ABSTRACT
Printed electronics implies the use of low-cost, scalable, printing technologies to fabricate electronic devices and circuits on flexible substrates, such as paper or plastics. The development of this new electronic is currently expanding because of the emergence of the internet-of-everything. Although lot of attention has been paid to functional inks based on organic semiconductors, another class of inks is based on nanoparticles obtained from exfoliated 2D materials, such as graphene and metal sulfides. The ultimate scientific and technological challenge is to find a strategy where the exfoliated nanoparticle flakes in the inks can, after solvent evaporation, form a solid which displays performances equal to the single crystal of the 2D material. In this context, a printed layer, formed from an ink composed of nano-flakes of TiS2 intercalated with hexylamine, which displays thermoelectric properties superior to organic intercalated TiS2 single crystals, is demonstrated for the first time. The choice of the fraction of exfoliated nano-flakes appears to be a key to the forming of a new self-organized layered material by solvent evaporation. The printed layer is an efficient n-type thermoelectric material which complements the p-type printable organic semiconductors The thermoelectric power factor of the printed TiS2/hexylamine thin films reach record values of 1460 µW m−1 K−2 at 430 K, this is considerably higher than the high value of 900 µW m−1 K−2 at 300 K reported for a single crystal. A printed thermoelectric generator based on eight legs of TiS2 confirms the high-power factor values by generating a power density of 16.0 W m−2 at ΔT = 40 K.
Graphical abstract
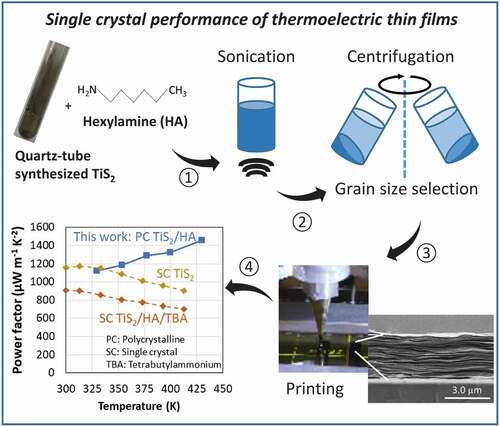
1. Introduction
The interest for printable and flexible thermoelectric devices has increased in recent years as a result of many potential applications such as wearable energy harvesting [Citation1–7], the powering of wireless sensors for the internet-of-everything [Citation8,Citation9], ultra-sensitive thermopiles [Citation10], etc. Of these different materials, p-type printed thermoelectric devices based on organic semiconductors have achieved reasonable performances [Citation11,Citation12]. zTs values in the range of 0.3–0.4 at room temperature were obtained with the polymer poly(3,4-ethylenedioxy-thiophene):polystyrene sulfonic acid (PEDOT:PSS) by tuning its level of doping [Citation13,Citation14]. Besides its low cost, the advantage of such a polymer is its intrinsic low thermal conductivity (~0.3 W m−1 K−1 for the through-plane value and below 1 W m−1 K−1 for the in-plane value) [Citation15] and its high-power factor (PF) of approximately 470 µW m−1 K−2. Another class of printable compounds based on exfoliated 2D materials was considered. The reason is their low dimensionality, a feature favorable for high performances [Citation16]. Exfoliated organic compounds such as graphene have not been able to compete with PEDOT:PSS because of its intrinsic high thermal conductivity [Citation17]. On the other hand n-type exfoliated inorganic thermoelectric materials based on metal chalcogenide compounds were considered as promising, due to their low cost, environmental friendliness and the high reported power factor of their bulk form [Citation18–20]. One of these compounds, TiS2 was recently used to prepare new n-type hybrid TiS2/organic superlattice materials as efficient n-type counterparts to the printed p-type PEDOT:PSS films. Hence, this choice of n- and p-type thermoelectric materials is today the most promising for the fabrication of efficient thermoelectric generators based on printing processes without high-temperature treatments, i.e. enabling a range of flexible substrates such as paper and plastics which are crucial in packaging and their further implementation in the internet-of-everything [Citation21].
The n-type hybrid TiS2/organic superlattice materials were synthesized firstly by an electrochemical intercalation of hexylammonium (HA+) cation in single crystals of TiS2 and co-intercalation of the dimethyl sulfoxide (DMSO) solvent [Citation22]. Part of the solvent was then exchanged with H2O to yield TiS2[(HA)x(H2O)y(DMSO)z]. In this compound the thermal conductivity of the composite layered material was reduced to 0.69 W m−1 K−1 at room temperature. Its in-plane power factor was furthermore measured at 450 µW m−1 K−2 and the zT was calculated to be 0.28 at 373 K, which is 3 times higher than TiS2. It is known both for organic and inorganic materials, that tuning the charge carrier concentration enables the optimization of the power factor because the Seebeck coefficient and electrical conductivity evolve in opposite directions with charge carrier concentration [Citation23,Citation24]. This was implemented by replacing the solvents co-intercalated with HA+ by tetrabutylammonium (TBA) and the power factor was then increased to 900 µW m−1 K−2 at room temperature (RT) [Citation25]. TBA has a higher boiling point and higher molecular weight than HA+. In this new system, the thermal conductivity remained quite low and in consequence an increase of zTs to 0.31 at 373 K and 0.33 at 430 K was observed.
