ABSTRACT
Formaldehyde is a well-known industrial material regularly used in fishery, vegetable markets, and fruit shops for maintaining their freshness. But due to its carcinogenic nature and other toxic effects, it is very important to detect it in very low concentrations. In recent years, amine-containing fluorescent probes have gained significant attention for designing formaldehyde sensors. However, the major drawbacks of these small molecular probes are low sensitivity and long exposure time, which limits their real-life applications. In this regard, polymeric probes have gained significant attention to overcome the aforementioned problems. Several polymeric probes have been utilized as a coating material, nanoparticle, quartz crystal microbalance (QCM), etc., for the selective and sensitive detection of formaldehyde. The main objective of this review article is to comprehensively describe the recent advancements in formaldehyde sensors based on small molecules and polymers, and their successful applications in various fields, especially in situ formaldehyde sensing in biological systems.
1. Introduction
Formaldehyde (FA, HCHO), is the simplest aldehyde, and its 40% aqueous solution is known as formalin. It is a colorless, poisonous gas with a strong smell, that causes severe damage to our central nervous system (CNS) and immune system [Citation1]. It is generally used in the making of many resins, polymers, wood products [Citation2], and protein cross-linkers for tissue fixation [Citation3]. The thermal and chemical decomposition of construction materials such as particleboard and urea-formaldehyde foam insulation heavily release FA in the atmosphere [Citation1]. FA is well known for its toxicity and carcinogenic nature [Citation4]. In humans, formaldehyde transforms into formic acid, giving rise to breathing difficulties, hypothermia, blood acidity, and coma or death [Citation5]. Recently, International Agency for Research on Cancer (IARC) has announced formaldehyde as one of the main Group 1 carcinogenic organic compounds in the World [Citation6]. The World Health Organization (WHO) declared the limit of exposure to formaldehyde as 80 parts per billion (ppb) as standard for 30 min [Citation7]. Occupational Safety and Health Administration (OSHA) fixed standard value for permissible exposure limit at 750 ppb, while maximum exposure value for Immediately Dangerous to Life or Health (IDLH) is considered as 20 parts per million (ppm) [Citation8,Citation9]. US Environmental Protection Agency has determined the maximum reference dosage of FA is 0.15–0.2 mg/kg body weight/day [Citation10]. In the brains of healthy individuals, the concentration of FA is in the 0.2–0.4 mM range [Citation11]. In body tissues, FA reacts with biomolecules like amino acids, proteins, nucleic acids and nucleotides, and produces unstable assemblies which transport FA to distant tissues from the respiratory tract [Citation12]. Consumed FA causes damage in liver and kidney (), which leads to albuminuria, jaundice, haematuria and acidosis, anuria, or inactivity in central nervous system leading to depression, and even causing heart failure [Citation5,Citation13]. Even a low level of FA harms the respiratory organs, nose, eyes, and originates allergies, commonly identified as sick house syndrome [Citation14]. Ingestion of 30 mL of 37% HCHO solution is reported to cause death in humans [Citation15]. Moreover, FA is toxic to neuronal cells and is able to disrupt the neuronal networks through τ protein polymerization and induction of hyperphosphorylation [Citation16].
Due to the fact that it is highly dangerous for mankind and nature, it has become very important to detect formaldehyde at a low concentration level. Nowadays many techniques are used to detect it efficiently, such as Raman spectroscopy [Citation17], liquid chromatography [Citation18], gas chromatography [Citation19], sol-gel methods [Citation20], transmission electron microscopy (TEM) [Citation21], conductometry [Citation22,Citation23], biosensor methods [Citation24], and ion chromatography [Citation25]. These techniques are highly sensitive, but not suitable for the detection of FA in living cells. In contrast, fluorescent probes have attracted significant attention from researchers due to their facile detection along with their highly sensitive nature. Consequently, in recent years researchers have developed several efficient fluorescent sensors for FA by taking the benefit of the aza-Cope rearrangement [Citation26,Citation27] or formaldehyde amine condensation [Citation28,Citation29]. Yet, the probes used for detection usually take a long response time due to the slow reaction between the probe and formaldehyde. Most of the reported probes are usually water-insoluble, thus some amount of volatile organic solvents is added into the aqueous media, which restricts their biological application. Also, the probes are not biodegradable and hence there remains a challenge of excretion from our body. In this regard, polymeric probes have emerged as an optimal solution to the above-mentioned problems.
Polymeric probes are being used in several sensing [Citation30,Citation31] and real-life applications to overcome the problems faced by small molecular probes. Recently, our group has published several articles on polymeric sensors [Citation32,Citation33] for the selective detection of metals [Citation34,Citation35] and volatile organic compounds [Citation36,Citation37]. Thus, hydrophilic fluorescent polymeric sensors with pendant primary amine groups have emerged as an alternative approach for FA sensing as they offer improved water solubility and high sensitivity. Various functional moieties are generally incorporated into the side chain or polymeric backbone to design polymeric sensing materials [Citation38,Citation39]. In this regard, polymeric probes are considered as an outstanding platform due to their multiple recognition sites that can bind with the lower concentration of analyte more effectively, and result in faster fluorescence response as well as desirable signal amplification [Citation40,Citation41]. Even though various polymeric sensors have been successfully developed for numerous environmental or food pollutants, very less effort has been devoted to fabricate water-soluble, fast-response polymeric materials for sensing formaldehyde [Citation42,Citation43]. Therefore, a detailed investigation in this regard is necessary to obtain a thorough understanding to design effective fast-response polymeric sensors having good aqueous solubility. Although some review articles have been published [Citation44,Citation45] focusing on the small molecular probes, to the best of our knowledge, there is no review article accentuating the importance of polymeric materials in FA sensing. In this review article, our major aim is to comprehensively review different types of reaction-based techniques for FA sensing, and their successful applications in various fields, especially in situ formaldehyde sensing.
2. Detection of FA using small molecule-based sensors
Fluorescent imaging has become a crucial part of recent scientific discoveries due to its ability to observe changes inside the cells, and tissues. Consequently, scientists have used this method of fluorescence detection for rapid and accurate detection of FA. According to recent research, in most cases of formaldehyde-detecting fluorescent probes, the amino group of the probe and the carbonyl group of HCHO generally react to detect formaldehyde via colorimetric changes. The detection-based reactions are generally categorized into two classes: (1) formimine type of intermediate forms from the reaction between FA and primary amine of probes, this consists of three types (i) 2-aza-Cope rearrangement [Citation46,Citation47] and subsequent β-elimination from the probe [Citation48,Citation49]; (ii) Schiff base-based probe [Citation50,Citation51]; (iii) aromatic hydrazine [Citation52,Citation53], and (2) aminal formation via reaction of FA and o-diamino probes [Citation54,Citation55]. Using these reactions and by their functional modifications, FA sensing mechanism can be attained via different processes, like photoinduced electron transfer (PET) [Citation56,Citation57], spiro cyclization [Citation58], intramolecular charge transfer (ICT) [Citation59], and also different response mechanisms such as, ratiometric [Citation60] and fluorophore turn-on-based detection [Citation61]. Also, many other types of reactions such as cyclization [Citation62], ring-opening reaction [Citation63], and nucleophilic addition [Citation64] are used to detect FA.
2.1. 2-Aza-Cope rearrangement-based fluorescent probe
Aza-Cope rearrangement reaction is currently used as a successful method to detect FA [Citation26]. If an amine group, more specifically a homoallylic one bearing a fluorescent probe, reacts with formaldehyde, 2-aza-Cope sigmatropic rearrangement reaction takes place. As per ), the amine group of the probe reacts with FA, and iminium intermediate is formed, which then undergoes 2-aza-Cope sigmatropic rearrangement reaction, which gives rise to a fluorescent product after hydrolysis.
Figure 2. Schematic representation of 2-aza-Cope rearrangement reaction and chemical structures of probes 1, 2, and 3.
