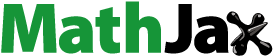
ABSTRACT
Supplementing sufficient oxygen to cells is always challenging in biomedical engineering fields such as tissue engineering. Originating from the concept of a ‘blood substitute’, nano-sized artificial oxygen carriers (AOCs) have been studied for a long time for the optimization of the oxygen supplementation and improvement of hypoxia environments in vitro and in vivo. When circulating in our bodies, micro-sized human red blood cells (hRBCs) feature a high oxygen capacity, a unique biconcave shape, biomechanical and rheological properties, and low frictional surfaces, making them efficient natural oxygen carriers. Inspired by hRBCs, recent studies have focused on evolving different AOCs into microparticles more feasibly able to achieve desired architectures and morphologies and to obtain the corresponding advantages. Recent micro-sized AOCs have been developed into additional categories based on their principal oxygen-carrying or oxygen-releasing materials. Various biomaterials such as lipids, proteins, and polymers have also been used to prepare oxygen carriers owing to their rapid oxygen transfer, high oxygen capacity, excellent colloidal stability, biocompatibility, suitable biodegradability, and long storage. In this review, we concentrated on the fabrication techniques, applied biomaterials, and design considerations of micro-sized AOCs to illustrate the advances in their performances. We also compared certain recent micro-sized AOCs with hRBCs where applicable and appropriate. Furthermore, we discussed existing and potential applications of different types of micro-sized AOCs.
1. Introduction
Oxygen is a necessary substance for aerobic cell survival and proliferation and an important factor in numerous metabolism processes and pathologies in the body [Citation1]. Owing to the limited oxygen solubility in the blood serums, culture media, and scaffold materials in tissue engineering, providing an efficient and precise oxygen supply remains a constant challenge in various biomedical fields [Citation2] such as oxygenation therapy and cancer therapy [Citation3], as well as in blood substitutes for transfusion.
Artificial oxygen carriers (AOCs) have long been studied as an approach to optimizing oxygen supplementation and improving hypoxia environments in vitro and in vivo [Citation4]. In fact, the concept of AOCs was originally derived from red blood cells (RBCs), i.e. the natural oxygen-delivering cells in whole blood. AOCs were originally developed as blood substitutes to compensate for the drawbacks of allogeneic blood transfusion, including shortages in emergency situations such as disasters and wars, limited numbers of healthy donors, complicated processing, short shelf lives [Citation5,Citation6], adverse effects such as immunosuppression and infectious disease transmission [Citation7], and religious and ethical concerns [Citation8].
Aiming to utilize the high oxygen binding and affinity of hemoglobin (Hb) in RBCs, purified stroma-free and endotoxin-free Hb from bovine or human sources became the basis material for the earliest AOCs in the 1950s [Citation9]. In the early forms of Hb-based oxygen carriers (HBOCs), the Hb was cell-free, i.e. chemically modified or crossed-linked with a molecular weight under 500 Da [Citation10]. The Cell-free Hb was administered to several US navy patients in the 1950s, but cardiovascular complications were noted as a side effect [Citation11]. In 1957, multiple materials were used to encapsulate Hb, such as nylon, collodion, gelatin, and silicone. The first Hb-loaded liposome was reported in 1977; the Hb was loaded into the phospholipids, fatty acids, and cholesterol liposomes [Citation12,Citation13].
Subsequently, chemically surface-modified (polymerized or conjugated) Hb was developed as HemAssist in 1985. Polyheme was clinically developed in 1996 as a polymerized version of Hb free of tetramer Hb [Citation7]. However, the clinical uses of early HBOCs were accompanied by many side effects including vasoconstriction, methemoglobin generation, and acute anemia [Citation14]. In view of these challenges, novel nano-seized Hb-based AOCs are now being energetically studied [Citation15,Citation16].
The other earliest type of AOCs were the perfluorocarbon (PFC)-based oxygen carriers (PFOCs) introduced by Clark and Gollan in the 1960s [Citation17]. PFCs are a group of synthetic chemicals. They both hydrophobic and lipophobic owing to fluorine’s low polarizability. Owing to the low van der Waals interactions between PFC molecules, they can dissolve a large quantity of oxygen [Citation18]. In addition, PFCs transport oxygen molecules according to Henry’s law, in which the loading and release of oxygen are controlled by the partial pressure of oxygen in the environment. The outstanding physical and chemical properties of PFCs make them effective materials for AOCs [Citation19]. In hypoxic tissues, when both PFCs and RBCs are simultaneously present in the circulation system, the PFCs release oxygen to guard the Hb-bound oxygen until it reaches the hypoxic tissues. Owing to the poor solubility of PFCs in water, Fluosol-DA (Green Cross Corp., Japan) was the first PFC nano-emulsion system to receive FDA approval in 1989. It included 20 wt.% (10.6% volume) of perfluorotripropylamine along with Pluronic F−68, egg yolk phospholipid, and potassium oleate. As a result of its short shelf life, pulmonary complications, reduced platelet, increased white blood cell (WBC) counts, and decreased neutrophil counts, it was discontinued in 1994 [Citation20]. The 2nd generation of PFOCs (such as Oxygent®) eventually met the same fates as their precedents [Citation7]. Although PFOCs have a lower solubility of oxygen than that of HBOCs under the same partial pressure, they have the advantages of being pasteurized more easily and being chemically inert to oxygen; as such, they can withstand more physical, metabolic, and chemical stimuli and can be preserved for a longer time. However, the polydispersity of previous PFOCs and toxicities of the poloxamer or phospholipids surfactants have been shown to activate the complementary system and macrophage activity, leading severe adverse reactions such as influenza-like symptoms [Citation21]. Moreover, the nano-sized PFC emulsions were rapidly cleared from circulation by the reticuloendothelial system, leading to short circulation times (half-life 6 − 12 hours) [Citation22]. Unfortunately, all of the previous HBOCs and PFCs nano-emulsion products have discontinued their manufacture or been disapproved by FDA; no reliable AOCs for replacing human blood exist in the market today. Correspondingly, greater efforts are focused on both HBOCs and PFOCs nowadays [Citation23–25].
In addition to nano-sized AOCs, micro-sized AOCs have emerged and gained profound attention. As their sizes usually range from a few to hundreds of microns, micro-sized AOCs can be distinguished from their nano counterparts in cultured tissues without internalization by cells or penetration into the extracellular matrix [Citation26]. Micro-sized AOCs can also help overcome some of the inherent limitations of nano-emulsion HBOCs and PFOCs, such as the toxicity of the surfactants and short circulation period.
Recently, several types of micro-sized AOCs have been proposed, such as microspheres, micro-sized emulsions, and microcapsules with core-shell architectures. Owing to the protective outer shell, the microcapsule structure possesses general merits such as protection of compounds in the core, controlled release of the core material, and an enhanced shelf life [Citation27]. The shell can act as a barrier between the oxygen-carrying core material and surroundings of the AOCs. Thus, we expect that high-oxygen-permeable materials will be desirable for the shell, as its thickness must be strictly controlled. In addition to providing oxygen permeability for rapid oxygen absorption and desorption, the shell can also be readily engineered in terms of its morphology, porosity, and biomechanical properties. Surface coatings or modifications (such as the conjugation of a receptor on the shell for specific site-targeting) is also possible [Citation28].
Another unique aspect of micro-sized AOCs is their potential for mimicking the morphology and rheology of RBCs. RBCs can circulate smoothly via blood capillaries and vessels owing to their extraordinary deformability and shear-thinning properties. RBC-mimicking AOCs should therefore have excellent biomechanical properties, such as in the deformability and viscoelasticity of their suspension. This will help them provide longer intravascular circulation based on the lower cellular adhesion and sufficient oxygen supply to peripheral tissues based on the better biodistribution. Then, they will have the potential to provide oxygen delivery functions similar to those of human RBCs, demonstrating, e.g. minimal accumulation in organs, non-aggregation, and sustainable oxygen supplementation. These can be particularly useful in many biomedical fields, such as perfusion culturing in vitro and transfusion alternatives in vivo.
In general, the fundamental biomaterials of micro-sized AOCs are chemicals for stabilizing or producing oxygen. The general approach is mainly based on three major oxygen-binding or oxygen-generating biomaterials: Hb, PFCs, and peroxides (POs). Their products can be categorized into four mainstream AOC types: HBOCs, PFOCs, POs, and oxygen microbubbles (OMBs). Even though those AOC types share some common techniques regarding the fabrication and oxygen delivery functions, the microparticles in each AOC category also have uniqueness in terms of their biomaterial properties, oxygen-releasing mechanisms, and limitations, making them suitable for certain biomedical applications. For instance, POs are frequently embedded in artificial scaffolds for tissue regeneration, whereas OMBs are usually injected into a targeted site for short period of oxygen therapy. shows the general formulation and oxygen-delivering strategy for each micro-sized AOC type inspired by RBCs.