The costly process relying on TiS2 single crystals was replaced and the electrochemical intercalation process was modified. Hence polycrystalline TiS2 powder was prepared and intercalated with HA by mechanical treatment [Citation26,Citation27]. The resulting compound was further processed in solution to yield thin films with a power factor of about 210 µW m−1 K−2 at RT. More recently, similar power factor of about 210 µWm−1K−2 were obtained on TiS2[HA]x nanocomposite printed on a paper substrate [Citation28]. Although the power factor was more than 4 times lower than the intercalated TiS2 single crystal, these studies demonstrated the potential of solution process for fabricating 2D thermoelectric inorganic nanomaterial layers. This technology based on hybrid intercalated TiS2 materials was also the subject of patent applications [Citation29,Citation30]. Further studies to increase the power factor, a composite of polycrystalline TiS2 and fullerene was carried out [Citation31]. In this system assembling C60 molecules on the surface of the TiS2 nanolayers led to a PF which was doubled compared to the paper cited above. However, it still lies far below the performance achieved with the intercalated single crystal. We sum up the results of all those studies on TiS2 in .
Figure 1. The power factor of this work vs. the previously reported values. The power factor of the intercalated TiS2 single crystal is represented by a dashed line; while the intercalated TiS2 polycrystalline is identified with a continuous line, with in addition the red point
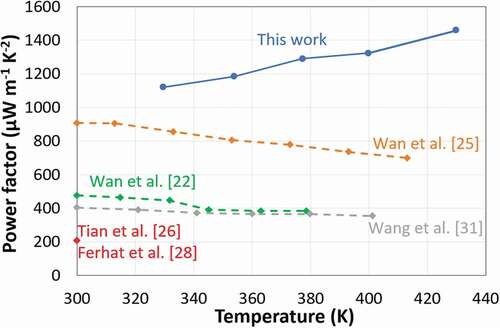
In this study, we show that it is possible to intercalate HA in polycrystalline TiS2 to get power factors of about 1400 µW m−1 K−2 at 420 K, i.e. higher than the intercalated TiS2 single crystal (). Moreover, we demonstrate a low-cost manufacturing process based on the deposition of high performance TiS2 films from a solution process at low temperature. This work paves the way for a possible replication of multiple patterns of p- and n-legs by printing technologies; which is required to reach the thermo-voltage up to useful values in electronics (order of 1 volt).
2. Experimental section
2.1. Synthesis of TiS2 powder
The TiS2 powder was synthesized by a solid-state reaction [Citation32] from stoichiometric amounts of Ti (Goodfellow) and S (Sigma Aldrich) powders. The precursors were mixed in a mortar, poured into a quartz ampoule that was sealed under vacuum, placed in a vertical furnace and subsequently heated at 700°C for 24 h. The mixture was finally cooled to RT. The recovered compound was ground in a mortar and analyzed by X-ray diffraction (XRD). The pattern corresponded to hexagonal TiS2 with space group P-3m1 (164) [Citation33]. Some sublimated sulfur was found on the inner wall which could indicate a slightly stoichiometric deviation towards S-rich synthesis conditions. Previous reports have shown that the excess of Ti can intercalate in the Van der Waals gap of TiS2 greatly improving the thermoelectric properties of the compound [Citation19].
2.2. Intercalation of hexylamine in TiS2
The intercalation of hexylamine in TiS2 as well as the ink formulation process are described and discussed in the core of this paper.
2.3. Printing of the films
The as-prepared ink was poured in a cartridge, which was installed in a dispenser and the dispenser mounted on the V-One printer from Voltera (Canada). The ink was then dispensed via a nozzle onto the desired substrate. The movement of the dispenser in 3 directions was computer-controlled that allowed to print AutoCAD draw patterns. The samples printed on a glass substrate or Kapton® tape had a size of 2 × 15 mm2 and a thickness of about 2.5 µm.
2.4. Structural characterizations
The scanning electron microscopy (SEM) investigations were carried out on a Hitachi (Japan) S-4700 equipped with a field emission gun. The XRD patterns were collected at room temperature on thin films printed on glass substrates using a Bruker (Germany) D8 diffractometer equipped with a copper anode and a point detector.
2.5. Thermoelectric characterization of the films
The electrical resistivity (ρ) and the Seebeck coefficient (S) of the films deposited on glass were directly measured as a function of temperature under a helium atmosphere on a Linseis (Germany) LSR-3 setup. The electrical conductivity (σ) was then calculated via σ = 1/ρ. The thickness of the films was measured by SEM analysis of their cross-sections. The samples for these characterizations were deposited on glass substrates and had 4 × 20 mm2 in size and about 2.5 µm in thickness. The thermal conductivity (κ) was measured on films which were deposited by Blade casting on chips supplied free of charge by Linseis. The chips were then sent to Linseis and their thermal conductivity was measured using the Thin Film Analyzer of the company [Citation34]. The figure of merit zT was then calculated via zT = (S2*σ*T)/κ. Hall effects measurements were made on an Ecopia (Korea) HMS-5500 material mounted with an AHT55T5 high temperature magnet. The voltage generated by the TEG stacks was measured using a home-made set up based on a Peltier device as hot source and ambient air as cold source.
