res A. 2009;90:362–371.
- Chen M, Briscoe WH, Armes SP, et al. Lubrication at physiological pressures by polyzwitterionic brushes. Science. 2009;323:1698–1701.
- Ishihara K, Iwasaki Y, Ebihara S, et al. Photoinduced graft polymerization of 2-methacryloyloxyethyl phosphorylcholine on polyethylene membrane surface for obtaining blood cell adhesion resistance. Colloids Surf B. 2000;18:325–335.
- Moro T, Kawaguchi H, Ishihara K, et al. Wear resistance of artificial hip joints with poly(2-methacryloyloxyethyl phosphorylcholine) grafted polyethylene: comparisons with the effect of polyethylene cross-linking and ceramic femoral heads. Biomaterials. 2009;30:2995–3001.
- Kyomoto M, Moro T, Saiga K, et al. Biomimetic hydration lubrication with various polyelectrolyte layers on cross-linked polyethylene orthopedic bearing materials. Biomaterials. 2012;33:4451–4459.
- Kurtz SM, Devine JN. PEEK biomaterials in trauma, orthopedic, and spinal implants. Biomaterials. 2007;28:4845–4869.
- Panayotov IV, Orti V, Cuisinier F, et al. Polyetheretherketone (PEEK) for medical applications. J Mater Sci Mater Med. 2016;27:118.
- Buck E, Li H, Cerruti M. Surface modification strategies to improve the osseointegration of poly(etheretherketone) and its composites. Macromol Biosci. 2020;20:1900271.
- Kyomoto M, Ishihara K. Self-initiated surface graft polymerization of 2-methacryloyloxyethyl phosphorylcholine on poly(ether ether ketone) by photoirradiation. ACS Appl Mater Interfaces. 2009;1:537–542.
- Nakano H, Noguchi Y, Kakinoki S, et al. Highly durable lubricity of photo-cross-linked zwitterionic polymer brushes supported by poly(ether ether ketone) substrate. ACS Appl Bio Mater. 2020;3:1071–1078.
- Dorozhkin SV. Bioceramics of calcium orthophosphates. Biomaterials. 2010;31:1465–1485.
- Liu X, Chu PK, Ding C. Surface modification of titanium, titanium alloys, and related materials for biomedical applications. Mater Sci Eng R Rep. 2004;47:49–121.
- Durham JW, Montelongo SA, Ong JL, et al. Hydroxyapatite coating on PEEK implants: biomechanical and histological study in a rabbit model. Mater Sci Eng C. 2016;68:723–731.
- Suzuki S, Grøndahl L, Leavesley D, et al. In vitro bioactivity of MOEP grafted ePTFE membranes for craniofacial applications. Biomaterials. 2005;26:5303–5312.
- Suzuki S, Whittaker MR, Grøndahl L, et al. Synthesis of soluble phosphate polymers by RAFT and their in vitro mineralization. Biomacromolecules. 2006;7:3178–3187.
- Penczek S, Kaluzynski K, Pretula J. Polyethylene–CaCO3 hybrid via CaCO3-controlled crystallization in emulsion. J Polym Sci A Polym Chem. 2011;49:1289–1292.
- Penczek S, Pretula J, Kaluzynski K. Synthesis of a triblock copolymer: poly(ethylene glycol)-poly(alkylene phosphate)-poly(ethylene glycol) as a modifier of CaCO3 crystallization. J Polym Sci A Polym Chem. 2005;43:650–657.
- Penczek S, Kaluzynski K, Pretula J. Determination of copolymer localization in polymer—CaCO3 hybrids formed in mediated crystallization. J Polym Sci A Polym Chem. 2009;47:4464–4467.
- Penczek S, Pretula J, Kaluzynski K. Poly(alkylene phosphates): from synthetic models of biomacromolecules and biomembranes toward polymer−inorganic hybrids (mimicking biomineralization). Biomacromolecules. 2005;6:547–551.
- Penczek S, Pretula JB, Kaluzynski K, et al. Polymers with esters of phosphoric acid units: from synthesis, models of biopolymers to polymer−inorganic hybrids. Isr J Chem. 2012;52:306–319.
- Kunomura S, Iwasaki Y. Immobilization of polyphosphoesters on poly(ether ether ketone) (PEEK) for facilitating mineral coating. J Biomater Sci Polym Ed. 2019;30:861–876.
- Tanahashi M, Yao T, Kokubo T, et al. Apatite coating on organic polymers by a biomimetic process. J Am Ceram Soc. 1994;77:2805–2808.
- Pan H, Sima M, Kopečková P, et al. Biodistribution and pharmacokinetic studies of bone-targeting N-(2-hydroxypropyl)methacrylamide copolymer−alendronate conjugates. Mol Pharm. 2008;5:548–558.
- Low SA, Kopecek J. Targeting polymer therapeutics to bone. Adv Drug Deliv Rev. 2012;64:1189–1204.
- Segal E, Pan H, Ofek P, et al. Targeting angiogenesis-dependent calcified neoplasms using combined polymer therapeutics. PLoS One. 2009;4:e5233.
- Miller K, Clementi C, Polyak D, et al. Poly(ethylene glycol)-paclitaxel-alendronate self-assembled micelles for the targeted treatment of breast cancer bone metastases. Biomaterials. 2013;34:3795–3806.
- Yang W, Liu S, Bai T, et al. Poly(carboxybetaine) nanomaterials enable long circulation and prevent polymer-specific antibody production. Nano Today. 2014;9:10–16.
- Otaka A, Yamaguchi T, Saisho R, et al. Bone-targeting phospholipid polymers to solubilize the lipophilic anticancer drug. J Biomed Mater Res A. 2020;108:2090–2099.
- Iwasaki Y, Yokota A, Otaka A, et al. Bone-targeting poly(ethylene sodium phosphate). Biomater Sci. 2018;6:91–95.
- Otaka A, Kiyono K, Iwasaki Y. Enhancement of osteoblast differentiation using poly(ethylene sodium phosphate). Materialia. 2021;15:100977.
- Kootala S, Tokunaga M, Hilborn J, et al. Anti-resorptive functions of poly(ethylene sodium phosphate) on human osteoclasts. Macromol Biosci. 2015;15:1634–1640.
- Wu J, Ma G. Recent studies of pickering emulsions: particles make the difference. Small. 2016;34:4633–4648.
- Binks BP, Kirkland M. Interfacial structure of solid-stabilised emulsions studied by scanning electron microscopy. Phys Chem Chem Phys. 2002;4:3727–3733.
- Aveyard R, Binks BP, Clint JH. Emulsions stabilised solely by colloidal particles. Adv Colloid Interface Sci. 2003;100-102:503–546.
- Hu JW, Yen MW, Wang AJ, et al. Effect of oil structure on cyclodextrin-based pickering emulsions for bupivacaine topical application. Colloids Surf B. 2018;161:51–58.
- Che K-M, Zhang M-Z, He J-L, et al. Polyphosphoester-modified cellulose nanocrystals for stabilizing pickering emulsion polymerization of styrene. Chinese J Polym Sci. 2020;38:921–931.
- Hiranphinyophat S, Otaka A, Asaumi Y, et al. Particle-stabilized oil-in-water emulsions as a platform for topical lipophilic drug delivery. Colloids Surf B. 2021;197:111423.