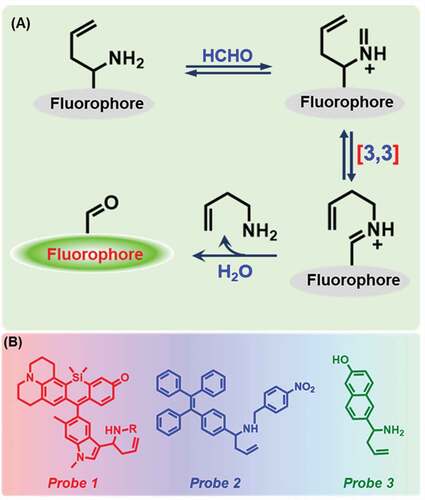
In 2015, Roth et al. [Citation61] had first used this method to detect formaldehyde. They reported a silicon rhodol julolidine-based fluorescent Probe 1 ()) to detect FA using donor-excited photoinduced electron transfer (d-PET) process [Citation65] in neuro screen-1 and living HEK293TN cells. The detection limit for FA was measured as 0.01 mM () in phosphate buffer solution (PBS, pH 7.4), and observed a 3-fold fluorescent increase (excitation: 633 nm) upon the addition of 5 mM FA. Later, Zhao et al. [Citation66] have synthesized a solid portable sensor, Probe 2, based on aggregation-induced emission (AIE) fluorophore (tetraphenylethylene), for FA detection through fluorescence ‘turn-on’ response ()). The probe exhibited high selectivity and sensitivity for gaseous FA within 60 min. The sensing limit of the FA test plate was quantified to be 0.036 mg/m3 (). A ratiometric fluorescent FA sensor consisting of 6‐hydroxy naphthalene chromophore (Probe 3) was developed by Lin and co-workers ()) [Citation67]. After reaction with an increasing concentration of FA, the emission peak at 451 nm was gradually increased while the peak at 359 nm decreased. Additionally, the probe showed an ability of FA sensing in HeLa cells ().
Table 1. Sensing properties of small molecule-based probes for FA detection
2.2. Aromatic amine (Schiff base)-based fluorescent probe
Schiff bases of the general formula RCH = NR are made by a simple condensation reaction between amine and aldehyde ()). These reactions are precisely used for generating C-N bonds in organic chemistry with a very high yield [Citation68]. Schiff bases are used as one of the most suitable and useful ligands for the detection of metal ions and some organic molecules due to their strong coordination capability [Citation69,Citation70].
Figure 3. (a) Schematic representation of Schiff base formation reaction, and (b) Chemical structure of different probes.
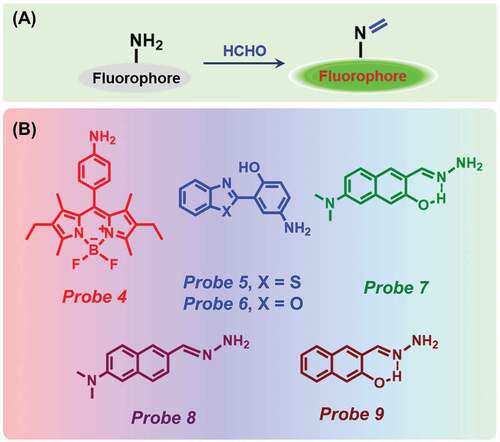
In 2012, Song et al. have used this approach for the first time to selectively detect FA [Citation71]. The difluoroborondipyrromethene (BODIPY)-based Probe 4 ()) showed a very weak fluorescent peak at 535 nm due to an active PET quenching effect. The effect was quickly demolished after the addition of an increasing amount of FA due to the formation of an imine compound. The detection limit of their probe was estimated as 165 nM (). Exploiting this method, Wu and co-workers in 2018 have prepared two fluorescent probes composed of benzothiazole (Probe 5) and benzoxazole (Probe 6) ()) unit for the detection of FA utilizing excited state intramolecular proton transfer (ESIPT) method [Citation72]. Probe 5 exhibited two absorption bands at 300 and 365 nm. After the addition of FA, the peak intensity at 365 nm decreased and a 5 nm blue shift was observed. In addition, a significant increase in fluorescence intensity at 519 nm was noticed with a blue shift at 476 nm attributed to the inhibition of PET process between benzothiazole and amino group. The fluorescence quantum yield of the probe was also increased from 0.098 to 0.156. Similarly, for Probe 6, the addition of FA resulted in an increase of fluorescence intensity at 486 nm along with an enhancement of quantum yield from 0.085 to 0.089. The limit of detection (LOD) of Probes 5 and 6 were determined as 2 and 29 µM, respectively (). The probes rapidly detected FA in less than 3 min. Besides, test strips were prepared using Probe 5 for quick naked-eye detection of FA. The imaging of FA was successfully achieved in arabidopsis thaliana tissues and MCF-7 cells utilizing Probe 5.
In a recent work, Chen et al. have fabricated three different naphthalene derivatives (Probes 7, 8 and 9) as FA sensors ()) [Citation73]. Among them, only Probe 7 showed high sensitivity and selectivity for FA due to the presence of both ESIPT and ICT effects. After adding FA to the solution of Probe 7 (PBS buffer solution containing 1% DMSO, pH 7.4), a 14-fold enhancement of emission intensity at 510 nm was observed. The fluorescence enhancement reached a plateau in 100 min upon consecutive addition of 100 μM of FA. The detection limit of Probe 7 for FA was estimated as 0.35 µM (). On the other hand, Probe 8 displayed less sensitivity toward FA, and in the case of Probe 9, no change in fluorescence was observed in the presence of FA due to the absence of ICT. Moreover, Probe 7 was effectively used for successful fluorescence imaging of exogenous and endogenous FA in living cells.
2.3. Hydrazine-based fluorescent probe
In the past few years, several fluorescent probes with hydrazine moiety were developed for selective detection of FA. Hydrazine moiety with an amino group has very strong nucleophilicity which showed high reactivity for FA. Upon reaction with FA, it forms active methylene hydrazine which restricts the PET process from the hydrazine to FA ()).
Figure 4. (a) Schematic representation of reaction between hydrazine-based probe with FA and (b) Different sensors for the detection of FA.
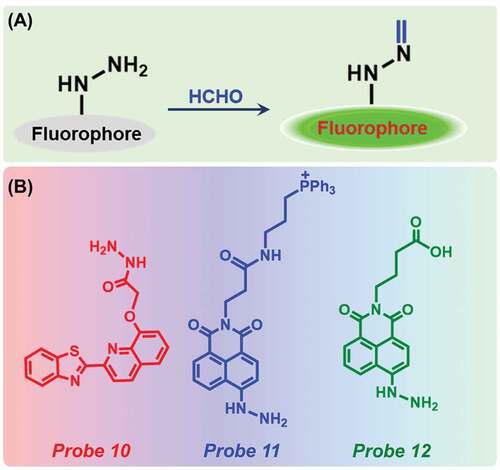
Liu et al. have first prepared a hydrazine moiety-based probe 10 ()) for FA detection [Citation74]. The probe had very weak fluorescence at 467 nm due to PET process from imine to the quinoline fluorophore, however, after adding FA, the fluorescence intensity increased by 5.5-fold due to the elimination of PET effect. The probe showed high selectivity in the presence of several other organic compounds, such as acetone, other aldehydes, formic acid, and acetic acid. The detection limit of the probe was determined as 900 nM (). The probe was able to successfully detect FA in several food samples. Nanofibers were fabricated by blending the probe with poly(vinyl alcohol) followed by electrospinning. Moreover, upon immersion of the nanofibers into water containing FA, a fluorescence increment was noticed. Later, in 2018, Xu et al. established a two-photon fluorescent probe (Probe 11, )) based on hydrazine moiety conjugated 1,8-naphthalimide [Citation75]. Upon addition of FA, the probe displayed a turn-on fluorescence at 539 nm as the condensation reaction of the probe and FA hindered the PET process and the fluorescence intensity reached an equilibrium state at 40 min. The detection limit of Probe 11 was determined to be 4.9 × 10−6 M (). Furthermore, the highly selective probe could be successfully utilized for FA imaging in living mice liver tissues and HeLa cells.
