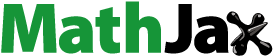
ABSTRACT
The oxygen reduction reaction (ORR) and oxygen evolution reaction (OER) are important processes for various energy devices, including polymer electrolyte fuel cells, rechargeable metal–air batteries, and water electrolyzers. We herein report the preparation of a rare metal-free and highly efficient ORR/OER electrocatalyst by calcination of a mixture of blood meal and ascidian-derived cellulose nanofibers. The obtained carbon alloys showed high ORR/OER performances and proved to be promising electrocatalysts. The carbon alloys synthesized entirely from biomass resources not only lead to a new electrocatalyst fabrication process but also contribute to CO2 reduction and the realization of a good life-cycle assessment value in fabrication of a sustainable energy device.
CLASSIFICATIONS:
1. Introduction
The oxygen reduction reaction (ORR) and oxygen evolution reaction (OER) are important processes for various energy devices, including polymer electrolyte fuel cells (PEFCs), rechargeable metal–air batteries, and water electrolyzers. At the cathode of PEFCs [Citation1] and metal–air batteries [Citation2] [Citation3,Citation4],, O2 molecules in the air are reduced to hydroxyl ions via the ORR and efficient catalysts are needed to lower the overpotential of the reaction. To achieve highly efficient energy conversion, Pt supported on carbon black (Pt/C) has been used as an ORR catalyst. However, the use of Pt, which is expensive and resource-constrained, has hindered the widespread use of these batteries [Citation5]. To avoid resource constraints and reduce costs, catalytic materials that do not use heavy or precious metals such as Pt are needed [Citation6]. The OER occurring at the cathode of rechargeable metal–air batteries and at the anode of water electrolysis systems [Citation7] is also an important process for efficient O2 generation in such devices [Citation8]. Pt, Ir, Ru, and other precious-metal catalysts have been used to reduce the OER overpotential, which is associated with the bottleneck process in water electrolysis.
For decades, bifunctional catalysts have been extensively explored as alternatives to precious-metal catalysts [Citation9], and numerous materials, including core–shell metal nanoparticles [Citation10] and metal oxide nanoparticles [Citation11], have been substituted for rare metals and rare-earth metals in ORR and OER catalysts. In addition, the literature includes reports that show carbon alloys (CAs) containing pyridine groups and metal atoms M surrounded by four pyridines (MN4 structure, especially FeN4) embedded in a graphite structure exhibit substantially enhanced ORR activity [Citation12]. Consequently, the development of CAs containing pyridine groups and MN4 structures has been intensively investigated [Citation13] [Citation14–16]. Ozaki and coworkers reported the preparation of CAs containing FeN4 structures by calcination of iron phthalocyanines and a polymer resin [Citation17]. Molecular adsorption of iron phthalocyanine and other derivatives to form carbon composites with FeN4 structures has also been examined [Citation18] [Citation19,Citation20]. However, these carbon sources are usually synthetic and require multistep synthesis routes involving substantial CO2 emissions. Thus, these materials have a high environmental impact in terms of life-cycle assessment (LCA) given that the number of PEFCs, metal–air batteries, and electrolyzers will increase even though they will incorporate conventional alternative electrocatalysts.
Sustainable resources, including recycled materials and biomass resources, provide a promising path to reducing carbon footprints associated with the synthesis of electrocatalysts. In particular, the synthesis of heteroatom-doped electrocatalysts from biomass resources is a substantial challenge toward realizing a sustainable energy source [Citation21] [Citation22]. The literature includes reports of the synthesis of electrocatalysts from biomass resources [Citation23] [Citation24,Citation25]. Hemoglobin and blood meal (BM), which is a dried waste blood from the meat processing of livestock, contain large amounts of heme protein and have strong potential as an FeN4 resource for electrocatalysts [Citation26]. Huge amounts of BMs (over thousands of tons per year) were produced and most of them were thrown away and causes the environmental pollution since it increases biochemical oxygen demand (BOD) of rivers, lakes and other water environments. Only a part of them were used as low-cost fertilizers containing high amount of Fe, N and P resources. The synthesis of ORR catalysts via pyrolysis of pig blood has been reported [Citation27] [Citation28]. Normally, to achieve sufficient catalytic activity and electrical conductivity to enable the pyrolysis of pig blood or BM itself, metal/metal oxide sources [Citation29] [Citation30], and carbon materials [Citation31] [Citation32–34], are added to the precursors. However, the literature contains few reports on the synthesis of electrocatalysts from other biomass resources.
Cellulose nanofibers (CNFs) are widely used in structural components and as reinforcements for polymer materials because of their high mechanical and thermal stabilities, which arise from the highly crystalline structure of cellulose. Furthermore, amount of ascidian tunicates as a biomass is also huge enough, which is over 7,000 tons/year obtained as same as BMs, which is enough amount to produce CNFs over tens tons/year. Comparing with wood biomass, the advantage of CNFs from ascidian tunicates is they can be harvested every year. Interestingly, CNFs obtained from marine organisms, including seaweeds and ascidians, exhibit greater crystallinity [Citation35] and a larger crystalline size than wood-based CNFs. In addition, calcining marine CNFs leads to the formation of carbon materials with highly graphitic structures, which in turn results in highly conductive carbons [Citation36] [Citation37]. Given the high conductivity of pyrolyzed CNFs prepared from marine bioresources, we anticipated that combining BMs and CNFs from marine bioresources would be a promising approach for preparing ORR/OER electrocatalysts with high catalytic activity and high electron conductivity. Actually, we have recently reported pyrolysis of polydopamine-coated cellulose nanocrystals obtained from ascidians show good ORR activities [Citation38].