In this review, we deliberately discuss the recent advances in micro-sized AOCs by summarizing the most illustrative examples of oxygen-delivering microparticles of different types from the past decade. The state-of-the-art fabrication methods, material compositions, and intended applications are evaluated and elaborated upon. Then, we provide design criteria and perspectives for the development of the next generation of micro-sized oxygen carriers.
2. Natural oxygen carriers – Red blood cells (RBCs)
Whole blood works as the medium for transporting nutrients and oxygen throughout the human body. It mainly contains a plasma medium, WBCs, RBCs, and platelets [Citation29]. RBCs comprise 40% of the blood and serve as the major oxygen-carrying vehicles. They are naturally engineered to circulate from the arterial vessels (up to ~100 µm) to the peripheral capillary vessels (down to ~5 µm) for approximately 120 days [Citation30]. A healthy RBC has a unique interchangeable morphology and core-shell (Hb-cell membrane) structure as shown in , providing it with an excellent deformability and oxygen capacity to meet the oxygen demands of each tissue and cell. Those properties are utilized by RBCs as efficient cell vehicles. Therefore, comprehension of the mechanisms and constitution of RBCs can help in the design and optimization of micro-sized AOCs.
2.1 Size and shape
As a microcapsule with a fluidic core and elastic membrane, the RBC’s shape and size is closely related to its biomechanical and physical properties, as well as the forces subjected to it from the surroundings (such as shear stresses). A healthy human RBC subjected to zero external forces has the shape of a biconcave discoidal with a size of approximately 7.5 µm in diameter and 2 µm in thickness [Citation31]. An RBC has multiple morphologies in its relaxed state, including anisocytosis (variation in size) and poikilocytosis (variation in shape). Under various pathological conditions, some of the abnormal shapes dominate and are often associated with different diseases [Citation32]. For example, shows typical shapes observed in the blood smear examinations of patients with COVID−19 [Citation33]. As another example, a decrease in the fraction of RBCs with biconcave shapes often leads to several diseases such as sickle cell anemia, which has still no effective treatments in most cases. Numerous studies have considered the conditions and indications of different RBC morphologies, but healthy RBCs can deliver oxygen the most efficiently, owing to the high deformability and high surface area of the gas exchange as they circulate through the body. Such high deformability helps the healthy RBCs morph into different shapes according to their flow conditions, leading to optimal blood rheological properties such as shear-thinning (which helps them pass through capillaries without occlusion).
Figure 3. Typical abnormal RBC morphologies observed in the peripheral blood samples from COVID − 19 patients, adapted with permission from [Citation33]. Copyright 2022 Marchi, Bozzini, Bertolone, Dima, Busti, Castagna, Stranieri, Fratta Pasini, Friso, Lippi, Girelli and Vianello.
![Figure 3. Typical abnormal RBC morphologies observed in the peripheral blood samples from COVID − 19 patients, adapted with permission from [Citation33]. Copyright 2022 Marchi, Bozzini, Bertolone, Dima, Busti, Castagna, Stranieri, Fratta Pasini, Friso, Lippi, Girelli and Vianello.](/cms/asset/4f8ee40e-4c7b-44f0-86c8-c97c9c074ec7/tsta_a_2223050_f0003_oc.jpg)
In view of the lessons learned from the sizes and shapes of human RBCs (hRBCs), the morphology consistency and size uniformity of the particles may become important to the design of micro-sized AOCs for clinical use.
2.2. Microcirculation
The human body contains a complex capillary network where blood flows constantly while carrying its vehicle components. Blood, as a non-Newtonian fluid, can flow steadily through complex microvascular structures owing to its shear-thinning behaviors [Citation34]. The viscosity of the blood is dependent on various components such as hematocrit and plasma, as well as the viscoelastic properties of formed elements [Citation35]. As RBCs comprise the most abundant formed elements in the blood, the viscosity of blood increases almost linearly with the hematocrit [Citation36]. Even in the narrowest blood capillaries of 3–4 m, RBCs can still pass without impediment owing to the deformability given by the cell membrane as supported by the spectrin network on their surface (as mentioned in the earlier section). Owing to this deformability, RBCs are not constricted in their shape as they face high shear stresses and intracapillary resistance; they deform themselves into different shapes (including parachutes and slippers) at different velocities [Citation37].
Notably, however, obstructions can occur during RBC circulation through the microvasculature when the integrity or morphology of the RBCs is affected by hemolysis, inflammation, adhesion, and damage. As one of the most famous examples, sickle cell disease alters the rheology properties of blood by impairing the RBCs’ ability to deformation. As a result, the viscosity of the blood increases, leading to a loss of microvascular function [Citation38]. Thus, the physical and mechanical properties of RBCs are tightly related to their behaviors in microcirculation. The rigidity of RBCs has been proven to affect their intracapillary transport resistance, pulmonary arterial pressure, and oxygenation [Citation39–41]. In general, the balance of the oxygen capacity and stiffness of RBCs are naturally optimized so that they can continuously release oxygen during microcirculation. The microcirculation phenomenon has been extensively studied; in this context, microfluidics have been demonstrated as an effective approach to accurately simulating the microcirculation of RBCs [Citation42]. RBC capillary models would also be useful in the design of the parameters for micro-sized AOCs (such as their shape and softness) to obtain the desired rheological properties for circulation in capillary-size vessels.
2.3 Storage as packed red blood cells (PRBCs)
Traditionally, whole blood is processed and stored in blood banks for future transfusion after collection from donors. To eliminate febrile and allergic reactions and improve the storage life, the general prepossessing includes anticoagulation treatment, pathogen reduction, WBC reduction (leukoreduction), and other specialized storage procedures [Citation43–45]. Nevertheless, the expiration of unseparated whole blood remains relatively fast, i.e. in the range of 20–30 days when stored at 2–6°C in a refrigerator (depending on the anticoagulant type) [Citation46].
Alternatively, the ex vivo storage of whole blood can be replaced using packed RBCs (PRBC), where 70% of the plasma is removed by centrifuge. The storage life of an PRBC can be prolonged to 42 days at 1–6°C [Citation47]. PRBCs are highly concentrated RBCs without plasma; thus, the probability of a transfusion-associated circulatory overload is lower than that with a whole blood transfusion [Citation48]. Leucodepletion of PRBCs is also recommended before transfusion to prevent primary alloimmunization to human leukocyte antigens; however, this significantly increases the cost [Citation49]. In addition, different anticoagulant or processing techniques during the separation and processing of RBCs from the whole blood may have various adverse effects on RBC oxygen-carrying functionality (e.g. affecting morphology changes and hemolysis) [Citation50,Citation51].
Furthermore, many dysfunctional elements can accumulate during the storage of PRBCs, including red blood cell-derived microparticles, aggregated Hb, band 3, and lipid raft proteins, which are biomarkers for many types of diseases [Citation52–54]. The causes of RBC degradation are progressive with the hypothermic storage duration and are often collectively referred to as RBC storage lesions, leading to increasing Hb denaturation and various physiological impacts on the transfusion recipients [Citation55]. Moreover, recent studies have shown that using older PRBCs can increase the coagulation potential and lead to hemorrhagic shock [Citation47]. After all, donor-related variables inevitably induce variability in the RBC component quality and play a role in the uncertainty of the blood storage life [Citation56]. All of the evidence from the related laboratory and clinical studies suggests that greater efforts needed to be spent on developing more advanced technologies for collecting, processing, and storing either whole blood or PRBCs to make transfusion safer and more cost-effective. The complex handling and storage of PRBCs and whole blood altogether have contributed to the high prices of blood transfusion for patients. Though the procedures and resources in each country and facility are different, in United States hospitals, the average cost of blood transfusion increased from an already high estimation of $155 per unit in 1990s [Citation57] to approximately $300 per unit in recent years [Citation58].
In comparison to PRBCs, AOCs (and especially AOCs with core-shell structures) have shown much longer shelf lives depending on the type owing to the simplicity of the composition, stability, and sterilizability, contributing to their biochemically inert shells. These facts emphasize the value of micro-sized AOCs as potential RBC alternatives in the future.