Another naphthalimide-based probe (Probe 12, )) was also reported by Yuan and co-workers for the extremely selective and sensitive detection of FA [Citation76]. After the addition of FA to this probe, a blue shift from 449 nm to 435 nm in absorption spectra occurred along with a color change from dark red to bright yellow. The probe by itself displayed very weak fluorescence, however, a substantial increase in fluorescence intensity at 519 nm was observed after the addition of FA, due to the inhibition of PET process. With increasing concentration of FA from 0 to 100 mM, the peak intensity increased linearly. The detection limit was determined as 0.36 µM (). No changes observed in fluorescence intensity in the presence of several aldehydes (benzaldehyde, p-chlorobenzaldehyde, oxalaldehyde, 2,4-dihydroxybenzaldehyde, p-hydroxylbenzaldehyde, etc.), metal ions (K+, Ca2+, Ba2+, Na+, Mg2+, etc.), or other compounds (cysteine, glutathione, homocysteine), indicated high selectivity of the probe. Further, the probe was employed for endogenous FA imaging in MCF-7 cells.
2.4. FA detection via aminal formation
An aminal group is a type of organic compound, having two amine groups attached to the same carbon atom. It has a general chemical formula -C(NR2)(NR2)-. When FA reacted with this diamine moiety, a condensation reaction took place and an imino intermediate was formed. This intermediate consequently opens the intramolecular deoxylactam, thus forming a strongly fluorescent ring-opened imidazole product ()).
Figure 5. (a) Schematic representation of aminal moiety-based detection technique and (b) probes used for FA detection.
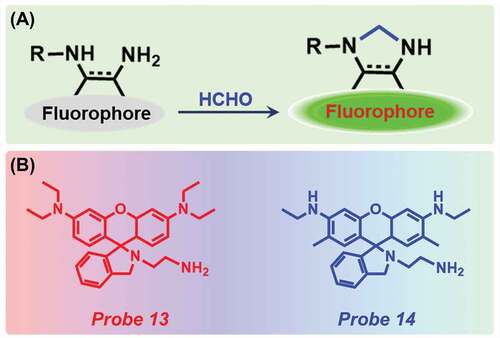
A rhodamine-based fluorescent probe, N-(rhodamine B)-deoxylactam-ethylenediamine (dRBEDA) (Probe 13, )) was synthesized by Li et al. for the detection of several aldehydes via the formation of aminal intermediate [Citation54]. Interestingly, only with FA, an increase in fluorescence intensity at 560 nm was noticed after 90 min along with the development of red color. The detection limit of the probe was quantified to be 3.5 mM (). The sensor was capable of labelling cell-surface aldehyde-exhibiting sialoproteins with high selectivity. Later, in 2016, He and co-workers prepared a rhodamine 6 G-based sensor (Probe 14) for the detection of FA ()) [Citation55]. The probe was completely nonfluorescent but with the addition of increasing concentrations of FA, the emission peak at 560 nm gradually increased and reached to maximum when 1.0 equivalent FA was present and a color change from colorless to pink was observed. After exciting with 365 nm UV light, naked-eye detection was noticed. The detection limit was estimated as 7.7 × 10−7 M (). To determine the selectivity, the probe was reacted with several other aldehydes, amino acids, glucose but no change was observed. Interestingly, the probe works better in the basic medium than the acidic one. The authors successfully applied the probe for rapid detection of FA in dried shiitake mushrooms.
3. Detection of FA using polymeric probes
Most of the small molecular probes are less soluble in aqueous medium which limits their biological applications. There is a need for polymeric sensors with good water solubility, high biocompatibility, low detection limit, and rapid sensing ability. Recently, a lot of organic polymeric probes have been used in the form of coating material, nanoparticles, QCM for the detection of FA.
In 2010, Feng and co-workers reported the first simple yet sensitive formaldehyde detection technique using an amine-terminated polymer Probe 15 () [Citation77]. They used regular pH indicators in a polymer film doped into an amine-terminated poly(ethylene glycol) (PEG) polymer which detects FA concentrations of 20 ppm to 250 ppb in less than 1 min and almost 50 ppb of FA within 10 min. Upon interaction of the amine groups present in the polymeric probe with FA, an alteration of pH occurred due to which the indicators showed a colorimetric change. Whereas for other aldehydes almost negligible changes were observed even after exposure for 5 min. This was the first report where FA was detected via naked eye.
Table 2. Sensing properties of polymeric probes for FA detection
In recent years, water-soluble supramolecular polymers have gained significant attention due to their potential biomedical applications. Looking into these aspects for selective detection of FA, Fan and co-workers have reported a water-soluble supramolecular polymer (Probe 16, )) using a compound as N1,N3,N5-tri(pyridin-4-yl)benzene-1,3,5-tricarboxamide (DTA) [Citation78] (). The addition of FA solution into DTA resulted in supramolecular self-assembly in water through hydrogen bonding interaction ()). When several aldehydes were added to DTA, only in the case of FA, the state of DTA changed to a colorless solution and a light blue fluorescence was observed under UV light (λem = 470 nm) ()). Moreover, a morphological change to fiber structure was noticed after FA addition as confirmed by Scanning Electron Microscopy (SEM) analysis ()). The LOD of DTA for FA was calculated to be 1.79 × 10−8 M.
Figure 6. (a) Proposed recognition mechanism of DTA for monitoring FA, (b) photographs of the DTA after addition of FA, and (c) SEM image of DTA before and after FA addition. Reused with permission from ref [Citation78]. Copyright 2019 Royal Society of Chemistry.
![Figure 6. (a) Proposed recognition mechanism of DTA for monitoring FA, (b) photographs of the DTA after addition of FA, and (c) SEM image of DTA before and after FA addition. Reused with permission from ref [Citation78]. Copyright 2019 Royal Society of Chemistry.](/cms/asset/e2720a7c-f85a-4849-ad2b-761b8e4e33d0/tsta_a_2018920_f0006_oc.jpg)
Li et al. have reported a naphthalimide functionalized chitosan-based polymeric molecule Probe 17 ()), where the hydrazine group reacts with FA to generate a turn-on fluorescence [Citation79]. Upon addition of FA, probe 17 showed a colorimetric (colorless to yellow) and fluorometric change (non-emissive to bright yellow) ()) due to PET process from pendant hydrazine units to naphthalimide fluorophores. Probe 17 rapidly interacted with FA and exhibited a fluorometric alteration within 1 min whereas its small molecular analogue took 30 min to exhibit any notable change. With increasing FA concentration, the emission peak intensities of aqueous solution of Probe 17 gradually increased. The end-to-end hydroxyl group present in the probe enhanced the FA concentration around the polymer chain. In acidic pH range from 1 to 6.5, an improved fluorescence was noticed as acid catalyzed the reaction between FA and naphthalimide groups. The detection limit of the sensor was evaluated to be around 0.05 ppm (1.66 µM) and emission intensity variation was detected with only 1 ppm of FA which further confirmed its sensitivity. Moreover, they successfully applied this sensor to detect HCHO in tap water and three other commercially available food samples as chicken, bream, and pork.
Figure 7. (a) Structure of chitosan-based polymeric sensor. (b) Colorimetric and (c) Fluorometric change of the probe in the presence of 100 ppm FA. Reused with permission from ref [Citation79]. Copyright 2018 American Chemical Society.