In the present paper, we report the synthesis of bifunctional heteroatom-doped CA electrocatalysts for both the ORR and the OER by pyrolyzing BM and CNFs from ascidians. The chemical properties and electrochemical performance of the synthesized CAs are discussed.
2. Experimental details
2.1. Materials
BM from pig was kindly supplied by Tomikura Sangyo. An alkaline aqueous dispersion of CNFs was obtained from ascidians in the Miyagi prefecture, Japan, using a previously reported sodium hypochlorite treatment method [Citation39]. Details of the extraction process of CNFs from tunicates of ascidian are provided in the supporting information, S1. To remove excess sodium hypochlorite, the CNFs were washed three times with membrane-filtered water and collected by centrifugation (~3000 rpm). After purification, a 2 wt% dispersion of CNFs in membrane-filtered water was prepared.
2.2. Synthesis of carbon alloys
The BM and CNFs were mixed in various weight ratios by hand grinding in water, and the resultant mixtures were dried at room temperature in vacuo. In order to avoid those heterogeneity, we mixed and grinded to homogenized the samples before pyrolysis. The pyrolyzed samples were derived from the same crude materials after mixing and grinding, therefore we considered that there are no intended heterogeneities. The dried samples were pyrolyzed in an electric oven at 700, 800, or 900°C in vacuo for 3 h. The heating rate was 20°C/min. After pyrolysis, the sample was slowly cooled to room temperature. After this pyrolysis process, powder-like CAs were obtained.
2.3. Characterization
Structures of pyrolyzed samples were observed using a scanning electron microscope (S-5200, Hitachi, Japan) and a transmission electron microscope (H-7650, Hitachi, Japan). In situ energy-dispersive X-ray analysis (EDX) spectra of samples were obtained using a transmission electron microscope equipped with an EDX system (EDAX, Genesis) and operated at an acceleration voltage of 100 kV. X-ray photoelectron spectroscopy (XPS) measurements were performed with a JPS9200 spectrometer (JEOL; Al Kα, 10 kV, 10 mA). A wide scan was performed from 0 to 1400 eV in 1 eV steps, and a narrow scan was performed for each element in 0.1 eV steps. Thermogravimetric analysis was performed on RIGAKU Thermo plus EvoII TG-DTA8210 at a heating rate of 10°C/min under a nitrogen atmosphere.
2.4. ORR/OER measurements
The ORR/OER performances were evaluated by linear-sweep voltammetry (LSV) measurements using a potentiostat (2325, BAS, Japan). Catalyst inks for each sample were prepared by dispersing 0.82 mg of catalyst in a 1 mL solution consisting of 6 μL Nafion (527,084, Sigma-Aldrich, USA), 334 μL isopropyl alcohol, and 84 μL water via sonication for 5 min. Twenty microliters of the ink was then cast onto a glassy carbon (GC, BAS, Japan) insert of a rotating ring-disk electrode (RRDE; 4 mm diameter, BAS, Japan) and dried. The catalyst loading on the electrode was 300 μg/cm2. A Pt wire and a Ag/AgCl electrode were inserted into the electrolyte as reference and counter electrodes, respectively. A 0.1 M KOH solution bubbled with N2 or O2 for 30 min was used as the electrolyte. In addition, 3 M methanol was added to an O2-saturated 0.1 M KOH solution for evaluation of the methanol oxidation activity of the catalysts.
The potential vs Ag/AgCl was converted to the reversible hydrogen electrode (RHE) scale using the following equation:
E(vs RHE) = E(vs Ag/AgCl) + 0.197 + 0.059 V·pH (1)
The number of electrons (n) involved in the ORR was calculated according to the Koutecký–Levich (K–L) equation:
where J, Jk, and Jd are the measured, kinetic, and diffusion-limiting current, respectively; F is the Faraday constant (96,485 C/mol); A is the electrode area (0.1256 cm2); k is the rate constant for oxygen reduction (M/s); DO2 is the diffusion coefficient of O2 in the electrolyte (1.93 × 10−5 cm2/s); ν is the viscosity of the electrolyte solution (1.009 × 10−5 cm2/s); CO2 is the saturated concentration of O2 in the electrolyte (1.26 × 10−6 mol/cm); and ω is the angular rotation rate.
The number of electrons n involved in the ORR was also calculated using the RRDE results and the following equation:
where ID and IR are the current densities of the disk and ring electrodes, respectively, and N is the capture efficiency (0.42). Reproducibility of the performance for several times from different crude materials.