3. Fabrication methods for micro-sized artificial oxygen carriers (AOCs)
The advances in micro-sized AOCs also reflect the maturity and development of novel fabrication methods for achieving the desired morphology with mono-dispersity. The major morphologies of micro-sized AOCs are microfibers, microparticles, and microcapsules. No standards exists for selecting techniques for different types of AOCs. In general, each method has advantages and limitations. In this section, we focus on recent advances in the technical aspects and unsolved issues in preparing micro-sized AOCs. Understanding the development of preparation methods based on past studies can help researchers decide the appropriate fabrication technologies for the next generation of micro-sized AOCs.
3.1 Coprecipitation and chemically crosslinking of hemoglobin (Hb)
Micro-sized HBOCs are often developed by chemical crosslinking. Hb was first precipitated by salts and micro-sized Hb particles were fabricated by subsequent chemical crosslinking, as shown in . The salts were removed by dissolution and glutaraldehyde was commonly used for the crosslinking [Citation59]. In another study, albumin was determined as biocompatible and suitable for dispersing particles; it was used for coprecipitation with Hb, as shown in [Citation61]. Kai et al. [Citation62] encapsulated Hb within a crosslinked bovine serum albumin (BSA) shell, where the cross-linkage of the albumin proteins was induced by genipin as previously described by Butler et al. [Citation60]. Li Li D et al. coated the surfaces of 4 μm-sized AOCs prepared by the coprecipitation method with polyethylene glycol (PEG) [Citation63], as shown in .
Figure 4. Fabrication of AOCs by coprecipitation and subsequent chemical cross-linking. (a) Fabrication scheme of hemoglobin microparticles, reprinted with permission from [Citation58]. Copyright 2012, American Chemical Society (b) Fabrication scheme of Hb particles, reprinted with permission from [Citation59]. Copyright 2013, American Chemical Society (c) Schematic representation of the assembled Hb microspheres with the surface modified by PEG, reprinted with permission from [Citation60]. Copyright 2012, American Chemical Society.
![Figure 4. Fabrication of AOCs by coprecipitation and subsequent chemical cross-linking. (a) Fabrication scheme of hemoglobin microparticles, reprinted with permission from [Citation58]. Copyright 2012, American Chemical Society (b) Fabrication scheme of Hb particles, reprinted with permission from [Citation59]. Copyright 2013, American Chemical Society (c) Schematic representation of the assembled Hb microspheres with the surface modified by PEG, reprinted with permission from [Citation60]. Copyright 2012, American Chemical Society.](/cms/asset/f94d6e5d-92c4-4a92-abe9-b2b10b0506e4/tsta_a_2223050_f0004_oc.jpg)
3.2 Electrospinning/Electrospraying
Electrospinning and electrospraying are electrohydrodynamic processes in which single or multiple electrically charged polymer solution jets are sprayed or spun for the formation of nano-sized to micro-sized fibers and particles, respectively [Citation64]. Usually, the particles or fibers form and solidify and are collected in an ultrasonic aqueous bath before solvent evaporation. This technique has been extensively used in the last decade to prepare AOCs. Single axial electrospinning or electrospraying is used to generate microfibers or microparticles with single phases. For instance, Erlane et al. [Citation65] produced a polycaprolactone (PCL) microparticle loaded with nano-sized calcium PO (CPO) oxygen-generating microparticles by electrospraying at a positive voltage of 12 kV. Those microparticles ranged in size from 5 to 15 μm and were later encapsulated together with cells in the hydrogel for enhanced cell expansion. Similarly, Sajedeh et al. [Citation66] fabricated polylactic-co-glycolic acid (PLGA) microparticles loaded with nano-CPO with average diameter of 5.3 μm by electrospraying at a constant voltage of 15 kV. Notably, the electrosprayed PLGA/CPO microparticles presented a biconcave disk-like morphology in spite of a wide size distribution. Morais et al. [Citation67] prepared mono-dispersed PCL/CPO microparticles with diameters of 17.0 ± 0.3 μm at a positive voltage of 12kV.
Coaxial electrospinning and electrospraying represent novel strategies for fabricating core-shell micro-sized AOCs. In addition to a single nozzle, Zhang et al. [Citation68] also employed a coaxial nozzle to prepare both single- and double-walled PCL/CPO microparticles with diameters of 26.4 ± 5.1 μm using coaxial electrospraying. Compared with the electrosprayed single-walled PCL/CPO microparticles, the double-walled structure provided an additional barrier to the outside water and slowed down the release of oxygen. Recently, Ma et al. [Citation69] fabricated a perfluorotributylamine (PFTBA)-PCL core-shell microfiber system using coaxial electrospinning with concentric spinnerets with an outer needle diameter of 1.2 mm and inner needle diameter of 0.3 mm to prolong the oxygen release of the PFTBA for nerve regeneration. A maximum positive voltage of 16 kV steadily generated core-shell microfibers with diameters of 7.5 ± 2.2 μm and 3.2 ± 2.4 μm.
Although electrospraying and electrospinning have the advantages of a high encapsulation rate and high production rate, one typical issue is the difficulty in controlling the size. In recent examples of produced micro-sized AOCs, the experimental conditions were individually optimized without a standard guideline. Several parameters can be optimized in the processes of electrospinning or electrospraying, such as the voltage, flow rate, and height. Among those parameters, a high voltage ranging from 5 kV to 20 kV has often been required to drive a polymer solution ejected at a constant speed; this has correspondingly been limited by machine strength and safety concerns. Therefore, future developments in electrodynamic processing in terms of the fundamental equipment such as the quality of micronozzle array [Citation70] are likely to improve the overall production quality of micro-sized AOCs. Moreover, coaxial electrodynamic processes are interesting because they can be arranged to produce microparticles with multiple layers [Citation71]; this can help in the design of novel micro-sized AOCs with multi-layered structures with potentially advanced properties.
3.3 Mechanical emulsification
Emulsification is one of the simplest and most widely used methods for the preparation of AOC emulsions from nano- to micro-sizes. It was used as early as in the 1st generations of HBOCs and PFOCs [Citation8]. To produce AOCs, an oil-in-water single emulsion and water/oil/water double emulsion are usually formed to produce the microparticles and microcapsules, respectively. When preparing AOCs composed of polymeric biomaterials (such as polymer-shelled microcapsules), solvent evaporation is often applied after the emulsification process [Citation72]. The interfacial tension between the phases plays a critical role in the size distribution, dynamic formation, and encapsulation rate of the emulsion droplets. Surfactants consequently become an important factor, because they can lower the interfacial tension between the oil phase and water phases, as well as prevent the coalescence of the microdroplets [Citation73]. Recently, Fu et al. [Citation74] studied the effects of the Pluronic surfactant type and concentration on preparing micro-sized PFC emulsions. They consequently determined the appropriate surfactant for stabilizing a micro-sized PFC emulsion with narrowest size distribution and highest stability. Zarzar et al. [Citation75] precisely controlled the droplet morphology in a perfluorocarbon-in-hydrocarbon-in-water or hydrocarbon-in-perfluorocarbon-in-water complex emulsion system from a Janus shape to a core-shell by changing the surfactant concentration, type, and temperature. Thus, they suggested the possibility of developing PFOC microcapsules with customized shapes by emulsification in the future. Another common emulsification parameter especially important in double emulsions is the phase composition or concentration ratio of each species. Nejati et al. [Citation76] studied the influences of process parameters on micro-sized poly (l-lactic acid) (PLLA) loaded with a polyvinylpyrrolidone/hydrogen PO (PVP/H2O2) mixture. They tested the effects of different concentrations of PLLA, molar ratios of H2O2 to PVP, and phase volume ratios (from aqueous to organic) on the size, encapsulation rate, loading amount, and initial burst release rate. The results helped them to design a multi-optimization strategy for fabricating the desired microcapsules by emulsification.
Nevertheless, remaining surfactant molecules on the particle surface can occasionally cause side effects and aggregations of particles; thus, minimizing the use of surfactant has becomes a challenging study topic in the manufacturing of AOCs.