![Figure 7. (a) Structure of chitosan-based polymeric sensor. (b) Colorimetric and (c) Fluorometric change of the probe in the presence of 100 ppm FA. Reused with permission from ref [Citation79]. Copyright 2018 American Chemical Society.](/cms/asset/35bd6845-4132-4377-8073-079de68cac9c/tsta_a_2018920_f0007_oc.jpg)
A graphene/poly(methyl methacrylate) (PMMA)-based sensor [Citation80], Probe 18, was developed by Alizadeh and co-workers for the detection of FA. The device was extremely selective for FA and could sense FA vapor at a precisely small amount and comparatively extensive range of concentration (0.05 and 5.0 ppm). Direct interaction of graphene surface and FA vapor was the main reason for sensor response. The polymeric material acted as an adhesive material when cast on the film. PMMA with more hydrophobic nature effectively deters H2O from the polymer surface and therefore from the graphene sheet surface which cuts the sensor response to water molecules. However, FA, which has an additional hydrophobic interaction capability compared to water molecules, can successfully pass over the polymer repulsion wall and result in a signal in the graphene sheet. The limit of detection of this material was calculated to be 10 ppb ().
Sayed and co-workers used a thiol responsive functionalized silica nanoparticle, Probe 19 for the selective colorimetric sensing of FA ()) [Citation81]. In the probe, the thiol groups interacted with squaraine dye which resulted in a loss of π-conjugation property of the chromophore. While, in the presence of FA, this reaction was inhibited and a chromogenic change was observed. Selective response to FA was observed only when both the thiol and polyamine groups were attached to the silica surface. After reaction with FA, a significant enhancement of absorbance at 642 nm was observed along with a colorimetric change from colorless to cyan. The bulky polyamine groups created a highly polar environment around thiols, which only reacted with small and polar FA. Due to the bulkiness of other aldehydes, no change was observed. The LOD for FA was calculated to be 36 ppb in water ().
Figure 8. The sensing mechanism of FA using Probe 19. Reused with permission from ref [Citation81]. Copyright 2016 American Chemical Society.
![Figure 8. The sensing mechanism of FA using Probe 19. Reused with permission from ref [Citation81]. Copyright 2016 American Chemical Society.](/cms/asset/0b2ceb83-6de5-4507-bdaf-d886813de9fe/tsta_a_2018920_f0008_oc.jpg)
Antwi-Boampong and BelBruno have established a polyethyleneimine/polyaniline (PEI/PANi) composite film Probe 20 prepared by spin casting for FA detection [Citation82]. The electrophile carbon atom of FA interacts with the nonbonding electrons on the primary amine functionalities of PEI to reversibly form a polymer bound adduct which is capable of extracting a proton from the doped PANi present in the PEI/PANi composite film. Moreover, chemical interaction with PEI traps FA in the film and increased the probability of interaction with the doped PANi. The film was presented to be receptive to the amount of FA existing in the air. The reaction with FA was expressively better than the response to water vapor, ammonia, or the other volatile organic compounds tested. These results suggest that it is indeed a good detector for real-time FA exposure in atmosphere.
In 2017, Tang and co-workers prepared a sensor Probe 21 with the stability of more than one year for FA detection [Citation83]. A polypyrrole-based molecular imprinted polymer (PPy-based MIP) was combined with a titanium dioxide nanotube (TiO2-NTA) to improve the bonding ability of the PPy-based MIP coating. After exposing it to FA, it precisely binds the FA molecules by the hydrogen bond interactions. The attached FA molecules contribute electrons to the PPy-based MIP sheet. The conjugation effect and the swelling effect of the PPy-based MIP layer also accelerate the electron transfer process and increase the conductance. The voids printed on the PPy-based MIP sheet can differentiate FA from other gases by molecular size and monomer functionalities. Apart from that, the sensor shows a decent selectivity for FA than acetic acid, acetaldehyde, acetone, and ethanol, and also very good immunity to humidity. The sensor has a good sensitivity of 13% for 1 ppm FA at room temperature ().
Iqbal et al. used assemblage of imprinted PMMA and core-shell gold nanoparticles assemblage in layer-by-layer for producing imp-PMAA/Au-NPs hybrid Probe 22 for the sensing study [Citation84]. The procedure for the synthesis of low-temperature imprinted-PMAA is shown in . Quartz microbalance (QMB) coated with imp-PMAA/Au-NPs hybrid layer (optimized thickness: 100 ± 20 nm) is used as a transducer. The sensor shows very high sensitivity due to non-covalent dispersion interactions of FA with the molecular recognition sites and enhanced surface area. The sensor has a very low detection limit of 152 ppb and fast response and recovery times, 28 and 13 sec, respectively. Due to its minimal humidity effect, the sensor was used for monitoring indoor air quality.
Figure 9. A schematic representation of the low-temperature synthesis of formaldehyde imprinted poly(methacrylic acid) (imp-PMAA). Reused with permission from ref [Citation84]. Copyright 2014 Royal Society of Chemistry.
![Figure 9. A schematic representation of the low-temperature synthesis of formaldehyde imprinted poly(methacrylic acid) (imp-PMAA). Reused with permission from ref [Citation84]. Copyright 2014 Royal Society of Chemistry.](/cms/asset/8c735e5c-4619-4b72-b52c-7a01945c5d51/tsta_a_2018920_f0009_oc.jpg)
Another molecularly imprinted polymer (MIP), Probe 23, was developed by Hussain and co-workers for sensing FA vapors in air [Citation85]. A QCM conjugated with copolymer thin film containing ethylene glycol dimethacrylate, methacrylic acid, and styrene, was prepared. Astonishingly, these MIPs indicated explicit behavior for different Volatile Organic Compounds (VOCs), such as methanol, acetaldehyde, dichloromethane, and formic acid. Although being an apt receptor in principle, the MIPs were not beneficial for amounts at 50% moisture due to surface saturation by water. This was compensated by changing the morphology from thin film to nanoparticles by introducing different primary amino groups into the polymer via allylamine. It had a detection limit of 500 ppm ().
In another study, ethylenediamine (EDA)-functionalized poly(ionic liquid)/polyacrylonitrile nanofibrous membrane (PIL/PAN NFM), Probe 24, was synthesized as a detector [Citation86]. The poly(ionic liquid) backbones in the ionic microchannels significantly improved electron transportation, thus helped in the detection of FA in aqueous solution by amplifying the current signals. Poly(1-butyl-3-vinyl imidazolium bis(trifluoromethanesulfonyl)imide) ([PBVIm][TFSI]) was used for construction of ionized matrixes and polyacrylonitrile (PAN) for electrospinning. The detection strength was determined as ranging from 360 ppm (3.6 × 102 mg/L) to 0.036 ppt (3.6 × 10−11 mg/L) (). Additionally, the intended ionic microchannels were used for FA detection in real water samples.
Liu and co-workers have prepared β-ketone ester-containing polymers (Probes 25 and 26) by radical polymerization of 2-(acetoacetoxy)ethyl methacrylate (AEMA) and poly(ethylene glycol methyl ether) methacrylate (PEGMA) in different ratios (for Probe 25 AEMA:PEGMA = 1:1; for Probe 26 AEMA:PEGMA = 1:2) for FA detection via Hantzsch reaction ()) in living systems [Citation87]. Upon incubation of the polymers and their control small molecules with 5 mM FA at 20 C, a strong fluorescence at 460 nm was observed after 5 min in the case of polymers, whereas, even after 30 min the control small molecules had only very weak fluorescence at 510 nm. For the polymeric probes (Probes 25 and 26), the fluorescent enhancements after 5 min were by 260 and 110 folds respectively, but the control probes had only 21- and 37-folds increase, which proves the significantly high sensitivity of the polymeric probes. The detection limits of Probes 25 and 26 were calculated to be 3.1 × 10−7 and 3.4 × 10−7 M, respectively (). Moreover, the polymeric probes successfully detected FA in living cells.
Figure 10. (a) Hantzsch Reaction and (b) the reaction of the polymeric probe with FA. Reused with permission from ref [Citation87]. Copyright 2018 American Chemical Society.