3. Results and discussion
3.1. Process for the fabrication of the TiS2/HA thin films
The different steps of the fabrication process are shown in . Steps a and b are similar to those published by Tian et al. [Citation26] However, it is worth mentioning that the quality of the TiS2 powder seems to be an important parameter. The formulation process carried out with a commercially available TiS2 powder always led to thin films with much lower thermoelectric performances. Scanning electron microscopy observations revealed a different crystal morphology between the two samples and a narrower particle size distribution for the self-made TiS2 that led to films with a perfect layered structure. The commercially available TiS2 did not lead to such a continuous layered structure as shown in Note S1 and Figure S1 (Supporting Information), which could explain the lower performances of its films reproduced in Figure S2 (Supporting Information). The formulation process presented is performed on TiS2 powder which is not exposed to air and the entire formulation process is performed in an argon atmosphere with a O2 level below 1 ppm. In step c, a two-stage centrifugation is applied. First, a centrifugation speed of 1000 rpm is used to remove the large particles (Figure S3 and S4, Supporting Information). Then, the supernatant is recovered and instead of using it directly as precursor for the films, it is centrifuged in a second stage at a higher speed of 9000 rpm. The sediment obtained is collected and used as the ink for further preparation of the films. The proposed formulation process is more adapted to a future scale-up using ink as a precursor of the films rather than evaporating the solvent of a supernatant to create the films on substrates. Another notable feature of this method is that the two-stage centrifugation removes the big particles from the ink as demonstrated by the SEM images shown in Note S2 and Figure S3 (Supporting Information). The ink could be then deposited by various printing technologies such as blade casting or dispenser printing. The final films were heated at 90°C for 30 minutes to remove the excess of HA and N-methylformamide.
Figure 2. Process for the preparation of Hybrid TiS2/HA thin films. (a) Intercalation of TiS2/hexylamine 1/3 molar ratio by mixing and grinding of the chemicals. (b) Dispersion of the slurry in N-methylformamide and sonication of the suspension. (c) Centrifugation at 1000 rpm of the recovered material. (d) Supernatant recovered and centrifuged at 9000 rpm. Sediment separated from the supernatant and further used as ink. (e) Deposition of the film either by blade casting or dispenser printing. (f) Film drying at 90°C for 30 minutes
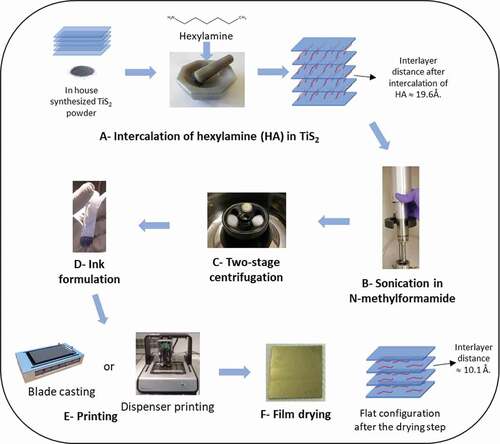
3.2. Investigations on the intercalation process
The X-ray diffraction (XRD) patterns collected after various steps of the formulation process are shown in . After mixing the hexylamine with TiS2 ()) the XRD detects two crystal phases. The pristine TiS2 is identified by its two main peaks, namely (001) and (101) located at 15.6° and 34.2° in 2-theta, respectively. The presence of the hybrid TiS2/HA material is detected by a series of (00 l) peaks with l = 1, 2, 3 and 4 ()). This series of peaks indicates that the hexylamine has been intercalated between the (001) planes of TiS2.
The main peak of the hybrid material located at 4.5° corresponds to a distance of 19.6 Å. According to A. Weiss et al. [Citation35] the intercalation of alkylamines CnH2n+1NH2 (4 < n < 17) between the TiS2 layers leads to an increase of interlayer distance and the organic molecules organize with a tilt angle compared to the TiS2 plane that depends on their length. For hexylamine this angle is about 56° [Citation35]. During the drying step ()) at elevated temperature the arrangement of the organic molecules inside the hybrid TiS2/HA material changes. The main (00 l) peak located at 4.5° in 2-theta is shifted towards a higher 2-theta angle of 8.7°. This corresponds to the modification of the interlayer distance in the hybrid material from 19.6 Å to 10.1 Å ()). This modification of the interlayer distance corresponds to a reorganization of the organic molecules between the TiS2 sheets. After the drying step the intercalated HA molecules form a monolayer sandwiched in the Van der Waals gap of TiS2 and lie parallel to the TiS2 sheets [Citation26].
3.3. Microstructure characterization of the thin films
SEM micrographs of a printed TiS2 film on a glass substrate are shown in . The cross-section view reveals the layered structure based on the hybrid inorganic-organic TiS2/HA. Hence, even when processed from solution, the evaporation of the suspension of TiS2 particles leads to a spontaneous alignment of the intercalated TiS2 sheets parallel to the substrate. Such a well-organized alignment has been attributed to the highly anisotropic character of the TiS2/HA nanosheets and to the forces which are exerted by surface tension while the solvent evaporates [Citation27]. Furthermore, few typical hexagonal flakes of the TiS2 material are visible at low and high magnifications top-view SEM images ()) despite the energetic mechanical process of exfoliation. The fact that it is possible to see the structure underneath the TiS2 flakes indicates their extremely thin nature. The two-stage centrifugation process allowed the selection of TiS2 flakes with a lateral size varying from a few hundred nanometers for the smallest up to 3000 nm for the largest.