3. Results and discussion
3.1. Chemical characterization of CAs
CAs with various CNF/BM ratios were prepared by pyrolysis of the CNFs and dried BM at 700, 800, or 900°C in vacuo. Sample compositions and pyrolyzed temperature (Tpyrolysis) are listed up in . shows typical scanning electron microscope (SEM) images of CNFs extracted from ascidians and dried BM. From the SEM image, the average width and length of the CNFs was 28.9 nm (see supporting information, S1) and greater than several micrometers, respectively. TGA curves and SEM images of pyrolyzed samples were shown in the supporting information, S7 and S8. From TGA curves, the weight of CNF almost burned at 900°C, but it still existed small percentage (1 ~ 2%). I agree with the reviewer’s comment that main component of carbon alloys derived from BMs. From those TGA curves, only a small amount of CNF derived carbons should exist in the carbon alloys.
Table 1. List of samples and their representative electrocatalytic performances measured at pH 13
BM is a mixture of blood proteins and lipids; its SEM images therefore show no clear characteristic structures. ) shows a typical SEM image of a pyrolyzed mixture of CNFs and BM. After pyrolysis, the CNFs and BM were transformed into a thin fibrous network and bulk sintered bodies, respectively.
To analyze the chemical compositions of the CAs prepared by pyrolysis of the BM and CNF mixtures, TEM–EDX and XPS analyses were performed. ) shows a typical TEM–EDX spectrum of one of the CA samples. Peaks attributable to C and O of the pyrolyzed CNFs and peaks attributable to the P and Fe of BM are clearly observed. The Ca was a contaminant from seashells, which were used as scaffolds for the ascidians during aquaculture. The Cu and other small peaks were originated from the Cu grids and the sample holder, respectively. Because heteroatom-doped carbons exhibit drastically enhanced electrocatalytic activity, the incorporation of N, S, P, and metals into carbon materials is important for understanding the catalytic properties of these materials. The TEM–EDX spectra show clear peaks for P and Fe, which are known as dopants for electrocatalysts. show Fe/C and P/C ratios of each sample in terms of pyrolysis temperature (Tpyrolysis). From this result, Fe and P atoms are well incorporated into the resultant carbons pyrolyzed at 900°C. Nitrogen concentrations in samples are relatively lower than other elements in the XPS results, therefore, the low sensitivity of EDX spectroscopy for light elements and low abundance of nitrogen comparing to carbon may have prevented it from being observed.
Figure 2. A typical EDX spectrum of a CA (BM/CNF = 10/1, pyrolyzed at 900°C) (a) and the Fe/C (b) and P/C (c) ratios as functions of Tpyrolysis.
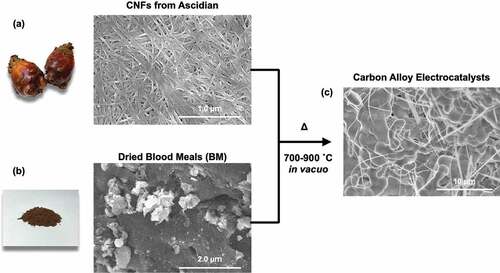
The wide-scan XPS spectra show that the obtained CAs contain N, O, and P atoms (–(c)). The strong C1s peak at 280–290 eV indicates that the pyrolyzed materials were converted into carbon materials. Small peaks attributed to P2p3/2, N1s, and O1s were observed at 133, 401, and 531 eV, respectively. In addition, small peaks attributed to Ca2p3/2 were observed at 350 eV, which is a contaminant from the original CNF.
Figure 3. Wide-scan XPS spectra of CAs pyrolyzed at 700°C (a), 800°C (b), and 900°C (c); narrow-scan XPS spectra of CAs prepared from precursors with a CNF/BM ratio of 1/2: N1s (d), Fe2p (e), C1s (f), and P2p3/2 (g).
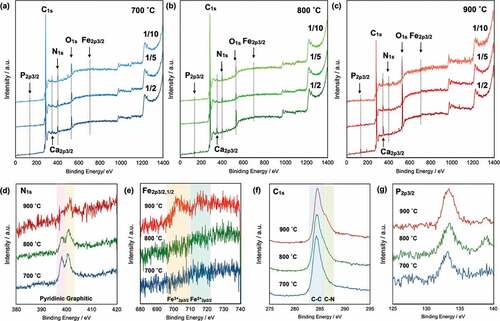
To discuss the effect of the Tpyrolysis on the carbon structures, narrow-scan XPS spectra were recorded for pyrolyzed samples. ) shows the narrow-scan spectra of the CA-1/2 samples pyrolyzed at 700°C (blue solid line), 800°C (green solid line), and 900°C (red solid line). Two distinct peaks attributable to pyridinic N and graphitic N were observed. Proteins do not normally contain pyridinic structures; the pyridinic N is therefore attributed to heme structures in the blood cells. Both peaks gradually decreased in intensity with increasing Tpyrolysis because the N-containing compounds are easily decomposed into N2 and NOx gases at elevated temperatures. However, small peaks remained in the spectrum of the CA pyrolyzed at 900°C. In addition, Fe peaks were not observed in the spectra of the CAs pyrolyzed at 700 and 800°C, whereas small Fe3+2p3/2 and Fe2+2p3/2 peaks emerged in the spectrum of the CA pyrolyzed at 900°C ()). These results indicate that Fe atoms were incorporated into the CA in the case of pyrolysis at 900°C. These results are consistent with the EDX analysis results, which indicate that the Fe/C ratio of the sample prepared at Tpyrolysis = 900°C is substantially greater than the Fe/C ratios of the samples prepared at Tpyrolysis = 700°C and 800°C. In the narrow-scan XPS spectra of C1s ()), peaks attributable to C–C bonds are clearly observed; however, shoulder peaks attributable to C–N bonds increase in intensity with increasing pyrolysis temperature [Citation40]. These simultaneous increases in C–N and Fe peak intensities indicate the formation of FeN4 structures based on the complex of Fe and pyridinic nitrogen in carbon. Because the FeN4 structure is important for the ORR, the CAs are expected to exhibit high ORR performance. In addition, the narrow-scan XPS spectra show that phosphate, which is a component of the phospholipids in the BM, was also incorporated into the CAs irrespective of the Tpyrolysis ()). The peak intensity and sharpness gradually increased with increasing Tpyrolysis.