![Figure 10. (a) Hantzsch Reaction and (b) the reaction of the polymeric probe with FA. Reused with permission from ref [Citation87]. Copyright 2018 American Chemical Society.](/cms/asset/44dc18e3-8a9c-4f5e-a710-53b4526985d3/tsta_a_2018920_f0010_oc.jpg)
In 2011, Ding and co-workers reported polyethyleneimine (PEI)-functionalized polyamide 6 (PEI-Pa 6) nano-fiber/net (NFN) (Probe 27), as a new detecting coating material on QCM for extremely sensitive FA detection [Citation88]. The NFN structure () had a big specific surface area, great porosity and large stacking density, which were very useful for sensing. They reported that the detection response mainly happened due to the nucleophilic addition interaction between the amine groups of PEI molecules and FA. Their QCM sensor had a very low detection limit of 50 ppb for FA and a response time of less than 100 seconds ().
Figure 11. QCM sensing layers schematic representation. Reused with permission from ref [Citation88]. Copyright 2011 Royal Society of Chemistry.
![Figure 11. QCM sensing layers schematic representation. Reused with permission from ref [Citation88]. Copyright 2011 Royal Society of Chemistry.](/cms/asset/49579583-263b-48a9-ab30-ba86b14abf7c/tsta_a_2018920_f0011_oc.jpg)
Another sensor strip utilizing 4-amino-3-penten-2-one (fluoral-p) modified electrospun polyacrylonitrile (PAN) (PAN/fluoral-p) (Probe 28) was developed for naked-eye detection of FA [Citation89]. The sensor strips displayed an important reflectance declining band at 417 nm which brought an intense color change from white to yellow due to the Hantzsch reaction between FA and probe. The probable mechanism of formaldehyde detection is shown in (). Due to the enormously big surface area and great porosity of the nanofibrous membranes, the sensitivity is much greater than normal filter paper-based probes. The sensor reached a very low detection limit of 40 ppb.
Figure 12. Reaction mechanism of the Probe 28. Reused with permission from ref [Citation89]. Copyright 2013 Royal Society of Chemistry.
![Figure 12. Reaction mechanism of the Probe 28. Reused with permission from ref [Citation89]. Copyright 2013 Royal Society of Chemistry.](/cms/asset/de83a7b3-c74a-4cb2-a6bf-4ac014c596e5/tsta_a_2018920_f0012_oc.jpg)
In 2015, Chuang et al. demonstrated a printable combination material of reduced graphene oxide (RGO) and PMMA Probe 29 for FA sensing [Citation90]. According to their study, 2% RGO/10% PMMA is the ideal ratio for FA sensing. The adsorption of FA disrupts the physical contact between PMMA and RGO, and the sensing sensitivity decreases. The detector exhibited decent selectivity for FA than other VOCs, carbon monoxide (CO), and nitric oxide (NO). The detection level of FA was estimated to be 100 ppm ().
Kanekiyo and co-workers reported a sensor Probe 30 based on a thin film consisting of primary amino groups on a glass substrate [Citation91]. The FA-responsive thin films were made by the radical copolymerization of a primary amine (N-(3-aminopropyl)methacrylamide hydrochloride), acrylamide and cross-linker (N,N′-methylene-bis-acrylamide) in the presence of 2,2′-azobis(2-methylpropionamidine)dihydrochloride as an initiator. After exposure with FA, the polymeric thin film was immersed in aqueous anionic dye solutions and the absorption spectra were recorded. They were successful in measuring 0–100 mM FA and the detection limit was calculated to be 3 mM (). Additionally, the sensitivity of this detector was massively affected by humidity, exposure time, and the monomer conformation of the film.
4. Conclusion and future perspective
A systematic review of the development and evolution of the different types of small molecular and polymeric probes for sensitive as well as selective detection of FA, with a special emphasis on the advantages of polymeric probes, is presented here. Through this comprehensive study, we conclude that different types of fluorescent sensors were designed and developed by using the mechanism of aza-Cope rearrangement or FA-amine condensation reaction for extremely selective sensing. Mounting evidence indicates that, among different types of techniques, the reaction-based colorimetric method has emerged as a significant platform for detecting FA, as visible color change appears after selective addition of FA, which in turn is important for real-world applications. Furthermore, various research groups have developed different types of small molecular probes and effectively used them as fluorescent imaging agents for detection of exogenous as well as endogenous formaldehyde in various living animal cells. Apart from the small molecular probes, researchers have explored the potential and advantages of using polymeric probes to overcome the problems of poor aqueous solubility, high detection limit, and longer exposure time, suffered by small molecular probes.
Despite having promising applications, some challenges still exist to further revolutionize this field. A major bottleneck for developing polymeric probes is their synthetic procedures. It is difficult to synthesize large-scale polymeric probes due to the long synthetic pathways. Investigations are underway to overcome these problems to synthesize polymeric materials in industrial scales to detect and mitigate the harmful effects of FA in our daily life. Furthermore, after FA addition, there is always the problem of biodegradability. In this regard, amino acid terminated polymers are evolving as a potential solution. Though developing novel polymeric materials for selective FA detection have gained significant attention of researchers in recent years, only a few reports are available wherein polymeric materials have been successfully used for in vivo study.
We believe that a lack of understanding of the reaction mechanism between polymeric probes and FA restricts their full potential. In addition to this, in most of the literature reports, the synthesized sensing systems are for single use only. This limits their applications for environmental monitoring where continuous and repeated monitoring play an important role. Therefore, a thorough investigation of the in vivo behavior is the most requirement for their clinical applications and as suitable therapeutic agents. Yet, the finding of novel polymeric materials will offer a ground-breaking push to the quest for new operative treatments for the chronic biological disorders causing damage to a large section of the human population [Citation92]. We are hopeful that this in-depth discussion about FA sensing mechanistic pathway and the recent development of the polymeric probes will highly encourage the scientific community to overcome and find a permanent solution to the FA problem. However, further studies are needed for developing the probable practices of using polymeric materials for successful application in numerous diseases.
Acknowledgments
PD acknowledges Scheme for Transformational and Advanced Research in Sciences (STARS), Ministry of Human Resource Development, Government of India [File No.: STARS/APR2019/CS/122/FS]. SR acknowledges the Council of Scientific and Industrial Research (CSIR), Government of India, for the junior research fellowship (JRF). PPB acknowledges DST-INSPIRE, Government of India, for fellowship.
Disclosure statement
No potential conflict of interest was reported by the author(s).
Additional information
Funding
Notes on contributors
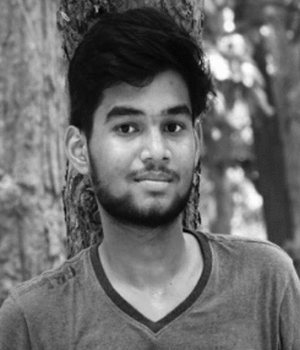
Swagata Pan
Swagata Pan is currently a PhD student in Prof. Priyadarsi De’s group in the Department of Chemical Sciences at Indian Institute of Science Education and Research Kolkata (IISER Kolkata), West Bengal, India. He received his BS-MS dual degree from IISER Kolkata in 2020. His research interest includes synthesis of biocompatible polymers for selective sensing of different toxic metal ions and volatile organic compounds.
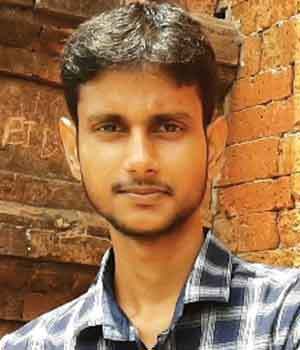
Subhadip Roy
Subhadip Roy is currently a PhD student in Dr. Priyadarsi De’s research group at Indian Institute of Science Education and Research Kolkata (IISER Kolkata), West Bengal, India. He received BSc degree in 2017 in Chemistry from Vidyaswagar University, India. In 2019, he obtained MSc degree from Presidency University. His research interest focuses on the design and development of polymeric sensors for the detection of toxic small molecules.