3.4 Shirasu-porous-glass (SPG) membrane emulsification
Membrane emulsification is another effective strategy for controlling the sizes of microparticles or microcapsules by applying a constant pressure to force dispersed permeation through the membrane pores to the continuous phase [Citation77]. In particular, Shirasu-porous-glass (SPG) membrane emulsification is frequently used to produce microcapsules or microspheres with precise size control in the areas of food science and AOCs. Besides its high encapsulation rate and narrow size distribution, SPG membrane emulsification also has the advantages of high productivity, low energy costs, and a wide span of pore sizes [Citation78]. In recent years, Lai et al. [Citation79,Citation80], Fu et al. [Citation74,Citation81], and Hashimoto and Ohta et al. [Citation82] successfully prepared mono-dispersed micro-sized PFOCs and HBOCs with sizes under 10 m using SPG membrane emulsification. Although it has been repeatedly reported that the size of the microparticle is linearly correlated to the membrane pore size between 1μ m and 20 μm, the actual dependency varies according to the membrane type, material, and solvent system [Citation83,Citation84]. After the fabrication of core-shell particles, isopropanol (IPA) can be used to induce shape changes to fabricate concaved deformable PFC-based oxygen carriers with similar sizes as RBCs and a narrow size distribution, as shown in . The concave-shaped PFOCs have a lower elastic modulus and higher flexibility than the original microparticles. Although the shape change has been hypothesized as being owing to partial fluidization owing to the sorption of IPA by the poly(lactic acid-co-ɛ-caprolactone) (PLC) polymer shell, further research into the actual mechanisms of the shape change is expected.
Figure 5. Preparation of AOCs by SPG membrane emulsification. (a) Hb/BSA microspheres reprinted with permission from [Citation79]. Copyright 2015 Elsevier B.V. (b) PLC shell/PFOB core microparticles reprinted with permission from [Citation73]. Copyright 2019 American Chemical Society.
![Figure 5. Preparation of AOCs by SPG membrane emulsification. (a) Hb/BSA microspheres reprinted with permission from [Citation79]. Copyright 2015 Elsevier B.V. (b) PLC shell/PFOB core microparticles reprinted with permission from [Citation73]. Copyright 2019 American Chemical Society.](/cms/asset/7df1fecf-4274-42a4-a201-21c453d57373/tsta_a_2223050_f0005_oc.jpg)
3.5 Flow focusing
Flow focusing has typically been used in the settings of microfluidic or T-junction devices to produce microdroplets based on Plateau – Rayleigh instability, with the advantage of a highly uniform size [Citation85]. The production of OMBs by flow- focusing techniques has also been applied as post-processing after a sonification preparation of microbubbles to control the size range of and shell thickness of the synthesized OMBs [Citation86]. Elena et al. [Citation87] showed success in generating mono-dispersed, non-coated, air-filled microbubbles by simple flow-focusing via a modified microfluidic device. However, the microbubbles were not stabilized by surfactants or phospholipids for practical uses as AOCs.
Until very recently, only a few studies had applied flow focusing techniques to microfluidic chips to manufacture OMBs specifically for oxygen delivery purposes. Ashwin et al. [Citation88] used a microfluidic device to produce microbubbles coated with different membranes on demand and in real time. Their device incorporated flow focusing geometry and could control the hemodynamic parameters, such as the blood saturation and blood oxygen tension in real-time by instantly adjusting the infused oxygen concentration.
A common drawback of the microfluidic flow focusing technique is the high cost of the dedicated microfluidic chips used as focusing chambers. Coaxial flow focusing (CFF) is an emerging technique which can be applied in the preparation of core-shell micro-sized AOCs. In this technique, two phases (liquid-liquid or liquid-gas) from feed tubes meet and pinch-off at the capillary exit of a concentric needle. Recently, Ma et al. [Citation89] applied a novel gas-driven CFF process to obtain PFC-core, lipid-shelled, and indocyanine green (ICG)-loaded microcapsules with a diameter of 37.5 ± 4.3 μm. The coaxial needle assembly was manufactured by laser welding technology including an inner needle of inner diameter = 0.4 mm and an outer needle of inner diameter = 1.0 mm. When the outer liquid solution (lipid suspension) and inner liquid solution (IGC dissolved PFC solution) were forced by a constant pressure of 1.5 kPa, a stable cone-jet was formed after passing the small orifice of diameter = 0.6 mm and spontaneously broke into the microcapsules with nearly 100% encapsulation and narrow size distribution. Meanwhile, Xu et al. [Citation90] applied a similar process to produce micro-sized core-shell PFOCs with lipid shell and LLL12 loaded PFC-core, in the size range of 4.9 ± 1.5 μm. In this study, they discovered the relationship between average droplet size and inner phase flow rate, outer phase flow rate, and focusing phase pressure difference, which was correlated to Laplace pressure.
One year later, Liu et al. [Citation91] used a liquid-driven CFF process to form mono-dispersed ethoxylated trimethylolpropane triacrylate (ETPTA)-shell, Hb-core microdroplets of with a diameter of 32.8 ± 1.8 μm. Inside the pressure chamber, a focusing aqueous phase containing the surfactant poly(vinyl alcohol) (PVA) drove the inner liquid phase (purified Hb in phosphate-buffered saline) and outer oil phase (ETPTA in ethanol) to produce the core-shell micro-sized HBOCs.
Both single and coaxial CFF techniques share the advantage of a narrow size distribution, whereas the latter usually has a lower cost and is suitable for producing core-shell micro-sized AOCs. If the diameter of the products can be controlled by customizing the parameters of the needles and flows, CFF techniques will be useful for producing RBC-mimicking AOCs with core-shell structures in the future.
3.6 Layer-by-layer method using template particles
To fabricate RBC-mimicking oxygen carriers or RBC-mimicking hollow templates potentially capable of loading oxygen, the main technical challenge concerns the fabrication of concave or biconcave microcapsules with moderate size distributions and desired biomechanical properties. This usually requires more sophisticated preparation techniques or post-possessing of the microparticles. The research trends regarding RBC-mimicking templates helped advance those for RBC-mimicking oxygen carriers in the past decade as tools for studying blood particulate analogue fluids [Citation92]. For example, techniques used to fabricate RBC-mimicking templates also succeeded later in preparing RBC-mimicking oxygen carriers.
‘Layer-by-layer’ (LbL) technology utilizes the attraction of the opposite surface charges between the template core and layer and between each layer to deposit one or multiple layers on a template core [Citation93]. Then, the template core coated by the layers can be removed by dissolution, resulting in hollow microcapsules [Citation94]. The shape of the template core largely determines the morphology of the final product. Nishit et al. [Citation95] used electrospinning and an LbL treatment to prepare RBC-shaped HBOC particles with a diameter of 7.2 μm from their original microspherical morphology, as illustrated in . After synthesis of mono-dispersed PLGA microspheres by electrospinning, the PLGA templates were incubated in 2-propoanl to induce shape changes. After a subsequent LBL coating of nine layers of Hb/BSA and poly(allylamine hydrochloride)/BSA (PAH/BSA) on the PLGA discoidal template, the resultant microcapsules were incubated in a cosolvent of tetrahydrofuran and 2-propoanl to remove the PLGA core for the formation of hollow microcarriers. These microcarriers had an elasticity of 93 ± 42 kPa, similar to that of RBCs. Shaillender et al. [Citation96] applied the LBL technique to produce a RBC-mimicking carrying system by taking erythrocyte ghosts as templates and coating them with biocompatible polyelectrolyte layers. Their products maintained the biconcave shape of the RBCs to a great extent with a highly uniform diameter of 6.7 μm and thickness of 2.8 μm. The pre-loading of oxygen-generating or oxygen-releasing agents to the erythrocyte ghost templates could potentially make these microcapsules micro-sized AOCs. Recently, Guo et al. [Citation97] synthesized rebuilt red blood cells (RRBCs) with modular designs using more complicated LbL approach with a similar RBC ghost as the template. Owing to the modular constructure of the RRBCs, Hb could be easily loaded into the RRBCs for oxygen delivery.
Figure 6. Fabrication process of RBC-mimicking micro-sized AOCs from PS template by LbL method, adapted with permission from [Citation94]. Copyright 2009 National Academy of Science.
![Figure 6. Fabrication process of RBC-mimicking micro-sized AOCs from PS template by LbL method, adapted with permission from [Citation94]. Copyright 2009 National Academy of Science.](/cms/asset/b620936f-a2ec-4eea-bc9e-37855094cd93/tsta_a_2223050_f0006_oc.jpg)
Instead of using RBC derivatives as a template, She et al. [Citation98] prepared discoidal shaped multilayer microcapsules with a diameter of 6.7 ± 0.5 μm and thickness of 2.8 ± 0.5 μm using a covalent LbL assembly of deprotonated PAH and glutaraldehyde onto a discoidal Ca(OH)2 template. Dextran sulfate was added to change the prismatic Ca(OH)2 particles into a biconcave discoidal shape as the desired template and then were dissolved by HCL after the assembly of 10 layers of PAH. Yu et al. [Citation99] utilized the same dextran-induced shape change on an inorganic Ca(OH)2 template to fabricate biconcave RBC-sized HBOCs by coprecipitation of Hb on the Ca(OH)2.