These chemical characterization results indicate that the CNF/BM mixtures were decomposed into CAs. Moreover, they show that heteroatoms such as N and P were incorporated into the CAs pyrolyzed at 700 or 800°C and that N, P, and Fe were incorporated into the samples pyrolyzed at 900°C. The amount of Fe in 700 and 800 0 C is less and thus Fe-N4 structures were less formed. The P and Fe contents increased with increasing BM content. The content of heteroatoms was changed with changing the pyrolysis temperature. Especially, same metal complex introduction was observed in the literature [Citation41]. Metal complexes were successfully introduced to carbon alloys from metal ion and carbon precursors at high pyrolysis temperature due to high chemical reactivity and the content of metal complexes increased with increasing the pyrolysis temperature.
3.2. ORR performance
) shows the linear-sweep voltammograms of all of the prepared CAs, as recorded at 1600 rpm in 0.1 M KOH(aq) (pH 13) in the voltage range from 0.2 to 1.0 V vs RHE (see supporting information, S3, which shows LSV curves recorded at different rotation speeds for each sample). Parameters used to characterize the ORR performance of electrocatalysts include the onset potential (Eonset), half-wave potential (Ehalf), and maximum current density (Imax). High Eonset, Ehalf, and Imax values indicate high ORR catalytic performance. The curves clearly show that the CA samples pyrolyzed at 900°C (red solid lines) exhibit better ORR performance than the CAs pyrolyzed at 800°C (green solid lines) and 700°C (blue solid lines). The sample names and measured Eonset, Ehalf, and Imax values are shown in . The Eonset of all samples are plotted as a function of Tpyrolysis in ). The Eonset value linearly increased with increasing Tpyrolysis. The best ORR performance was observed in the case of CA-1/10-900°C; its Eonset, Ehalf, and Imax were 1.01 V, 0.821 V, and 4.84 mA/cm2, respectively. Notably, these values are similar to those reported for Pt/C [Citation9].
Figure 4. (a) Linear-sweep voltammograms of CAs recorded at an RRDE rotation speed of 1600 rpm in pH 13 KOH (aq). (b) Plot of Eonset as a function of the pyrolysis temperature (Tpyrolysis). (c), (d) Tafel plots of CAs pyrolyzed at 900°C and K–L plots of the corresponding samples and CAs obtained from CNF/BM = 1/10 pyrolyzed at 900°C. (f) Electron transfer number N as a function of Tpyrolysis.
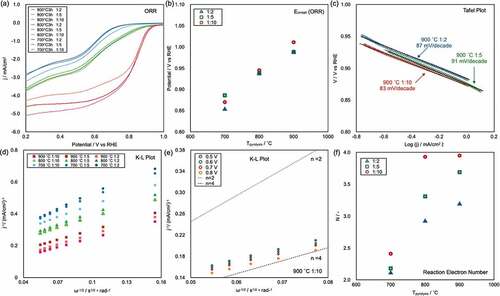
The rate-limiting process of the ORR on catalysts can be estimated from Tafel plots. The Tafel plots for the ORR on the CAs pyrolyzed at 900°C are shown in ). The Tafel slopes of the respective catalysts range from 82 to 91 mV/decade, indicating that the rate-limiting step of the ORR on these catalysts was the adsorption of O2 molecules onto active sites, which is the same rate-limiting step of the ORR on Pt/C [Citation42]. Two pathways exist for the ORR: a two-electron (2e−) process, in which O2 molecules are reduced to H2O2, and a four-electron (4e−) process, which offers complete reduction from O2 molecules to OH−. To maximize the battery performance, an electrocatalyst should catalyze the ORR via 4e− process.