Figure 4. SEM images of a film prepared by blade casting. (a) Cross-section view. (b) Top view at low magnification showing that the film is composed of 2D thin flakes, (c) Top view at high magnification showing a characteristic hexagonal shape of TiS2 flake
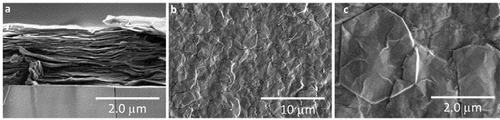
Further analysis of the thin film microstructure suggests a good agreement between the observed SEM observations and the collected XRD patterns. Indeed, the TiS2/HA grains lying parallel to the substrate ()) cause a strong diffraction from the (00 l) planes (). It is worth mentioning that the XRD pattern collected indicates a superior phase purity and crystallinity of thin films in comparison to previous reports. In particularly, Tian et al. [Citation26] observed two intercalated phases called A and B; while in this work only the intercalated phase B is detected, which corresponds to dried hybrid TiS2/HA crystals. The film in these experiments has therefore a high crystal phase purity. Furthermore, the crystallinity of TiS2/HA crystals in this film is better as the full width at half maximum (FWHM) of the peaks is narrower than for the corresponding peaks of previously reported films (e.g. the peak at 8.7° has a FWHM of 0.233° in our film and 1.06° in the film of Tian et al.) ()). Similar trend is observed in comparison to Wan et al. [Citation27] ()) where narrow FWHM on B1, B2 and B3 peaks indicate a high crystallinity in our films (e.g. FWHM of B1 is 0.233° while it is 1.25° in the previous study). Finally, very recently, Gu et al. [Citation36] observed a significant increase of the thermoelectric power factor on a mechanically exfoliated and restacked at high-temperature bulk pristine TiS2. In this independent study Gu et al. also demonstrate that the crystal quality seems to be the key parameter in achieving the high thermoelectric properties in 2D based materials.
Figure 5. Comparison of the X-ray diffraction diagrams collected on the TiS2/HA films in this publication and (a) Tian et al. [Citation26] and (b) Wan et al. [Citation27]. Redrawn with the permissions of The Royal Society of Chemistry and Elsevier
![Figure 5. Comparison of the X-ray diffraction diagrams collected on the TiS2/HA films in this publication and (a) Tian et al. [Citation26] and (b) Wan et al. [Citation27]. Redrawn with the permissions of The Royal Society of Chemistry and Elsevier](/cms/asset/0d4cbbc1-66a5-417f-a130-2791a7c30334/tsta_a_1978802_f0005_oc.jpg)
3.4. Thermoelectric properties of the thin films
In the past, several theoretical and experimental investigations have been carried to explain transport properties of Ti1+xS2 systems [Citation19,Citation20,Citation37–39]. Authors agree that a perfect TiS2 crystal is a narrow band gap semiconductor that tends to have a (semi)metallic behavior because of the titanium interstitial defects (Tii) located in the van der Waals gaps. It means, that for low concentrations of Tii the material keeps its semiconducting behavior with a relatively high Seebeck coefficient while for high Tii concentrations the material behaves like a semimetal with a low Seebeck coefficient. Experimental data confirms the sensitivity of the Seebeck coefficient to small excess of Ti (about 1% excess of Ti leads to a decrease of the Seebeck coefficient by more than half its value [Citation19,Citation39]). Hence, reports in the literature might include a slight difference in the purity of the TiS2, which is difficult to detect but may significantly affect the electrical conductivity and the Seebeck coefficient of the TiS2 before the hexylamine intercalation.
The electrical conductivity and the Seebeck coefficient of the printed TiS2/HA are 740 S cm−1 and −123 µV K−1 at 330 K, respectively. These values are superior to the one reported for an intercalated TiS2/HA/DMSO single crystal (650 S cm−1 and −78 µV K−1 at 330 K) [Citation25]. Remarkably, the power factor of the micrometer thick films formed by the printing process increases from 1120 µW m−1 K−2 at 330 K to 1460 µW m−1 K−2 at 430 K at the best. The comparison of the power factor at 420 K demonstrates superior thermoelectric performances (i.e. 1400 µW m−1 K−2) in comparison to hybrid TiS2/HA single crystals (700 µW m−1 K−2) reported by Wan et al. and other solution-processed layers (210 µW m−1 K−2) reported by Tian et al. The electrical conductivity, Seebeck coefficient and the resulting power factor versus temperature are presented in . As mentioned above the variation of the TiS2 stoichiometry might be one possible, although speculative, explanation for the systematically higher thermoelectric properties of the printed TiS2/HA thin films. However, all the reported HA intercalated TiS2 undergo an increase of electrical conductivity and a decrease in Seebeck coefficient. The intercalation of HA is driven by a charge transfer process between amine (slight reducing agent) and TiS2 forming bonds with a polar/ionic character due to the interaction between the lone pair of the nitrogen and the 3d orbitals of the Ti atoms: RNH2 + TiS2 → (RNH2)+(TiS2)−. This mechanism implies that HA takes an active role in the modification of the electronic density at the HA/TiS2 interface and increase the charge carrier density, thus contributing to a rise in conductivity and decrease in Seebeck coefficient [Citation26]. Hence, we truly speak about a new material formed upon intercalation, also called a hybrid organic-inorganic superlattice of TiS2. In those TiS2/HA superlattices, the crystallinity seems to play an essential role on the thermoelectric performance; which is a challenge when films are formed by exfoliated nanosheets. The degree of disorder in a semiconductor is expected to smoothen the band edge and decrease the Seebeck coefficient as well as the electrical conductivity. Another reason for the superior thermoelectric performance of our printed film compared to other solution-processed TiS2/HA films is the low degree of disordered as reflected by a good crystallinity (see ). Finally, it is worth to mention here that further increase of the thermoelectric power factor may be still possible as the record value for pristine single crystal TiS2 is as high as 3710 µW m−1 K−2 at 300 K [Citation20].