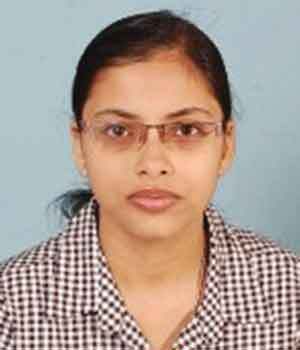
Neha Choudhury
Dr. Neha Choudhury received her Ph.D. in 2020 from the group of Prof. Priyadarsi De at the Indian Institute of Science Education and Research Kolkata (IISER Kolkata), India. She is now a postdoctoral fellow in the group of Prof. Byeong-Su Kim at the Yonsei University (Republic of Korea), and her research is focused on biodegradable polymers.
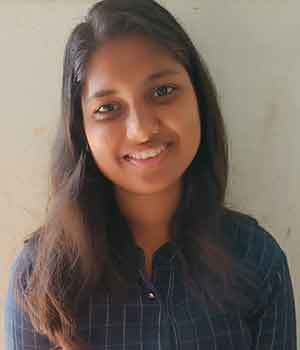
Priyanka Priyadarshini Behera
Priyanka Priyadarshini Behera is currently a 3rd-year BS-MS student in the Department of Chemical Sciences at Indian Institute of Science Education and Research Kolkata (IISER Kolkata), West Bengal, India. Her research interests include synthetic chemistry, chemical biology, drug delivery, and polymer chemistry. She is mainly interested in the synthesis of stimuli-responsive polymers for potential biomedical applications and future possibilities.
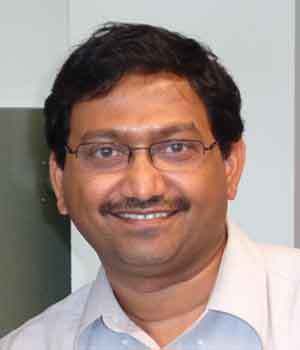
Kannan Sivaprakasam
Dr. Kannan Sivaprakasam is a Professor in the Department of Chemistry and Biochemistry at St. Cloud State University, St. Cloud (USA). He received his Ph.D. in chemistry from the Indian Institute of Science, Bangalore in 1999. He was a postdoctoral fellow in University of Bordeaux from 1998-1999, Cornell University from 2000-2006, and University of Minnesota from 2006-2007. After a brief stint at Paddock Labs from 2007-2009, he joined St. Cloud State University in 2009. His interdisciplinary research group includes students majoring in chemistry, physics, biomedical science, materials science, electrical and mechanical engineering. His research work focuses on developing hydrogel for biomedical devices, use of synthetic and modified aluminosilicates as detoxifiers for nitrates, phosphates and heavy metals.
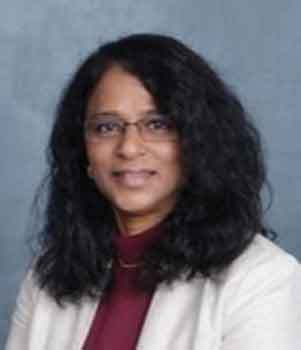
Latha Ramakrishnan
Dr. Latha Ramakrishnan is the Dean of the College of Science and Technology and a Professor in the Department of Chemistry and Biochemistry at Bloomsburg University of Pennsylvania, PA, U.S.A. She received her Ph.D. in Physical Polymer Science from Indian Institute of Science, Bangalore, India. She completed her post-doctoral training and held a research associate position at Cornell University, Ithaca, NY between 2000-2006. She started her academic career as an Assistant Professor of Biochemistry in 2006 at St. Cloud State University and became a full professor there in 2014. Dr. Ramakrishnan’s research interest includes studying molecular mechanisms of GABAergic neurotransmission systems in neurological diseases such as epilepsy, investigating behavioral pharmacology of convulsant and anticonvulsant drugs in flatworms, and polymeric SiRNA delivery systems for investigating GABAergic neurotransmission in planaria.
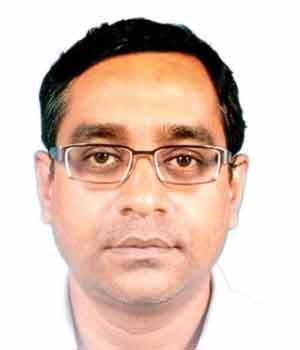
Priyadarsi De
Dr. Priyadarsi De is a Professor in the Department of Chemical Sciences at Indian Institute of Science Education and Research Kolkata (IISER Kolkata), India. He received his Ph.D. degree from Indian Institute of Science, Bangalore, India. After his post-doctoral studies at UMASS Lowell (2002-2006) and Southern Methodist University (2007-2008), he worked in PhaseRx, Inc., Seattle, for fifteen months before joining IISER Kolkata in November, 2009. His research group at IISER Kolkata mostly works on controlled synthesis of bio-inspired macromolecular architectures from naturally occurring amino acids and fatty acid based renewable resources for various applications. He is Associate Editor of Journal of Macromolecular Science, Part A: Pure and Applied Chemistry (Taylor & Francis Group, April, 2019 to Present), and Editorial Advisory Board Member of Polymer Chemistry (Royal Society of Chemistry, September 2015 - Present).
References
- Leikauf GD. Environmental toxicants: human exposures and their health effects. 3rd ed., Lippmann M. Ed. Hoboken (NJ): Wiley; 2009. p. 257–316.
- Salthammer T, Mentese S, Marutzky R. Formaldehyde in the indoor environment. Chem Rev. 2010;110:2536–2572.
- Puchtler H, Meloan SN. On the chemistry of formaldehyde fixation and its effects on immunohistochemical reactions. Histochemistry. 1985;82:201–204.
- Liteplo RG, Beauchamp R, Meek ME, et al. Formaldehyde concise international chemical assessment document 40. Geneva: World Health Organization; 2002.
- Baan R, Grosse Y, Straif K, et al. A review of human carcinogens-part F: chemical agents and related occupations. Lancet Oncol. 2009;10:1143–1144.
- Formaldehyde, 2-Butoxyethanol and 1-tert-Butoxypropan-2-ol. IARC Monographs on the Evaluation of Carcinogenic Risks to Humans: World Health Organization International Agency for Research on Cancer: Lyon, France, 2006;88: p. 39–329.
- WHO air quality guidelines for Europe. 2nd ed. Copenhagen: World Health Organization; 2000.
- Park HJ, Kim J, Choi NJ, et al. Nonstoichiometric Co-rich ZnCo2O4 hollow nanospheres for high performance formaldehyde detection at ppb levels. ACS Appl Mater Interfaces. 2016;8:3233–3240.
- Geng BY, Fang CH, Zhan FM, et al. Synthesis of polyhedral ZnSnO3 microcrystals with controlled exposed facets and their selective gas-sensing properties. Small. 2008;4:1337–1343.
- Formaldehyde (CASRN 50−00−0). Integrated risk information system. Document 0419. Washington (D.C): U.S. Environmental Protection Agency; 1998.
- Tong Z, Han C, Luo W, et al. Accumulated hippocampal formaldehyde induces age-dependent memory decline. Age (Omaha). 2013;35:583–596.
- Reingruber H, Pontel LB. Formaldehyde metabolism and its impact on human health. Curr Opin Toxicol. 2018;9:28–34.
- Kilhovd BK, Juutilainen A, Lehto S, et al. Increased serum levels of methylglyoxal-derived hydroimidazolone-AGE are associated with increased cardiovascular disease mortality in nondiabetic women. Atherosclerosis. 2009;205:590–594.
- Kim WJ, Terada N, Nomura T, et al. Effect of Formaldehyde on the Expression of Adhesion Molecules in Nasal Microvascular Endothelial Cells: the role of formaldehyde in the pathogenesis of sick building syndrome. Clin Exp Allergy. 2002;32:287–295.
- Devaraj P, Babu V, Cengiz EI. Qualitative detection of formaldehyde and ammonia in fish and other seafoods obtained from Chennai’s (India) fish markets. Environ Monit Assess. 2021;193(1–8):78.