Solvent-induced shape changes have been used in effective strategies for processing microparticles or microcapsules into concave shapes, and to fabricate polymer or inorganic templates into discoidal shapes (as in the above-mentioned studies). For instance, 2-proponal was hypothesized to induce the collapse of PLGA microspheres by the partial solubilization and fluidization of the core [Citation95]. In spite of the simple immersion or incubation procedure, the mechanisms of the shape changes have yet not been fully interpreted, and the choice of solvent remains highly specific to the template material.
3.7 Particle replication in non-wetting templates (PRINT)
The ‘Particle Replication In Non-Wetting Templates’ (PRINT) technique is an imprint-based lithographic technique which utilizes elastomeric, low-surface-energy fluoropolymer molds on a high-surface-energy sheet, allowing for the molding of monodisperse and shape-specific particles [Citation100]. This technique is promising for fabricating the desired shapes of microcapsules owing to its highly customized mold. Merkel et al. [Citation101] first used PRINT to fabricate hydroxyethyl acrylate hydrogel microparticles to mimic the shape and elastic properties of RBCs. By adding different amounts of cross-linker to the hydrogel solution, they adjusted the rigidity of the resultant hydrogel particles from 63.9 to 7.8 kPa, similar to the rigidity of RBCs. Owing to a meniscus formed during the filling of the polymer solution in a mold with size of 2–3 μm, the hydrogel particles swelled to discoidal shapes after hydration with diameters of 5.2 to 5.9 m and thicknesses of 1.2 to 1.5 μm (similar to those of RBCs). Chen at al [Citation102]. extended this method to fabricate RBC-mimicking HBOCs by incorporating bovine Hb into a PRINT-prepared RBC-mimicking microgel. They utilized a similar procedure to prepare triethylene glycol acrylate (TEGA) and 2-carboxylethyl acrylate crosslinked hydrogel particles by using patterned wells with a diameter of 2 μm and thickness of 0.6 μm. After the swelling and transfer to a PBS solution, the hydrogel hydrated to approximately 6.3 μm in diameter and 1.8 μm in thickness, with a narrow size distribution. Although PRINT techniques can produce nanoparticles or microparticles with consistent sizes and shapes for encapsulating small-molecule drugs, RNA, and AOCs, the yield is directly limited by the number of mold cavities.
4. Innovative designs and functions of recent micro-sized AOCs
Based on the understanding of RBC mechanisms, certain micro-sized AOCs have shown improved performance in different aspects in recent years. Various parameters can contribute to innovations in the micro-sized AOCs, including discoveries of new materials and the fabrication of sophisticated morphologies and decorations on the surfaces of microparticles. Below, we discuss the novel design and functions of a few groups of micro-sized AOCs (such as Hb- and PFC-based AOCs) and others, along with the resulting improvements in circulation, oxygen-releasing profiles, and biocompatibility through illustrative examples.
4.1 Hb-based micro-sized AOCs
Owing to Hb’s excellent oxygen-carrying ability, HBOCs have remained as the largest topic in either nano-sized or micro-sized AOCs for decades. The weight percent of Hb molecules in an RBC is approximately 33%; this can also act as a target for the encapsulation rate of Hb in micro-sized HBOCs.
The sources of Hb are divided into human and animal (commonly bovine and marine). The corresponding Hb products vary in terms of the oxygen capacity, affinity, stability, and other chemical properties [Citation14]. The extracted Hb molecules are usually chemically modified to prevent protein decomposition, which may cause toxicities to organs such as the kidneys [Citation103]. Hb with a large molecular weight can be obtained by crosslinking and polymerization to prevent the molecular conversion from a single Hb to its dimer form [Citation104]. To further increase the intravascular retention, the modified or crosslinked Hbs can be conjugated to or encapsulated into lipid and polymer shells to form Hb-encapsulated microparticles.
Another strategy encapsulates Hb without chemical crosslinking. To achieve effective encapsulation of Hb molecules, this strategy requires a suitable supporting material able to sustain the Hb core without affecting the oxygen transportation performances, in addition to avoiding other side effects. Phospholipids have been studied for the preparation of artificial RBCs but are very expensive. Thus, alternative biodegradable polymers have been studied for encapsulation, such as polylactide (PLA), PEG, polyethylene oxide (PEO), and PCL [Citation105]. Moreover, amphiphilic copolymers based on combinations of these biodegradable polymers (PEO/PLA, PEO/PDB, PEG/PLA, PEG/PLC, etc.) have be used to encapsulate Hb, superoxide dismutase catalase, and methemoglobin reductase while self-assembling and avoiding protein absorption. Those diblock amphiphilic copolymers can also degrade into harmless species and form polymersomes or micelles in the body fluid with similar micellar structures and sizes as those of liposomes [Citation106]. In view of the above advantages, new HBOC microcapsules based on amphiphilic copolymers have been developed in recent years. Sun et al. [Citation107] encapsulated carbonylated Hb molecules into a polypeptide platform made from poly(l-lysine)-block-poly(l-phenylalanine) diblock copolymers. The core-shell microparticles had a spherical morphology with a mean diameter of approximately 4 to 5 μm; the Hb content reached 32 wt.%. Wang et al. [Citation108] formed polymeric HBOC micelles with triblock copolymers of poly(ethylene glycol)-block-poly(acrylic acid)-block-polystyrene (PEG-b-PAA-b-PS) for photodynamic therapy. In their novel approach, the PEG-b-PAA-b-PS copolymer could self-assemble into polymeric micelles and the Hb was covalently conjugated by the carbodiimide chemistry. Then, zinc phthalocyanine was encapsulated into the HBOC micelles as a photosensitizer, resulting in a polymeric photodynamic therapy agent with an oxygen self-compensating ability.
As reported by several researchers, proteins also show potential for creating oxygen carriers owing to their longer stability, biocompatibility, biodegradability, amphipathic nature, and half-life [Citation109]. Albumin has become another candidate for the shells of micro-sized HBOCs owing to its high affinity to hydrophobic Hb molecules [Citation110]. For instance, Lai et al. [Citation80] and Ohta et al. [Citation82] used a similar strategy to fabricate bovine Hb-albumin (bHb-BAS) and human Hb – albumin (hHb-HAS) microspheres with narrow size distributions, respectively. They crosslinked bHb-BAS and hHb-HAS emulsions based on glutaraldehyde and formed mono-dispersed micro-sized HBOCs showed high resistance to metHb formation and an Hb weight ratio of 10 wt.% to 20 wt.%, respectively. Although glutaraldehyde is the most commonly used crosslinking agent, excess glutaraldehyde is toxic and requires efforts for removal. Recently, Kai et al. [Citation62] developed micro-sized HBOCs encapsulated by genipin-linked BSA shells, yielding an optimal encapsulation rate of 40–45 wt.% for the crosslinked Hb.
Owing to the thermodynamically reversible nature of the Hb and oxygen binding reaction, the photothermal effect induced in the light-responsive particles by near-infrared irradiation makes controllable oxygen delivery possible with HBOCs. Liu et al. [Citation111] utilized typical light-responsive particles, i.e. molybdenum disulfide quantum dots (MoS2 QDs), to control the oxygen release from HBOCs to the targeted area in tissue. For this purpose, the Hb and MoS2 QDs were incorporated into a gelatin methacrylate (GelMA) inverse opal (GelMA is a microcarrier gel with an ordered porous structure). Similarly, Zhang et al. [Citation112] developed Hb and light-responsive black phosphorus quantum dots-loaded GelMA microneedles for accelerating oxygen release by controlling local temperature increases on a skin. However, the current photothermal control approach still has limitations. For example, it can only shift the binding equilibrium one-way to unbinding, i.e. it cannot suppress the oxygen release.
Notably, Wang et al. [Citation113] recently developed a novel design for a micro-sized HBOC (Hb-HKUST−1) with porous metal-organic frameworks (MOFs), with HKUST−1 as core and Hb as the shell. Owing to the Hb-HKUST−1, this design had an eight times larger oxygen capacity than that of pure Hb and a longer oxygen retention time of approximately 16 hours. Although this new micro composite still has certain potential issues owing to its irregular shape, low deformability, and unknown stability, it may suggest the utilization of MOFs for oxygen delivery in vitro and in vivo as a new area for AOCs.