The printed TiS2/HA thin films exhibit a sensitively higher charge carrier concentration of about 2.34 × 1021 cm−3 than on the previously reported on a polycrystalline TiS2/HA film and intercalated TiS2 single crystal that are 1.6 × 1021 cm−3 and 7.59 × 1020 cm−3, respectively. Using the charge carrier concentration, the intercalation level of the hexylamine into TiS2 is estimated as follows. First, it is assumed that each intercalated hexylamine brings an additional charge into TiS2. Then, the data published by M. Beaumale et al. [Citation19] et H. Imai et al. [Citation20] are used as references of pristine TiS2 with a charge carrier concentration of 1.1 × 1020 and 2.8 × 1020 cm−3, respectively. At the end, the additional charges introduced by the hexylamine intercalation are estimated to be about 2.06 × 1021 and 2.23 × 1021 cm−3 so the resulting intercalation level is about 12–13% in respect of the Ti atoms in the TiS2. It means that each eighth Ti atom of the TiS2 is affected by the hexylamine intercalation. Similar considerations lead to intercalation levels of about 8 and 3% for TiS2/HA obtained by Tian et al. and Wan et al., respectively.
Apart the high charge carrier concentration with a high intercalation level, a relatively high electron mobility of 3.22 cm2.V−1.s−1 at 293 K is observed. It remains lower than the value reported for the intercalated TiS2 single crystal (6.41 cm2.V−1.s−1) but higher than the value observed on polycrystalline TiS2/HA (2.4 cm2.V−1.s−1). Such values make sense as the electrons are preferentially located on the inorganic TiS2 flakes. Finally, the average rate increase of the Seebeck coefficient versus temperature is higher for the hybrid printed layer (34 µV K−1 for ΔT = 50 K) than for the hybrid single crystal (17 µV K−1 for ΔT = 50 K). These differences in thermoelectric properties could be an indicator of higher effective mass in the hybrid printed TiS2/HA films. In conclusions, the enhancement of the thermoelectric properties of the hybrid TiS2/HA materials is a result of the fabrication process that leads to higher crystal quality expressed by higher crystal phase purity, larger lateral size of the TiS2 flakes and better ordering of the hybrid TiS2/HA material.
Figure 6. In-plane thermoelectric properties of a film from this work in comparison to previous reports on single crystal TiS2 (SC TiS2) [Citation19,Citation20], single crystal TiS2 intercalated with hexylamine and DMSO (SC TiS2/HA/DMSO) and single crystal TiS2 intercalated with hexylamine and tetrabutylammonium (SC TiS2/HA/TBA) [Citation22,Citation25]. Temperature dependence of the electrical resistivity (a), the Seebeck coefficient (b) and the power factor (c).(The measurements were carried out for a heating and cooling with an accuracy for the Seebeck coefficient of ± 7%, for the electrical conductivity is ± 8%, for the power factor is ± 15%)
![Figure 6. In-plane thermoelectric properties of a film from this work in comparison to previous reports on single crystal TiS2 (SC TiS2) [Citation19,Citation20], single crystal TiS2 intercalated with hexylamine and DMSO (SC TiS2/HA/DMSO) and single crystal TiS2 intercalated with hexylamine and tetrabutylammonium (SC TiS2/HA/TBA) [Citation22,Citation25]. Temperature dependence of the electrical resistivity (a), the Seebeck coefficient (b) and the power factor (c).(The measurements were carried out for a heating and cooling with an accuracy for the Seebeck coefficient of ± 7%, for the electrical conductivity is ± 8%, for the power factor is ± 15%)](/cms/asset/2051a55d-8bfd-4088-b677-88d6c199d758/tsta_a_1978802_f0006_oc.jpg)
3.5. Influence of the process parameters on the properties of films
Several parameters of the process were tested before finding the optimal conditions. All the experimental details are given in the Supporting Information. First, the impact of the TiS2 particle size was investigated. For this purpose, the TiS2/HA ink was prepared from a mildly ground home-made TiS2 powder (Note S3 and Figure S6, Supporting Information). The reduction of the particle size from few micrometers to below 1 micrometer dramatically impacts the transport properties. Indeed, the electrical conductivity of films is reduced by two orders of magnitude (Table S1, Supporting Information). The hall effect measurements indicate that the initial size of a few micrometers is therefore ideal for the electron transport. Furthermore, the ink formulation process was carried out with different amines such as undecylamine. In all cases the films’ performances were lower than with hexylamine as intercalant, mainly because of a better processability of the latter amine (Note S4 and Figure S7, Supporting Information).