) shows the K–L plots calculated via Equationequation (2)(2)
(2) using data from the linear-sweep voltammograms recorded under various RRDE rotation speeds (one method for evaluating the reaction electron number (N) of the ORR) for whole samples at 0.6 V, and ) shows the plot for the CAs prepared from CA-1/10-900°C at different potentials. The linearity of the K–L plots shows the reaction kinetics for dissolved O2 and the electron transfer numbers of the ORR. In the K–L plots of whole samples, linear slopes are observed; the slopes gradually decrease with increasing Tpyrolysis. This result indicates that the N of the ORR increased with increasing Tpyrolysis. From ), the K–L plots of CAs prepared from CA-1/10-900°C were constantly found to be close to the N = 4 line, which indicates that the ideal 4e− process was achieved on this catalyst. The N value was also evaluated using Equationequation (2)
(2)
(2) on the basis of HO− yields measured using an RRDE in 0.1 M KOH. ) shows a plot of N as a function of Tpyrolysis. The N values increase with increasing Tpyrolysis, similar to the K–L plot data.
The EDX and XPS chemical analysis results discussed in the previous section indicate that pyridinic N, P and FeN4 structures were formed in the case of CAs pyrolyzed at 900°C. Normally, pyridinic N and FeN4 structures strongly enhances the performance of ORR. In order to reveal the catalytic center of ORR, the disc electrode was immersed in 10 mM KCN solution to block Fe atom with CN. By this poisoning experiment, ORR performance decrease when the FeN4 structure is the catalytic center. From the LSV curves before and after poisoning experiment, the Eonset value slightly decreased (See supporting information, S2). Given that the pyridinic N and FeN4 structures both enhance the ORR performance, especially pyridinic N is the catalytic center. The N1s peak decreased with increasing pyrolysis temperature but ORR performance increased. This was caused by successful formation of Fe-N4 structures even though the content of N was decreased. Off cause, N-doped graphene also increase the ORR catalytic activities, but Fe-N4 structure is more sufficient for ORR catalytic activities. Therefore, ORR activities increased with increasing the pyrolysis temperature.
The linear-sweep voltammograms of the GC, BM, and CNFs pyrolyzed at 900°C were recorded using an RRDE, like the voltammograms of the other CA samples (see supporting information, S4). A comparison of the results shows that the BM-900°C and CNF-900°C exhibited better ORR performance than the bare GC electrode; however, their performance was relatively lower than that of CA-1/10-900°C. Notably, the alloyed materials exhibited the best ORR performance and the pyrolyzed original materials exhibited worse performance. ORR performance of carbon alloys from those composites showed superior performance than carbons obtained from BM or CNF, respectively as shown in the supporting information, S4. And also, the carbons pyrolyzed only from CNFs showed higher ORR performance than that of BMs. Therefore, carbon alloys from BMs had inferior performance than those two carbons and it can not be explained if only BMs contribute to the performance. These results suggest that the alloying process enhances the ORR performance.
3.3. OER performance
Linear sweep voltammograms for the OER of all the CAs are shown in ). The CAs pyrolyzed at 700°C exhibit a high Eonset; however, the CAs pyrolyzed at 800°C and 900°C show lower Eonset values (). The OER overpotential (η) values obtained from the Eonset results are plotted as a function of Tpyrolysis in ). This plot shows that the η of the CAs was not affected by the Tpyrolysis, whereas the η of the CA-1/10 series decreased with increasing Tpyrolysis; it also shows that the OER performance of the CA-1/10-900°C was prominent. The Eonset(OER) hierarchy was IrO2/C ≈ CA-1/100–900°C > Pt/C ≫ GC (see supporting information, S4). The Tafel slopes of the CA-1/2-900°C, CA-1/5-900°C, and CA-1/10-900°C specimens were 188, 159, and 124 mV/decade, respectively ()). A smaller Tafel slope normally indicates a smooth reaction during the OER, and the results strongly indicate high performance of the CA-1/10-900°C sample.
Figure 5. (a) Linear sweep voltammograms of CAs, as recorded at a rotation speed of 1600 rpm in pH 13 KOH (aq). (b) Plots of the overpotential (η) as a function of the Tpyrolysis. (c) Tafel plots of the OER on CAs pyrolyzed at 900°C.
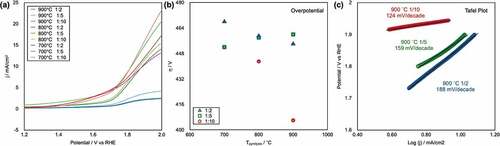
According to the literature, CAs containing heteroatoms such as N and P show high OER performance [Citation41]. Because the CA-1/10-900°C contains both N and P, and because the XPS signal of P in this CA was obviously sharp, these heteroatoms played important roles in the OER. From the XPS analysis, it is clear that P was incorporated in the carbon alloys. P should be incorporated into the graphite network therefore it is very difficult to characterize local chemical structures around P atoms, but from literatures, C-P and P-O peaks were found in the peak of narrow scan XPS spectra of P2p at 132.0 eV and 133.5 eV [Citation43]. Those peaks were also found in our results, which indicates P atoms were incorporated in the graphite network and some of them were oxidized. In addition, the OER process requires high electron conductivity of the catalysts because a high voltage is applied to the electrodes; low conductivity would result in chemical degradation of the catalysts and the electrodes. This highly developed graphitic structure of carbon alloys originated from highly crystalline structures of ascidian CNFs also contributes to the high OER performance. From the Raman scattering spectra (See supporting information, S6), broad peaks attributed to defect (D) and graphite (G) structures were found at 1,350 cm−1 and 1580 cm−1, respectively. The ratio between those two peak intensities represents quality of the carbon materials. From the Raman spectra of CA-1/10-700, 800, and 900 °C, the D/G ratio is almost constant in each case, and the ratio was c. a. 1, which means the quality of the carbon obtained from CNFs and BMs were close to commercially available carbon blacks.