4.2 Perfluorocarbon (PFC)-based micro-sized AOCs
PFOCs are the second most investigated type of AOCs. Although the oxygen solubility of PFCs does not equal that of Hb, they are much easier to process, stabilize, and incorporate into microcapsules, owing to their chemically inert nature. An et al. [Citation114] first developed PFC-loaded microparticles as micro-sized PFOCs by encapsulating mixed perfluorodecalin and perfluorotripropylamine droplets into a PEG diacrylate-based hydrogel. Nevertheless, the encapsulation rate of the PFC nanoemulsions in the gel microparticles was generally very low, e.g. at 0.12%. This is because oxygen rapidly diffuses from the PFC emulsion as radicals and interferes with the UV-initiated polymerization of the hydrogel microparticles. This issue was overcome by the encapsulation of PFC molecules directly in the polymeric shell by demulsification, significantly increasing the encapsulation rate. In recent years, most studies have fabricated micro-sized PFOCs with core-shell structures by using PEG, PCL, PLC, PLGA, L-a-phosphatidylcholine, and other polymers as shell materials. Instead of simply providing stabilization using the surfactants on the droplet surface, polymer-shelled microparticles require much less surfactant and can avoid most of the surfactant molecules on the surface by centrifugation after their production. Thus, the core-shell structured PFOCs have higher biocompatibility, a longer oxygen-releasing time, and a longer shelf life. For example, Lee et al. [Citation115] developed PCL/perfluorooctane (PCL/PFO) core-shell oxygen carriers with sizes of 100 to 500 m and an average shell thickness of 10
m. Under a hypoxia condition of 1% oxygen, the PCL/PFO microcapsules could release sufficient oxygen through the porous membrane for cell proliferation for t3 days (>5.0 mg/L) and were exhausted by 10 days, demonstrating the ability to prolong cell survival until the ingrowth of new blood vessels. Later, the same group seeded human bone marrow-derived mesenchymal stem cells uniformly on the same PCL/PFO system and proved a significant increase of survival in stem cells survival adhered to the microcarriers owing to their sustainable oxygen release. This research demonstrated the potential of using micro-sized AOCs to aid stem cell therapy [Citation116].
PLC is well-known as a thermoplastic biodegradable elastomer with the potential to act as a tissue engineering scaffold (e.g. for a blood vessel, esophagus, or bladder) owing to its elastomeric properties [Citation117–119]. Fu et al. [Citation81] utilized PLC to produce PLC/perfluorooctyl bromide (PLC/PFOB) microcapsules called concave-shaped deformable PFC-based oxygen carriers. These showed high biocompatibility and deformability owing to their elastomer PLC shells. They passed through silicon microchannels with a width of 4.5 μm despite having sizes larger than 6 μm, i.e. similar to those of RBCs. It will become important to mimic the excellent deformability of RBCs to utilize micro-sized AOCs.
4.3 Other micro-sized AOCs
POs and OMBs are two other rising types of oxygen carriers. The utilization of solid or liquid peroxides as oxygen-generating biomaterials to facilitate tissue regeneration has risen in recent decades. This type of oxygen carrier has a unique property in that they do not require the absorption of the ambient oxygen as they work. The mechanism of oxygen generation of a PO is straightforward; they react with water to produce hydroperoxide, which decomposes into water and oxygen. However, as water is always abundant in the environment, the reaction is very fast and uncontrolled; thus, the resultant volatile oxygen bubbles and radicals can cause cell death [Citation120]. This problem is perfectly solved by the encapsulation of the PO particles in a hydrophobic polymer as barriers to oxygen permeation to slow down the oxygen generation rate and prolong the half-life of the release time. Therefore, PO-based, polymer-covered microparticles are frequently embedded or crosslinked in scaffolds or gels to provide sustainable oxygen to cells [Citation65,Citation121–123].
According to the reaction kinetics, the oxygen capacity and release rate of metal PO-loaded micro-sized AOCs are proportional to the PO concentration in the microparticle. However, a high concentration of PO could increase the formation and accumulation of intermediates such as H2O2 and result in undesirable cytotoxicity [Citation124]. To eliminate the side effects owing to reactive oxygen species, catalase (an antioxidant enzyme) can be used to accelerate the decomposition of H2O2 [Citation125]. For example, Mohseni-Vadeghani et al. [Citation126] recently immobilized catalase on the surfaces of CaO2-loaded PLLA microparticles and CaO2/PPLA core-shell microparticles as oxygen carriers. They showed that a 2:1 weight ratio of catalase to microspheres with a catalase grafting efficiency of 17.1% was optimal for increasing the cell viability by speeding up the H2O2 decomposition. Furthermore, they showed different oxygen and calcium ion release profiles from the PO-filled microparticles and core-shell microparticles owing to the structural discrepancies.
OMBs are micro-sized AOCs filled with oxygen or PFC gases and coated with biomimetic and oxygen-permeable lipids. As the lipid shell is ultra-thin and can be easily deformed or destructed when subjected to ultrasound irradiation, OMBs are frequently used in ultrasound-mediated oxygen therapy. The oxygen capacity is then directly proportional to the sizes of the OMBs; it usually varies from 5 to 500 m to according to the application and geometry of the targeted area [Citation127]. Commonly, OMBs must be stabilized using highly concentrated surfactants, making them prone to coalescence and Oswald ripening [Citation128]. Although OMBs can provide a high amount of oxygen in a short time by in vivo intravenous injection, the retention time of OMBs is generally very low (in minutes) relative to those of other micro-sized AOCs [Citation129]. Thus, for a more sustainable oxygen supply, the lipid shells of OMBs must be strengthened to achieve a higher circulation resistance. For instance, Reusser et al. [Citation130] utilized longer acyl-chain phospholipids as shells to increase the circulation time of the microbubbles to approximately 2 hours. Alternatively, the short circulation life of OMBs can be resolved by continuously supplying microbubbles through the vascular system to treat hypoxia. Very recently, Vutha et al. [Citation88] developed an OMB delivery system based on a microfluidic device to realize real-time, on-demand, and long-term intravenous oxygen delivery. They created a flow-focusing microfluidic device for producing lipidic OMBs with controlled particle sizes, tunable gas fractions, and volumetric flow rates. In their device, the initial microbubbles were broken up into microbubbles owing to a velocity-induced shear controlled according to customized nozzles and step changes. Thus, resultant OMB size could be changed in real-time by simply adjusting the liquid and gas input flow rates. These types of systems have great potential for automatically delivering oxygen on-demand, as they can be incorporated into oxygen-monitoring systems to providing on-time regulation of the production rates of the OMBs.
4.4 Comparison of current micro-sized AOCs to human RBCs (hRbcs)
Following years of development, many developers of current micro-sized AOCs are trying to mimic hRBCs to match their better performance in circulation. Some AOCs have achieved certain characteristics of hRBCs, such as their biconcave morphology, high elasticity, or high Hb loading by using alternative biomaterials, whereas others derived from RBC components (such as cell membranes) have generally become closer to original hRBCs.
In , we summarize examples of micro-sized AOCs either inspired by hRBCs or intended to mimic their properties and functionalities.
Table 1. Micro-sized AOCs which were inspired by hRBC.
As mentioned above, the impressive deformability of RBCs enables them to fold themselves as they pass through a vascular capillary with an inner diameter smaller than their size. Mimicking such deformability is crucial and especially meaningful for the in vivo application of micro-sized AOCs, as the oxygen carriers must be circulated or perfused in many cases in tissue engineering. Soft microparticles mimicking the morphology of healthy RBCs with advanced biomaterials have already been manufactured in several studies on HBOCs and PFOCs. Compared with the measured Young’s modulus of healthy RBCs of 4.4 ± 0.6 kPa [Citation133], the elastic moduli of the micro-sized AOCs made of, for example, a TEGA hydrogel could reach similarly low values, such as 6.5 kPa [Citation102]. Moreover, micro-sized oxygen carriers are often tested and have passed through silicon microchannels and recovered their shapes like RBCs. Therefore, it is believable that new materials softer than RBC membranes for AOC shells will continue to emerge with the advances in polymer science.
The oxygen capacity of an RBC is decent owing to its 33% Hb content. HBOCs or PFOCs have an ability to approach or even transcend the oxygen capacity of hRBCs, as they can also reversibly hold oxygen molecules. For micro-sized HBOCs, the highest Hb loading rate achieved so far is 70% [Citation102]. Regarding the micro-sized PFOCs, the oxygen-carrying capacity is also linearly dependent on the amount of PFCs incorporated in the microcarrier. However, to carry the same amount of oxygen to their maximum capacity, the micro-sized PFOCs are expected to need to be oxygenated under pure oxygen owing to the oxygen partial pressure according to Henry’s law.