Finally, both of the ink and films aging were investigated. Highest performance is obtained when the film is prepared from a 9000 rpm ink which is only a few hours old. The properties gradually degrade as the ink ages (Note S5 and Figure S8, Supporting Information). In addition, jellification of the ink is observed while it ages. We suspect that a self-ordering between the 2D TiS2 flakes and HA molecules leads to the jellification of the ink. It was also concluded that to achieve high power factors the TiS2/HA films need to be deposited using non- jellified ink. A similar jellification process was observed on a sonicated MoS2 and WS2 but the mechanism of the viscosity modification remains unclear [Citation40]. The explanation of this mechanism could be the purpose of another study which should include considerations about the oxidation of the TiS2 in the presence of water or oxygen. The mechanism of oxidation was investigated elsewhere by modeling the oxidation pathways of TiS2 [Citation41,Citation42]. It was pointed out that such oxidation occurs more easily at the edges of flakes or in the middle of the flakes at sites where sulfur vacancies exist. The influence of the replacement of S atoms by O atoms leads to a bandgap opening which is expected to affect the Seebeck coefficient of the compound and of its intercalated derivatives. In the entire process described in this paper the compounds are exposed to an inert atmosphere ([O2] = [H2O] = ~1ppm). These conditions might be optimal to avoid the oxidation of TiS2 and therefore achieve a high Seebeck coefficient in comparison to the thin films reported elsewhere.
Figure 7. Influence of the films aging on their properties. Temperature dependence of the in-plane power factor of a film 4 days and 19 days after its preparation
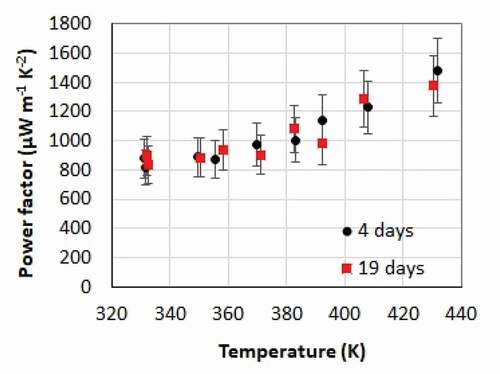
The thermoelectric properties of a film were measured 4 days and 19 days after its deposition. The power factor is presented versus temperature in . No significant variation is observed. This means that once a film has been deposited, it will remain stable in N2 for at least 19 days.
In order to estimate the zT of the TiS2/HA films, the measurement of their thermal conductivity is performed by the 3 ω method. Samples are prepared by applying the precursor ink onto Si chips specially designed for this purpose and provided by Linseis [Citation34]. The in-plane thermal conductivity varies from 1.20 W m−1 K−1 at 330 K to 1.00 W m−1 K−1 at 430 K for a 2 µm thick film (Figure S9, Supporting Information). The power factor of such a film varies from 800 µW m−1 K−2 at 330 K to 1000 µW m−1 K−2 at 430 K. The zT increases from 0.2 to 0.4 when the temperature rises from 330 K to 430 K, which is the highest zT reported for n-type printed thermoelectric materials.
3.6. Fabrication of a thermoelectric generator by dispenser printing
Next, the dispenser printer (V-One from Voltera) is used in order to create patterns, i.e. thermoelectric legs, with the TiS2/HA inks ()) either on glass substrate or Kapton® tape. The legs were then connected in series by printing wires of silver paste while copper pieces were used as external contacts for the printed thermoelectric generator stacks (TEG stacks, see Note S6, Figure S10 and Table S2, Supporting Information).
The power output of a TEG stack made of eight connected legs on glass substrate was evaluated ()). The resistance of the stack is 250 Ω. An open circuit voltage of 30.1 mV is obtained for a temperature gradient of 40 K. The maximum generated power output is 0.713 µW. This outstanding value is almost 3 times above the one reported by Tian et al. for such a temperature gradient. This level of performance would be suitable for low-power wide-area network (LPWAN) [Citation43]. The films have a length of 15 mm, a width of 2 mm and an average thickness of 2.79 µm as shown in Figure S11 (Supporting Information). The power density of the TEG is then calculated at a value of 16.0 W m−2, which is also much higher than the values previously reported for the hybrid TiS2 superlattices for the same ΔT of 40 K (0.8 W m−2).
The individual TEG stacks made of 8 connected legs were connected in series to prepare TEG modules of 1, 2 or 3 stacks. A photograph of module with 3 stacks is shown in ) and the performance of the modules as a function of the number of stacks in ). The voltage generated for a ΔT around 15°C increases gradually as the number of stacks is increased. Note that the measured open-circuit voltage for 2-stack (24 mV) and 3-stack (32 mV) TEG (connected in series) is lower than the calculated voltages (30 mV for 2 stack and 45 mV for 3 stack) due to some defects on the TiS2/HA films and quality of contacts TiS2/HA-Ag contacts.