- Lu J, Miao JY, Su T, et al. Formaldehyde induces hyperphosphorylation and polymerization of tau protein both in vitro and in vivo. Biochim Biophys Acta Gen Subj. 2013;1830:4102–4116.
- Zhang Z, Zhao C, Ma Y, et al. Rapid analysis of trace volatile formaldehyde in aquatic products by derivatization reaction-based surface enhanced raman spectroscopy. Analyst. 2014;139:3614–3621.
- Yu PH, Cauglin C, Wempe KL, et al. A novel sensitive high-performance liquid chromatography/electrochemical procedure for measuring formaldehyde produced from oxidative deamination of methylamine and in biological samples. Anal Biochem. 2003;318:285–290.
- Ohata H, Otsuka M, Ohmori S. Determination of acetaldehyde in biological samples by gas chromatography with electron-capture detection. J Chromatogr B: Biomed Sci Appl. 1997;693:297–305.
- Bunkoed O, Davis F, Kanatharana P, et al. Sol-gel based sensor for selective formaldehyde determination. Anal Chim Acta. 2010;659:251–257.
- Chung FC, Wu RJ, Cheng FC. Fabrication of an Au@SnO2 core–shell structure for gaseous formaldehyde sensing at room temperature. Sensor Actuat B Chem. 2014;190:1–7.
- Xie H, Sheng C, Chen X, et al. Multi-wall carbon nanotube gas sensors modified with amino-group to detect low concentration of formaldehyde. Sensor Actuat B Chem. 2012;168:34–38.
- Ishihara S, Labuta J, Nakanishi T, et al. Amperometric detection of sub-ppm formaldehyde using single-walled carbon nanotubes and hydroxylamines: a referenced chemiresistive system. ACS Sens. 2017;2:1405–1409.
- Mitsubayashi K, Matsunga H, Nishio G, et al. Bio-sniffer sticks for breath analysis after drinking. Sensor Actuat B Chem. 2005;108:660–664.
- Lorrain JM, Fortune CR, Dellinger B. Sampling and ion chromatographic determination of formaldehyde and acetaldehyde. Anal Chem. 1981;53:1302–1305.
- Zhou Y, Yan J, Zhang N, et al. A ratiometric fluorescent probe for formaldehyde in aqueous solution, serum and air using Aza-Cope reaction. Sensor Actuat B Chem. 2018;258:156–162.
- Brewer TF, Chang CJ. An Aza-Cope reactivity-based fluorescent probe for imaging formaldehyde in living cells. J Am Chem Soc. 2015;137:10886–10889.
- Chang X, Wang Z, Qi Y, et al. Dynamic chemistry-based sensing: a molecular system for detection of saccharide, formaldehyde, and the silver ion. Anal Chem. 2017;89:9360–9367.
- Li J, Hou C, Huo D, et al. Development of a colorimetric sensor array for the discrimination of aldehydes. Sensor Actuat B Chem. 2014;196:10–17.
- Choudhury N, De P. Recent progress in pendant rhodamine-based polymeric sensors for the detection of copper, mercury and iron ions. J Macromol Sci Part A. 2021. DOI:10.1080/10601325.2021.1960172
- Bhattacharya S, Shunmugam R. Polymer based gels and their applications in remediation of dyes from textile effluents. J Macromol Sci Part A. 2020;57:906–926.
- Choudhury N, Mete S, Kambalpalli S, et al. Side‐chain glycylglycine‐based polymer for simultaneous sensing and removal of copper (II) from aqueous medium. J Polym Sci A Polym Chem. 2018;56:914–921.
- Choudhury N, Saha B, Ruidas B, et al. Dual-action polymeric probe: Turn-on sensing and removal of Hg2+; chemosensor for HSO4–. ACS Appl Polym Mater. 2019;1:461–471.
- Choudhury N, Ruidas B, Mukhopadhyay CD, et al. Rhodamine-appended polymeric probe: an efficient colourimetric and fluorometric sensing platform for Hg2+ in aqueous medium and living cells. ACS Appl Polym Mater. 2020;2:5077–5085.
- Choudhury N, Saha B, De P. Recent progress in polymer-based optical chemosensors for Cu2+ and Hg2+ ions: a comprehensive review. Eur Polym J. 2021;145(1–35):110233.
- Bauri K, Saha B, Mahanti J, et al. A nonconjugated macromolecular luminogen for speedy, selective and sensitive detection of picric acid in water. Polym Chem. 2017;8:7180–7187.
- Saha B, Choudhury N, Seal S, et al. Aromatic nitrogen mustard-based autofluorescent amphiphilic brush copolymer as ph-responsive drug delivery vehicle. Biomacromolecules. 2018;20:546–557.
- Hu J, Liu S. Engineering responsive polymer building blocks with host-guest molecular recognition for functional applications. Acc Chem Res. 2014;47:2084–2095.
- Choudhury N, Ruidas B, Saha B, et al. Multifunctional tryptophan-based fluorescent polymeric probes for sensing, bioimaging and removal of Cu2+ and Hg2+ ions. Polym Chem. 2020;11:2015–2026.
- Liu H, Lin S, Feng Y, et al. CO2-responsive polymer materials. Polym Chem. 2017;8:12–23.
- Schattling P, Jochum FD, Theato P. Multi-stimuli responsive polymers−the all-in-one talents. Polym Chem. 2014;5:25–36.
- Mei J, Leung NL, Kwok RT, et al. Aggregation-induced emission: together we shine, United We Soar! Chem Rev. 2015;115:11718–11940.
- Qin A, Lam JWY, Tang BZ. Luminogenic polymers with aggregation-induced emission characteristics. Prog Polym Sci. 2012;37:182–209.
- Bi A, Yang S, Liu M, et al. Fluorescent probes and materials for detecting formaldehyde: from laboratory to indoor for environmental and health monitoring. RSC Adv. 2017;7:36421.
- Manna SK, Achar TK, Mondal S. Recent advances in selective formaldehyde detection in biological and environmental samples by fluorometric and colorimetric chemodosimeters. Anal Methods. 2021;13(1–22):1084.
- Xie Z, Ge J, Zhang H, et al. A highly selective two-photon fluorogenic probe for formaldehyde and its bioimaging application in cells and zebrafish. Sensor Actuat B Chem. 2017;241:1050–1056.
- Bruemmer KJ, Walvoord RR, Brewer TF, et al. Development of a general Aza-Cope reaction trigger applied to fluorescence imaging of formaldehyde in living cells. J Am Chem Soc. 2017;139:5338–5350.
- Song X, Han X, Yu F, et al. A reversible fluorescent probe based on C−N isomerization for the selective detection of formaldehyde in living cells and in vivo. Analyst. 2018;143:429–439.
- Yang M, Fan J, Du J, et al. Imaging of formaldehyde in live cells and daphnia magna via Aza-Cope reaction utilizing fluorescence probe with large stokes shifts. Front Chem. 2018;6(1–10):488.
- Zhou W, Dong H, Yan H, et al. HCHO-reactive molecule with dual-emission-enhancement property for quantitatively detecting HCHO in near 100% water solution. Sensor Actuat B Chem. 2015;209:664–669.
- Lee YH, Tang YH, Verwilst P, et al. A biotin-guided formaldehyde sensor selectively detecting endogenous concentrations in cancerous cells and tissues. Chem Commun. 2016;52:11247–11250.
- Tang Y, Kong X, Xu A, et al. Development of a two-photon fluorescent probe for imaging of endogenous formaldehyde in living tissues. Angew Chem Int Ed. 2016;55:3356–3359.
- Tang Y, Kong X, Liu Z-R, et al. Lysosome-targeted Turn-on fluorescent probe for endogenous formaldehyde in living cells. Anal Chem. 2016;88:9359–9363.
- Li Z, Xue Z, Wu Z, et al. Chromo-fluorogenic detection of aldehydes with a rhodamine-based sensor featuring an intramolecular deoxylactam. Org Biomol Chem. 2011;9:7652–7654.