3.4. Total ORR/OER performance of CAs
shows the overall linear-sweep voltammograms for the CA-1/10-700°C, −800°C, and −900°C, which provide information about both the ORR and OER properties of the CAs. These plots clearly show that Tpyrolysis = 900°C was the best pyrolysis temperature for realizing high-performance bifunctional electrocatalysts. The overall oxygen electrode activity can be evaluated by the variance of the OER and ORR metrics (ΔE = Ej=10(OER) − Ehalf(ORR)) [Citation44,Citation45]. The smaller the ΔE value, the closer the electrode to an ideal reversible oxygen electrode. The ΔE value was calculated from total linear-sweep voltammograms of CA-1/10-900°C, and the value was estimated as 936 mV (inset of ). This value is similar to that of highly active electrocatalysts that include a noble metal (e.g. Pt/C [Citation46], ΔE = 940 mV; Ir/C [Citation47], ΔE = 920 mV; see supporting information S5), transition metal (e.g. CaMn4Ox [Citation47], ΔE = 1040 mV; NixOy/N-doped C [Citation46], ΔE = 930 mV), or non-metal material (e.g. N-doped graphene/carbon nanotubes [Citation48], ΔE ≈ 1000 mV).
Figure 6. Overall linear-sweep voltammograms of CA-1/10-700°C, −800°C, and −900°C and calculation of ΔE (inset). From this plot, the potential at j = 10 mA/cm2 on the OER (Ej=10(OER)) and the half-wave potential of the ORR (Ehalf(ORR)) were determined to be 1.757 and 0.821 V, respectively. The difference between these values, ΔE, was 936 mV.
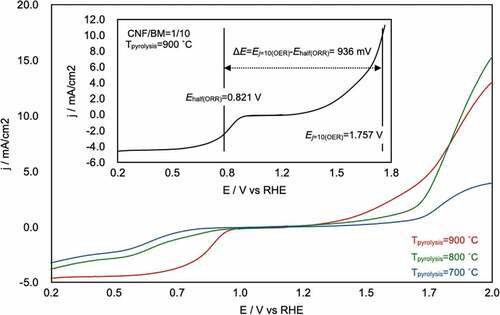
It is well-known that Fe-N4 structures show high ORR catalytic property and it was generally understood by high affinity of Fe atom surrounded by four pyridinic nitrogen atoms to oxygen molecules [Citation20]. P was assisted the adhesion of oxygen molecules in the ORR activities [Citation43]. Regarding OER, edge nitrogen atom has high affinity to OH− ions, this was also associate with the high performance of OER [Citation20].
3.5. Conclusions
In the present paper, we presented high-performance ORR/OER bifunctional electrocatalysts prepared by pyrolysis of all-biomass resources (CNFs and BM mixtures). A high pyrolysis temperature enabled the formation of heteroatom doped CAs containing N, P, and Fe. The best electrocatalyst showed high ORR/OER performance comparable with that of previously reported noble- and transition-metal electrocatalysts and CAs such as N-doped graphene. Notably, we did not use any synthetic materials when preparing the high-performance bifunctional electrocatalysts. Both the methodology developed for preparing the electrocatalysts and the electrocatalysts themselves will contribute to realizing a sustainable society by reducing CO2 emissions associated with battery production and will contribute to the high LCA of energy devices such as PEFCs, metal–air batteries, and water electrolyzers.
Supplemental Material
Download MS Word (3.6 MB)Acknowledgments
H.Y. thanks OIKAWA DENIM for providing tunicates of ascidians, which was the source used to prepare CNFs. H.Y. thanks Ms. Mayumi Sasaki at WPI-AIMR, Tohoku University for acquiring the data. H.Y. also thanks Mr. Koki Nakamura at AZUL Energy for acquiring preliminary data. This work was partly supported by NEDO (No. 2020-0115004) and KAKENHI, JSPS, Japan (Nos. 18H05482, 19KK0357, 20H04625) and “Five-Star-Alliance”, MEXT, Japan.
Supplemental data
Supplemental data for this article can be accessed here.
Disclosure statement
No potential conflict of interest was reported by the author(s).
Additional information
Funding
References
- Demarconnay L, Coutanceau C, Léger JM. Electroreduction of dioxygen (ORR) in alkaline medium on Ag/C and Pt/C nanostructured catalysts - effect of the presence of methanol. Electrochim Acta. 2004;49(25):4513–4521.