Figure 8. The printed TEG stack and module based on TiS2/HA films. (a) Sketch of a TEG stack made of eight TiS2/HA legs connected in series. (b) Power output generated by the TEG stack at different temperature gradients. The cold side is at 302 K for the three temperature gradients and the hot side is at 322 K, 332 K and 342 K for the gradients of 20 K, 30 K and 40 K, respectively. (c) Photograph of a TEG module with 3 serial stacks. (d) The voltage generated by TEG stacks connected in series as a function of the number of stacks
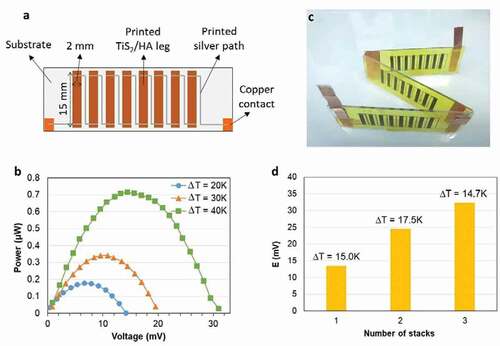
Finally, the flexibility and durability tests on TiS2/HA legs printed on a Kapton ® tape were carried out. The experimental results showing a very good bending resistance and durability are presented in Supporting Information (Note 7 and Figure S11).
4. Conclusions
A fabrication process of hybrid polycrystalline TiS2/hexylamine thin films that lead to average power factors of 1100–1460 µW m−1 K−2 in the 330 K-430 K temperature range has been described. These values exceed the reported performance for hybrid thin films based not only on polycrystalline but also single crystal TiS2. It has been shown that these properties are obtained thanks to the proper selection of size of the exfoliated 2D nanoflakes and a better organization of the crystal in the final layered hybrid material as well as high intercalation levels of hexylamine into TiS2. They are furthermore strongly influenced by the process parameters such as the quality and particle size of the TiS2 powder and the length of the amine chain. In addition, versatile inks were developed which can be directly used in printing processes. Prototype TEG stacks and modules based on n-type printed films were prepared. An outstanding maximum power output of 0.713 µW and power density of 16.0 W m−2 for a temperature gradient of 40 K was generated by an individual stack made of eight printed legs connected in series. It is a major achievement to demonstrate that properties even better than those of the corresponding single crystal can be obtained by printing techniques. Such a performance may be useful for the low-power applications e.g. LPWAN that are foreseen for the development of the internet-of-everything.
Author contributions
S.J., B.D. and R.C. designed the research. S.J., B.D., D.P., Z.U.K. and R.C. performed the experiments. All the authors discussed the results. S.J., M.J.L., X.C and R.C. wrote the manuscript.
Received: ((will be filled in by the editorial staff))
Revised: ((will be filled in by the editorial staff))
Published online: ((will be filled in by the editorial staff))
Supplemental Material
Download MS Word (1.1 MB)Acknowledgments
We thank Dr. Vincent Linseis from Linseis Messgeraete GmbH for the thermal conductivity measurements of the thin films using the Thin Film Analyzer (TFA). Research in Sweden is funded by the Knut and Alice Wallenberg Foundation (Tail of the Sun) and the Support from the Swedish Government Strategic Research Areas in Materials Science on Functional Materials at Linköping University (Faculty Grant SFO-Mat-LiU No. 2009-00971). Z.U.K. would like to acknowledge Venteskapsrådet’s grant #2016-00479.
Disclosure statement
We declare that none of the authors have competing financial or non-financial interests.
Supplemental Material
Supplemental data for this article can be accessed here.
Additional information
Funding
References
- Bahk JH, Fang H, Yazawa K, et al. Flexible thermoelectric materials and device optimization for wearable energy harvesting. J Mater Chem C. 2015;3:10362–10374.
- Chen B, Kruse M, Xu B, et al. Flexible thermoelectric generators with inkjet-printed bismuth telluride nanowires and liquid metal contacts. Nanoscale. 2019;11:5222–5230.
- Wang Y, Shi Y, Mei D, et al Wearable thermoelectric generator to harvest body heat for powering a miniaturized accelerometer. Appl Energy. 2018;215:690–698.
- Liu Z, Chen G. Advancing flexible thermoelectric devices with polymer composites. Adv Mater Technol. 2020;5:2000049.
- Lv H, Lian L, Zhang Y, et al. A flexible spring-shaped architecture with optimized thermal design for wearable thermoelectric energy harvesting. Nano Energy. 2021;88:106260.
- Qu D, Huang X, Li X, et al Annular flexible thermoelectric devices with integrated-module architecture. NPJ Flex Electron. 2020;4:1.
- Li Z, Deng L, Lv H, et al. Mechanically robust and flexible films of ionic liquid-modulated polymer thermoelectric composites. Adv Funct Mater. 2021;31:2104836.
- Raj A, Steingart D. Review—Power sources for the internet of things. J Electrochem Soc. 2018;165(8):B3130–B3136.
- Miraz MH, Ali M, Excell PS, et al Internet of nano-things, things and everything: future growth trends. Future Internet. 2018;10:68.
- Zhao D, Martinelli A, Willfahrt A, et al. Polymer gels with tunable ionic Seebeck coefficient for ultra-sensitive printed thermopiles. Nat Commun. 2019;10:1093.
- Luo J, Billep D, Blaudeck T, et al Chemical post-treatment and thermoelectric properties of poly(3,4-ethylenedioxylthiophene):poly(styrenesulfonate) thin films. J Appl Phys. 2014;115:054908.
- Zheng Y, Zeng H, Zhu Q, et al. Recent advances in conducting poly-(3,4-ethylenedioxythiophene):polystyrene sulfonate hybrids for thermoelectric applications. J Mater Chem C. 2018;6:8858.
- Kim GH, Shao L, Zhang K, et al. Engineered doping of organic semiconductors for enhanced thermoelectric efficiency. Nat Mater. 2013;12:719–723.