- He L, Yang X, Ren M, et al. An ultrafast illuminating fluorescent probe for monitoring formaldehyde in living cells, shiitake mushrooms, and indoors. Chem Commun. 2016;52:9582–9585.
- Jiang L, Hu Q, Chen T, et al. Highly sensitive and rapid responsive fluorescence probe for determination of formaldehyde in seafood and in vivo imaging application. Spectrochim Acta Part A. 2020;228(1–7):117789.
- Hongwei G, Guoqiang L, Ranhao Y, et al. An aldimine condensation reaction based fluorescence enhancement probe for detection of gaseous formaldehyde. Microchem J. 2020;156(1–6):104793.
- Uno S-N, Kamiya M, Yoshihara T, et al. A spontaneously blinking fluorophore based on intramolecular spirocyclization for live-cell super-resolution imaging. Nat Chem. 2014;6:681–689.
- Yang X, He L, Xu K, et al. The development of an ICT-based formaldehyde-responsive fluorescence Turn-on probe with a high signal-to-noise ratio. New J Chem. 2018;42(1–4):12361.
- Hao Y, Zhang Y, Zhang A, et al. A benzothiazole-based ratiometric fluorescent probe for detection of formaldehyde and its applications for bioimaging. Spectrochim Acta Part A. 2020;229(1–6):117988.
- Roth A, Li H, Anorma C, et al. A reaction-based fluorescent probe for imaging of formaldehyde in living cells. J Am Chem Soc. 2015;137:10890–10893.
- Wang T, Douglass EF, Fitzgerald KJ, et al. A “Turn-On” fluorescent sensor for methylglyoxal. J Am Chem Soc. 2013;135:12429–12433.
- Chen J, Zeng L, Xia T, et al. Toward a Biomarker of Oxidative Stress: a Fluorescent Probe for Exogenous and Endogenous Malondialdehyde in Living Cells. Anal Chem. 2015;87:8052–8056.
- Togashi M, Terai T, Kojima H, et al. Practical fluorescence detection of acrolein in human plasma via a two-step tethering approach. Chem Commun. 2014;50:14946–14948.
- Ueno T, Urano Y, Kojima H, et al. Mechanism-based molecular design of highly selective fluorescence probes for nitrative stress. J Am Chem Soc. 2006;128:10640–10651.
- Zhao X, Ji C, Ma L, et al. An aggregation-induced emission-based “Turn-On” fluorescent probe for facile detection of gaseous formaldehyde. ACS Sens. 2018;3:2112–2117.
- He LW, Yang XL, Liu Y, et al. A ratiometric fluorescent formaldehyde probe for bioimaging applications. Chem Commun. 2016;52:4029–4032.
- Gupta VK, Agarwal S, Jakob A, et al. A Zinc-selective Electrode based on N,N′-Bis(acetylacetone)ethylenediamine. Sensor Actuat B Chem. 2006;114:812–818.
- Jain AK, Gupta VK, Ganeshpure PA, et al. Ni(II)-selective ion sensors of salen type schiff base chelates. Anal Chim Acta. 2005;553:177–184.
- Liu T, Zhang YF, Hou TT, et al. Sensitive fluorescent detection of fibrin based on the inner filter effect of gold nanoparticles. RSC Adv. 2017;7:23422–23426.
- Song H, Rajendiran S, Kim N, et al. A tailor designed fluorescent ‘Turn-On’ sensor of formaldehyde based on the BODIPY motif. Tetrahedron Lett. 2012;53:4913–4916.
- Wu Y, Zheng Z, Wen J, et al. Imaging of formaldehyde in live cells and plants utilizing small molecular probes with large stokes shifts. Sensor Actuat B Chem. 2018;260:937–944.
- Chen W, Yang M, Luo N, et al. An intramolecular charge transfer and excited state intramolecular proton transfer based fluorescent probe for highly selective detection and imaging of formaldehyde in living cells. Analyst. 2019;144:6922–6927.
- Liu C, Shi C, Li H, et al. Nanomolar fluorescent quantitative detection of formaldehyde with an 8-hydroxyquinoline derivative in aqueous solution and electrospun nanofibers. Sensor Actuat B Chem. 2015;219:185–191.
- Xu A, Tang Y, Lin W. Development of a mitochondrial-targeted two-photon fluorescence turn-on probe for formaldehyde and its bio-imaging applications in living cells and tissues. New J Chem. 2018;42:8325–8329.
- Yuan W, Zhong X, Han Q, et al. A novel formaldehyde fluorescent probe based on 1, 8-naphthalimide derivative and its application in living cell. J Photochem Photobiol A. 2020;400(1–6):112701.
- Feng L, Musto CJ, Suslick KS, et al. Highly sensitive colorimetric detection method for gaseous formaldehyde. J Am Chem Soc. 2010;132:4046–4047.
- Fan YQ, Huang Q, Zhang YM, et al. Forming a water-soluble supramolecular polymer and an AIEE Hydrogel: two novel approaches for highly sensitive detection and efficient adsorption of aldehydes. Polym Chem. 2019;10:6489–6494.
- Li P, Zhang D, Zhang Y, et al. Ultrafast and efficient detection of formaldehyde in aqueous solutions using chitosan-based fluorescent polymers. ACS Sens. 2018;3:2394–2401.
- Alizadeh T, Soltani LH. Graphene/poly(methyl methacrylate) chemiresistor sensor for formaldehyde odor sensing. J Hazard Mater. 2013;248-249:401–406.
- Sayed SE, Pascual L, Licchelli M, et al. Chromogenic detection of aqueous formaldehyde using functionalized silica nanoparticles. ACS Appl Mater Interfaces. 2016;8:14318–14322.
- Antwi-Boampong S, BelBruno JJ. Detection of formaldehyde vapor using conductive polymer films. Sensor Actuat B Chem. 2013;182:300–306.
- Tang X, Raskin JP, Lahem D, et al. A formaldehyde sensor based on molecularly-imprinted polymer on a TiO2 nanotube array. Sensors. 2017;17(1–15):675.
- Iqbal N, Afzal A, Mujahid A. Layer-by-layer assembly of low-temperature- imprinted poly(methacrylic acid)/gold nanoparticle hybrids for gaseous formaldehyde mass sensing. RSC Adv. 2014;4:43121–43130.
- Hussain M, Kotova K, Lieberzeit PA. Molecularly imprinted polymer nanoparticles for formaldehyde sensing with QCM. Sensors. 2016;16(1–9):1011.
- Wang Z, Wang W, Sun G, et al. Designed ionic microchannels for ultrasensitive detection and efficient removal of formaldehyde in an aqueous solution. ACS Appl Mater Interfaces. 2020;12:1806–1816.
- Liu G, Shegiwal A, Zeng Y, et al. Polymers for fluorescence imaging of formaldehyde in living systems via the hantzsch reaction. ACS Macro Lett. 2018;7:1346–1352.
- Ding B, Wang X, Yu J, et al. Polyamide 6 composite nano-fiber/net functionalized by polyethyleneimine on quartz crystal microbalance for highly sensitive formaldehyde sensors. J Mater Chem. 2011;21:12784–12792.
- Wang X, Si Y, Mao X, et al. Colorimetric sensor strips for formaldehyde assay utilizing fluoral-p decorated polyacrylonitrile nanofibrous membranes. Analyst. 2013;138:5129–5136.
- Chuang WY, Yang SY, Wu WJ, et al. A room-temperature operation formaldehyde sensing material printed using blends of reduced graphene oxide and poly(methyl methacrylate). Sensors. 2015;15:28842–28853.
- Kanekiyo Y, Mitani Y, Suda M, et al. Development of formaldehyde gas sensor that exhibits distinct color changes. Sens Mater. 2020;32:1101–1109.
- Ghosh P, Bera A, Bhadury P, et al. From small molecules to synthesized polymers: potential role in combating amyloidogenic disorders. ACS Chem Neurosci. 2021;12:1737–1748.