- Cao R, Lee JS, Liu M, et al. Recent progress in non-precious catalysts for metal-air batteries. Adv.Energy Mater. 2012;2(7):816–829
- He P, Wang Y, Zhou H. Titanium nitride catalyst cathode in a Li-air fuel cell with an acidic aqueous solution. Chem Commun. 2011;47(38):10701–10703.
- Yang F, Liu X, Zhang H, et al. Boosting oxygen catalytic kinetics of carbon nanotubes by oxygen-induced electron density modulation for advanced Zn-Air batteries. Energy Storage Mater. 2020;30:138–145.
- Debe MK. Electrocatalyst approaches and challenges for automotive fuel cells. Nature. 2012;486(7401):43–51.
- Gasteiger HA, Markovic NM. Just a dream—or future reality? Science. 2009;324(5923):48–50. (80-.).
- Wang Y, Wu Q, Zhang B, et al. Recent advances in transition metal carbide electrocatalysts for oxygen evolution reaction. Catalysts. 2020;10(10):1–30.
- Cheng F, Shen J, Peng B, et al. Rapid room-temperature synthesis of nanocrystalline spinels as oxygen reduction and evolution electrocatalysts. Nat Chem. 2011;3(1):79–84.
- Katsounaros I, Cherevko S, Zeradjanin AR, et al. Oxygen electrochemistry as a cornerstone for sustainable energy conversion. Angew Chem Int Educ. 2014;53:102–121.
- Lin X 2016 The kinetic and mechanism of the oxygen reduction reaction on Pt, Au, Cu, PtCu/C and CuAu/C in alkaline media Thesis
- Liang Y, Li Y, Wang H, et al. Co3O4 nanocrystals on graphene as a synergistic catalyst for oxygen reduction reaction. Nat Mater. 2011;10(10):780–786.
- Hu C, Dai L. Carbon-based metal-free catalysts for electrocatalysis beyond the ORR. Angew Chem Int Educ. 2016;55(39):11736–11758.
- Kumar A, Ibraheem S, Anh Nguyen T, et al. Molecular-MN4 vs atomically dispersed M−N4−C electrocatalysts for oxygen reduction reaction. Coord Chem Rev. 2021;446:214122.
- Qu K, Wang Y, Vasileff A, et al. Polydopamine-inspired nanomaterials for energy conversion and storage. J Mater Chem A. 2018;6(44):21827–21846.
- Abe H, Nozaki K, Sokabe S, et al. S/n co-doped hollow carbon particles for oxygen reduction electrocatalysts prepared by spontaneous polymerization at oil-water interfaces. ACS Omega. 2020;5(29):18391–18396.
- Yu YM, Zhang JH, Xiao CH, et al. High active hollow nitrogen-doped carbon microspheres for oxygen reduction in alkaline media. Fuel Cells. 2012;12(3):506–510.
- Ichi OJ, Ichi TS, Furuichi A, et al. Enhancement of oxygen reduction activity of nanoshell carbons by introducing nitrogen atoms from metal phthalocyanines. Electrochim Acta. 2010;55(6):1864–1871.
- Yasuda S, Furuya A, Uchibori Y, et al. Iron-nitrogen-doped vertically aligned carbon nanotube electrocatalyst for the oxygen reduction reaction. Adv Funct Mater. 2016;26(5):738–744.
- Cao R, Thapa R, Kim H, et al. Promotion of oxygen reduction by a bio-inspired tethered iron phthalocyanine carbon nanotube-based catalyst. Nat Commun. 2013;4(1):1–7.
- Abe H, Hirai Y, Ikeda S, et al. Fe azaphthalocyanine unimolecular layers (Fe AzULs) on carbon nanotubes for realizing highly active oxygen reduction reaction (ORR) catalytic electrodes. NPG Asia Mater. 2019;11(1): doi:10.1038/s41427-019-0154-6.
- Wang K, Wang H, Ji S, et al. Biomass-derived activated carbon as high-performance non-precious electrocatalyst for oxygen reduction. RSC Adv. 2013;3(30):12039–12042.
- Singh SK, Takeyasu K, Nakamura J. Active sites and mechanism of oxygen reduction reaction electrocatalysis on nitrogen-doped carbon materials. Adv Mater. 2019;31(13):1–17.
- Antolini E. Nitrogen-doped carbons by sustainable N- and C-containing natural resources as nonprecious catalysts and catalyst supports for low temperature fuel cells. Renewable Sustainable Energy Rev. 2016;58:34–51.
- Guo C, Li Y, Xu Y, et al. A highly nanoporous nitrogen-doped carbon microfiber derived from bioresource as a new kind of orr electrocatalyst. Nanoscale Res Lett. 2019;14(1). doi: 10.1186/s11671-019-2854-9
- He G, Yan G, Song Y, et al. Biomass juncus derived nitrogen-doped porous carbon materials for supercapacitor and oxygen reduction reaction. Front Chem. 2020;8:1–10.
- Sokolov SV, Sepunaru L, Compton RG. Taking cues from nature: hemoglobin catalysed oxygen reduction. Appl Mater Today. 2017;7:82–90.