A few research studies have adopted different methods to produce RBC replicas as templates with recombined RBC membranes for loading Hb or PFCs as oxygen carriers [Citation96,Citation97,Citation131]. As those particles are derived from components in natural RBCs, they can preserve the properties of RBCs to a great degree. For instance, Liu et al. [Citation131] recently developed aggressive man-made RBCs (AmmRBCs), i.e. spindle-shaped pseudo-RBCs with a transverse length of 0.5 μm and longitudinal length of 2 μm. In spite of the smaller volume of their particles relative to that of hRBCs, the membrane of the oxygen carrier was recombined with the hRBCs’ membrane, making them biocompatible and suitable for encapsulating an Hb-polydopamine (PDA) complex. As the PDA was an antioxidant for scavenging H2O2, the AmmRBCs shared a similarity with natural RBCs, as both contained natural antioxidative enzymes. However, the rebuilt RBCs as oxygen carriers still have limitations such as limited sources for the materials derived from donated blood, a complicated fabrication method, and relatively low yields.
In contrast to the complex bioenvironment and fragile nature of hRBCs, micro-sized AOCs based on polymeric platforms generally comprise much more simplified components with controlled resistance to metabolic transformation under severe conditions such as high temperatures and pH changes. Accordingly, they can be sterilized by means of filtration, pasteurization, and chemical cleaning, eliminating the sources of several diseases such as AIDS and hepatitis [Citation134]. In addition, the longer storage life tremendously reduces the potential cost of using AOCs as a blood substitute. To this end, it is expected that a new generation of micro-sized AOCs based on either Hb or PFCs possessing all of the advantages of hRBCs will be proposed in the near future.
5. Potential applications of micro-sized AOCs
AOCs have proven useful as blood alternatives, but have also demonstrated significant impacts on other biomedical fields. Nano-sized AOCs have been widely studied for various applications. However, the applied research on micro-sized AOCs was not as energetic as that on nano-sized AOCs until recently. Based on the previous applied research on nano-sized AOCs, the main fields where recent RBC-inspired micro-sized AOCs have achieved success are discussed below.
5.1 Tissue engineering via perfusion cultivation system using AOCs and channeled scaffold
Providing an oxygen supply is critically important to regenerating three-dimensional tissues in vitro. Like natural animal tissues which obtain sufficient oxygen from their capillary vessel networks and blood containing RBCs, scaffolds with channels and AOCs are necessary for tissue engineering. One model simulation predicted that a perfusion of PFC-based AOCs such as Oxygent® would be useful for the cultivation of cardiac tissue constructs [Citation135].
Supplying oxygen using AOCs requires a perfusion system for the cell culture media containing the AOCs. The cell culture media containing the AOCs work like the blood in our body. Gas exchangers and pumps work like the lungs and heart in our body, respectively [Citation136–138]. The perfusion system shown in simulates a circulation system similar to that in the body. The media containing PFC nano-sized micelles flow through the channels of scaffold in one direction. The perfusion system shown in utilizes an alternative infusion and withdrawal flow, enabling the system to be compact and easy to operate. Even the static culture shown in demonstrates the usefulness of AOCs for maintaining ex vivo cultures of human pancreatic slices. The corresponding researchers concluded that their technology would be useful for real-time regeneration/developmental studies in the human pancreas.
Figure 7. Culture systems using PFC-based AOCs. (a) Diagram of perfusion loop of oxygen supply with AOCs for culturing cardiac fibroblasts, adapted with permission from [Citation136]. Copyright 2006 UPV/EHU Press (b) Diagram of PerfusionPal system operation in which dense blood substitute acts as a piston to drive the flow of medium up and down, reprinted with permission from [Citation135]. Copyright 2020 Shoemaker et al. (c) Diagram outlining the perfluorocarbon (PFC) membrane AirHive cell culture dish compared to a transwell dish, reprinted with permission from [Citation134]. Copyright 2020 Mirza Muhammad Fahd Qadir et al.
![Figure 7. Culture systems using PFC-based AOCs. (a) Diagram of perfusion loop of oxygen supply with AOCs for culturing cardiac fibroblasts, adapted with permission from [Citation136]. Copyright 2006 UPV/EHU Press (b) Diagram of PerfusionPal system operation in which dense blood substitute acts as a piston to drive the flow of medium up and down, reprinted with permission from [Citation135]. Copyright 2020 Shoemaker et al. (c) Diagram outlining the perfluorocarbon (PFC) membrane AirHive cell culture dish compared to a transwell dish, reprinted with permission from [Citation134]. Copyright 2020 Mirza Muhammad Fahd Qadir et al.](/cms/asset/4ff40f80-668d-46a9-97af-6d87be906802/tsta_a_2223050_f0007_oc.jpg)
To the best of our knowledge, the AOCs applied in tissue engineering have mainly been nano-sized AOCs so far. In mechanobiology and physiology, the dynamic interactions between RBCs and endothelial cells comprise a popular topic that has not yet been sufficiently clarified [Citation139]. Hence, we expect that the micro-sized AOCs inspired by RBCs will be applied in perfusion cultivation and contribute to regenerating more functional tissues by mimicking the interactions between RBCs and endothelial cells in tissue engineering and regenerative medicine.
5.2 Local oxygen therapies using AOCs and regenerative medicine
Hyperbaric oxygen therapy (HBOT) is the intermittent inhalation of 100% oxygen at a pressure greater than 1 atmosphere. This therapy increases the amount of oxygen dissolved in the blood by 10–20 times compared to the amount of oxygen obtained by breathing under a normal atmospheric pressure. This dissolved oxygen amount is slightly more than sufficient to meet the resting cellular requirements [Citation140,Citation141]. Furthermore, the dissolved oxygen does not transport itself via binding to Hb, so the HBOT can carry oxygen to the body independent of the amount of Hb or its ability to bind. Since Behnke and Shaw succeeded in the treatment of decompression sickness using hyperbaric oxygen in 1937 [Citation142], HBOT has become a useful therapy for treating several diseases such as chronic refractory osteomyelitis, delayed radiation-induced injuries with bone necrosis, cardiac ischemia, air or gas arterial or venous emboli, and severe or symptomatic carbon monoxide poisoning [Citation140,Citation143–147]. However, HBOT typically requires hyperbaric chambers including either mono-place chambers for accommodating a single patient at one time or multi-place chambers for accommodating multiple patients at the same pressure [Citation145]. There is a limitation to the number of hospitals able to provide hyperbaric treatment because of the space required to set up such hyperbaric chambers. In addition, several complications from HBOTs have been described with varying degrees of seriousness [Citation140,Citation141]. The most frequent side effect is hyperbaric-associated middle ear barotrauma [Citation148,Citation149]. Recently, the possibility of using AOCs as an alternative to HBOT was investigated with various animal models. Below, we introduce recent studies using PFOCs.
Myocardial infarction is caused by the occlusion of a coronary artery, leading to the depletion of oxygen from cardiac tissue. Thus, myocardial cells in the infarcted areas under hypoxia lose their functions and undergo cell death. A sufficient and continuous oxygen supply is necessary to overcome the hypoxic conditions of damaged cardiac tissues. In 2005, Radisic et al. reported that PFC emulsions were effective in myocardial protection therapy, based on a mathematical model of oxygen distribution from the PFC and experiments on rat neonatal cardiac tissues cultured in a medium supplemented with PFC emulsions [Citation135]. Liu et al. showed that a single dose of a dodecafluoropentane -based perfluorocarbon emulsion could significantly reduce myocardial injury in a rat myocardial ischemia model using 99mTc-duramycin single-photon emission computed tomography imaging [Citation150]. Furthermore, Qin et al. showed that pigment epithelium-derived factor-loaded PFC nanoemulsions reduced ischemic myocardial injuries in a rat myocardial ischemia model [Citation151].