- Bubnova O, Khan ZU, Wang H, et al. Semi-metallic polymers. Nat Mater. 2014;13:190–194.
- Liu J, Wang X, Li D, et al Thermal conductivity and elastic constants of PEDOT:PSS with high electrical conductivity. Macromolecules. 2015;48(3):585–591.
- Markov M, Zebarjadi M. Thermoelectric transport in graphene and 2D layered materials. Nanoscale Microscale Thermophys Eng. 2019;23(2):117–127.
- Fugallo G, Cepellotti A, Paulatto L, et al Thermal conductivity of graphene and graphite: collective excitations and mean free paths. Nano Lett. 2014;14(11):6109–6114.
- Chmielowski R, Bhattacharya S, Xie W, et al. High thermoelectric performance of tellurium doped paracostibite. J Mater Chem C. 2016;4:3094–3100.
- Beaumale M, Barbier T, Breard Y, et al. Electron doping and phonon scattering in Ti1+xS2 thermoelectric compounds. Acta Mater. 2014;78:86–92.
- Imai H, Shimakawa KY. Large thermoelectric power factor in TiS2 crystal with near stoichiometric composition. Phys Rev B. 2001;64:241104(R).
- Qu D, Li X, Wang H, et al. Assembly strategy and performance evaluation of flexible thermoelectric devices. Adv Sci. 2019;6:1900584.
- Wan C, Gu X, Dang F, et al. Flexible n-type thermoelectric materials by organic intercalation of layered transition metal dichalcogenide TiS2. Nat Mater. 2015;14:622–627.
- Bhattacharya S, Gunda NSH, Stern R, et al. Achieving optimum carrier concentrations in p-doped SnS thermoelectrics. Phys Chem Chem Phys. 2015;17:9161–9166.
- Bubnova O, Khan ZU, Malti A, et al. Optimization of the thermoelectric figure of merit in the conducting polymer poly(3,4-ethylenedioxythiophene). Nat Mater. 2011;10:429–433.
- Wan C, Tian R, Kondou M, et al. Ultrahigh thermoelectric power factor in flexible hybrid inorganic-organic superlattice. Nat Commun. 2017;8:1024.
- Tian R, Wan C, Wang Y, et al. A solution-processed TiS2/organic hybrid superlattice film towards flexible thermoelectric devices. J Mater Chem A. 2017;5:564.
- Wan C, Tian R, Azizi AB, et al. Flexible thermoelectric foil for wearable energy harvesting. Nano Energy. 2016;30:840–845.
- Ferhat S, Domain C, Vidal J, et al. Flexible thermoelectric device based on TiS2(HA)x n-type nanocomposite printed on paper. Org Electron. 2019;68:256–263.
- Aslan SM, Gall A, Lemmer U, et al. Screen print-capable titanium disulfide for use in a thermoelectric generator. European patent EP 3587507. 2018 Aug 22.
- Chmielowski R, Delatouche B, Jacob S, et al. Method for preparing titanium sulfide/amine thin films and thin films obtained. FR 3087764. 2018 Oct 26.
- Wang L, Zhang Z, Geng L, et al. Solution-printable fullerene/TiS2 organic/inorganic hybrids for high-performance flexible n-type thermoelectrics. Energy Environ Sci. 2018;11:1307–1317.
- Sofer Z, Sedmidubský D, Luxa J, et al Universal method for large-scale synthesis of layered transition metal dichalcogenides. Chem Eur J. 2017;23(42):10177–10186.
- Riekel C, Schöllhorn R. Structure refinement of nonstoichiometric TiS2. Mater Res Bull. 1975;10(7):629–633.
- Linseis V, Völklein F, Reith H, et al. Advanced platform for the in-plane ZT measurement of thin films. Rev Sci Instrum. 2018;89:015110.
- Weiss A, Ruthardt R. Layer-intercalation-compounds of Titaniumdisulfide with n-Alkylamines. Z Naturforsch B. 1973;28(5–6):249–251.
- Gu Y, Song K, Hu X, et al Realization of an ultrahigh power pactor and enhanced thermoelectric performance in TiS2 via microstructural texture engineering. ASC Appl Mater Interfaces. 2020;12(37):41687–41695.
- Klipstein PC, Bagnall AG, Liang WY, et al. Stoichiometry dependence of the transport properties of TiS2. J Phys C. 1981;14:4067–4081.
- Stoliaroff A, Latouche C, Jobic S. Versatile electrical behavior of 1T-TiS2 elucidated from a theoretical study. Phys Rev B. 2019;99:165122.
- Thompson AH, Gamble FR, Symon CR. The verification of the existence of TiS2. Mat Res Bull. 1975;10(9):915–919.
- Coleman JN, Lotya M, O’Neill A, et al. Two-dimensional nanosheets produced by liquid exfoliation of layered materials. Science. 2011;331:568–571.
- Sherrell PC, Sharda K, Grotta C, et al. Thickness-dependent characterization of chemically exfoliated TiS2 nanosheets. ACS Omega. 2018;3:8655–8662.
- Cucinotta CS, Dolui K, Pettersson H, et al hic properties and chemical reactivity of TiS2 nanoflakes. J Phys Chem C. 2015;119(27):15707–15715.
- Haxhibeqiri J, De Poorter E, Moerman I, et al. A survey of LoRaWAN for IoT: from technology to application. Sensors. 2018;18:3995.