- Zhang J, Li Q, Zhang C, et al. A N-self-doped carbon catalyst derived from pig blood for oxygen reduction with high activity and stability. Electrochim Acta. 2015;160:139–144.
- Jiang WJ, Hu WL, Zhang QH, et al. From biological enzyme to single atomic Fe-N-C electrocatalyst for efficient oxygen reduction. Chem Commun. 2018;54(11):1307–1310.
- Kim HS, Lee J, Jang JH, et al. Waste pig blood-derived 2D Fe single-atom porous carbon as an efficient electrocatalyst for zinc–air batteries and AEMFCs. Appl Surf Sci. 2021;563:150208.
- Zhang C, Antonietti M, Fellinger TP. Blood ties: co 3 O 4 decorated blood derived carbon as a superior bifunctional electrocatalyst. Adv Funct Mater. 2014;24(48):7655–7665.
- Zheng J, Guo C, Chen C, et al. High content of pyridinic- and pyrrolic-nitrogen-modified carbon nanotubes derived from blood biomass for the electrocatalysis of oxygen reduction reaction in alkaline medium. Electrochim Acta. 2015;168:386–393.
- Guo CZ, Chen CG, Luo ZL. A novel nitrogen-containing electrocatalyst for oxygen reduction reaction from blood protein pyrolysis. J Power Sources. 2014;245:841–845.
- Li W, Sun L, Hu R, et al. Surface modification of multi-walled carbon nanotubes via hemoglobin-derived iron and nitrogen-rich carbon nanolayers for the electrocatalysis of oxygen reduction. Materials (Basel). 2017;10 :564.
- Vij V, Tiwari JN, Lee WG, et al. Hemoglobin-carbon nanotube derived noble-metal-free Fe 5 C 2-based catalyst for highly efficient oxygen reduction reaction. Sci Rep. 2016;6(1):1–8.
- Saito T, Kuramae R, Wohlert J, et al. An ultrastrong nanofibrillar biomaterial: the strength of single cellulose nanofibrils revealed via sonication-induced fragmentation. Biomacromolecules. 2013;14(1):248–253.
- Ishida O, Kim D-Y, Kuga S, et al. Microfibrillar carbon from native cellulose. Cellulose. 2004;11(3/4):475–480.
- Kim DY, Nishiyama Y, Wada M, et al. Graphitization of highly crystalline cellulose. Carbon N Y. 2001;39(7):1051–1056.
- Grewal M, Matsuo Y, Yabu H. Heteroatom-doped carbon electrocatalysts prepared from marine biomass cellulose nanocrystals and bio-inspired polydopamine for oxygen reduction reaction. New J Chem. 2021;45(41):19228–19234.
- Matsuki S, Kayano H, Takada J, et al. Nanocellulose production via one-pot formation of c2 and c3 carboxylate groups using highly concentrated NaClO aqueous solution. ACS Sustain Chem Eng. 2020;8(48):17800–17806.
- Yuan H, Chen H, Li D, et al. Catalytic synthesis and simultaneous co-doping of hierarchically porous carbon with in-situ coated graphene from biomass tar as efficient catalyst for ORR. Electrochem Commun. 2019;100:52–59.
- Li XX, Zhu PY, Li Q, et al. Nitrogen-, phosphorus-doped carbon–carbon nanotube CoP dodecahedra by controlling zinc content for high-performance electrocatalytic oxygen evolution. Rare Met. 2020;39(6):680–687.
- Shinagawa T, Garcia-Esparza AT, Takanabe K. Insight on Tafel slopes from a microkinetic analysis of aqueous electrocatalysis for energy conversion. Sci Rep. 2015;5(1):1–21.
- Li J-C, Hou P-X, Cheng M, et al. Carbon nanotube encapsulated in nitrogen and phosphorus co-doped carbon as a bifunctional electrocatalyst for oxygen reduction and evolution reactions. Carbon. 2018;139:156–163.
- Ma TY, Ran J, Dai S, et al. Phosphorus-doped graphitic carbon nitrides grown in situ on carbon-fiber paper: flexible and reversible oxygen electrodes. Angew Chem. 2015;127(15):4729–4733.
- Liao Z, Wang Y, Wang Q, et al. Bimetal-phthalocyanine based covalent organic polymers for highly efficient oxygen electrode. Applied Catalysis B: Environmental. 2019;243:204–211.
- Masa J, Xia W, Sinev I, et al. Mn x O y /NC and Co x O y/NC nanoparticles embedded in a nitrogen-doped carbon matrix for high-performance bifunctional oxygen electrodes. Angew Chem Int Educ. 2014;53(32):8508–8512.
- Gorlin Y, Jaramillo TF. A bifunctional nonprecious metal catalyst for oxygen reduction and water oxidation. J Am Chem Soc. 2010;132(39):13612–13614.
- Tian GL, Zhao MQ, Yu D, et al. Nitrogen-doped graphene/carbon nanotube hybrids: in situ formation on bifunctional catalysts and their superior electrocatalytic activity for oxygen evolution/reduction reaction. Small. 2014;10(11):2251–2259.