Peripheral nerve injury is another indication for HOBT because oxygen promotes nerve regeneration and reduces inflammatory cells. For instance, Schwann cells are involved in peripheral nerve regeneration, including myelin formation, the secretion of neurotrophins, and the production of extracellular matrix molecules. In 2013, Ma et al. revealed that perfluorotributylamine (PFTBA) with a thrombin-enriched fibrin hydrogel increased the viability of Schwann cells and the expression of regeneration-related genes under hypoxia in both 2D and 3D cultures [Citation152]. In rats with 12-mm-sciatic nerve defects, transplantation of Schwann cells with the PFTBA hydrogel showed better sciatic nerve regeneration in comparison to a control without the hydrogel [Citation153]. However, the time for releasing oxygen from the PFTBA hydrogel was limited to 48 hours. Later, Teng et al. modified the PFTBA hydrogel using a coaxial electrospinning technique to prolong the oxygen supply [Citation69]. PFTBA core-shell fibers containing PFTBA in the core and PCL-chitosan in the shell were able to extend the oxygen supply to up to 144 hours. The Schwann cells in the PFTBA core-shell fibers increased the survival and expression of regeneration-related genes under hypoxic conditions in vitro. Furthermore, improved axonal regeneration and remyelination by the PFTBA core-shell fibers were observed in a 17-mm rat sciatic nerve defect model.
Chronic refractory osteomyelitis and delayed radiation-induced injuries with bone necrosis are also indications for HOBT, because hypoxia inhibits bone growth and recovery. Furthermore, in the case of osteomyelitis caused by successive invasions of microorganisms, the oxygen supply has a direct antibacterial effect and enhances the antimicrobial activity of leukocytes. Recently, it was reported that human periosteum-derived osteoblasts in PFO emulsion-loaded hollow microparticles containing PFO in the core and PCL in the shell increased cell viability and maintained the ability of osteogenic differentiation [Citation154]. In a miniature pig staphylococcus aureus mandibular osteomyelitis model, implanted human periosteum-derived osteoblasts in PFO emulsion-loaded hollow microparticles promoted new bone formation [Citation154,Citation155].
Other animal studies have shown that AOCs improve cell viability and functionality in several tissues, including liver and pancreas tissues. It is expected that micro-sized AOCs will be further investigated for clinical applications for HOBT-approved diseases.
5.3 AOCs and AOCs-encapsulated scaffolds for wound dressing
Local oxygenation therapy is as effective for skin wound healing as it is for myocardial infarctions and peripheral nerve injuries. The topical Hb spray Granulox® was approved in Europe in 2012. It was reported that Granulox® improved the wound closure, wound size, pain, sloughing, and exudate levels of patients with chronic diabetic foot ulcers [Citation156].
Wound dressings containing AOCs for excellent wound healing have been studied in previous research [Citation111,Citation123,Citation157–159]. shows an example of oxygen-releasing hydrogel sponge, i.e. an alginate-based hydrogel embedded with H2O2-PLGA microspheres [Citation123]. The sizes of the AOCs containing H2O2 were controlled by sieving to between 50–250 µm. Perfluorodecalin-encapsulated albumin nanoparticles were embedded in the self-healing hydrogel [Citation159]. In another study, injectable hydrogels and microcarriers [Citation111] were tested to be as suitable as sheet hydrogels for wound dressings [Citation157]. Recently, molybdenum disulfide quantum dots or microalgae [Citation158] were used as AOCs for wound dressing. In this field, AOCs with sizes larger than the sizes of RBCs can be applied, as they do not circulate or make direct contact with cells.
Figure 8. Oxygen releasing scaffold using micro-sized AOCs for new wound dressing, adapted with permission from [Citation122]. Copyright 2018 Jeongyeon Choi et al.
![Figure 8. Oxygen releasing scaffold using micro-sized AOCs for new wound dressing, adapted with permission from [Citation122]. Copyright 2018 Jeongyeon Choi et al.](/cms/asset/b63ab7dd-900c-41ea-b296-b0d659f6089f/tsta_a_2223050_f0008_oc.jpg)
5.4 Organ transplantation
Conventional cold storage cannot be used for many potential donor organs. For example, in general only 63% of UK deceased donor livers are transplanted. The perfusion of oxygenated blood with medications and nutrients at body temperature, called normothermic machine perfusion (NMP), is expected to solve this problem. Randomized control trials of NMP in liver transplantation have indicated its usefulness [Citation160]. In lung transplantation, ex vivo normothermic perfusion (EVNP) before transplantation has been clinically studied to reassess donor lung functions [Citation161]. HBOCs were found to show potential in several reported preclinical and clinical research studies; illustrations of certain HBOC applications are shown in [Citation14]. Albumin-derived perfluorodecalin-filled nanocapsules have also applied for the EVNP of kidneys in rats, suggesting the potential of using PFOCs [Citation162]. The functions of micro-sized AOCs for such applications have not yet been explored.
Figure 9. Application of HBOC in solid organ preservation. (a) Pre-oxygenation process of HBOC-added preservation solution. (b) Static cold preservation of isolated liver after pre-oxygenation with the HBOC-added preservation solution. (c) Mechanical perfusion of the isolated liver after pre-oxygenation. Adapted with permission from [Citation14]. Copyright 2021 Cao et al.
![Figure 9. Application of HBOC in solid organ preservation. (a) Pre-oxygenation process of HBOC-added preservation solution. (b) Static cold preservation of isolated liver after pre-oxygenation with the HBOC-added preservation solution. (c) Mechanical perfusion of the isolated liver after pre-oxygenation. Adapted with permission from [Citation14]. Copyright 2021 Cao et al.](/cms/asset/7a09bf35-8e1b-4e79-92ad-bbea2128add3/tsta_a_2223050_f0009_oc.jpg)
Figure 10. Functionalization process and confocal microscopic images of Ru(ddp)-loaded FDC emulsions stabilized with 2% F127 under hypoxic condition (a,b) and normoxic condition (c,d). Scale bars: 20 μm, reprinted with permission from [Citation73]. Copyright 2019, American Chemical Society.
![Figure 10. Functionalization process and confocal microscopic images of Ru(ddp)-loaded FDC emulsions stabilized with 2% F127 under hypoxic condition (a,b) and normoxic condition (c,d). Scale bars: 20 μm, reprinted with permission from [Citation73]. Copyright 2019, American Chemical Society.](/cms/asset/0f3aedcc-874d-4dea-b435-6d924843c380/tsta_a_2223050_f0010_oc.jpg)
5.5 Biosensors
It is always meaningful to monitor the oxygen tension as it is closely related to fates of cells, such as their growth, differentiation, and death, tissue damage and inflammation, and cancer development [Citation163]. RBCs are natural oxygen sensors, as oxy-hemoglobin and deoxy-hemoglobin have different visible light and infrared red-light absorption. Pulse oximeters utilize this phenomenon. The degree of RBC hemoglobin desaturation is strongly correlated to the oxygen demands of tissues. In addition, RBCs release adenosine triphosphate in response to the low local oxygen tension as they circulate in the blood vessels. Therefore, in reality, RBCs perform as both oxygen sensors and oxygen carriers, enabling them to deliver oxygen on-demand to tissues through the complex vasculature of the body [Citation164]. For the same reason, AOCs also have the potential to be modified to monitor oxygen levels while simultaneously carrying oxygen, thereby achieving automatic site-sensing oxygen transfer. Liu et al. [Citation165] recently developed chitosan/Hb microcapsules as micro-sized oxygen biosensors by immobilizing them on the surface of an Au nanoparticle-modified-glass carbon electrode. Owing to the protection of the chitosan shell, the problem of electrode fouling by direct adsorption of the Hb onto the electrode surface was evaded; moreover, the sensitivity of the oxygen biosensor was higher than when depositing Hb without a shell [Citation166]. Previously, they prepared chitosan/Hb nanocomposites on the surface for a same oxygen-sensing purpose [Citation167]. However, despite those nanoparticles or microparticles having functionalities for both oxygen sensing and oxygen carrying, they could not work as both simultaneously, as those particles were immobilized on the surface of the probe for enhancing the oxygen diffusion. Fu et al. [Citation74] recently loaded Ru(dpp) nanoparticles uniformly on the surface of micro-sized perfluorodecalin emulsions as an oxygen-sensitive probe. The author showed that the micro-sized emulsions could reflect the oxygen level in the surroundings during oxygen supply to the cells in vitro, as shown in the confocal microscopic images in . Theranostics is another key technology in medicine. As we described, RBCs naturally have theranostic natures for delivering and sensing oxygen. AOCs inspired by RBCs could therefore have both oxygen delivery and sensing functions in the future.
5.6 Future challenges in applying micro-sized AOCs
Despite the promising oxygen capacity of PFCs and Hb, oxygen with a high oxygen partial pressure must still be replenished during circulation for PFOCs and HBOCs to maintain their maximum capacities. For the other micro-sized AOC types, increased efforts need to be spent on increasing the oxygen capacity or mitigating the initial oxygen burst to prolong the duration of oxygen release [Citation168]. As most micro-sized AOCs are constructed with a core-shell structure, the properties of the shell materials are important to the behaviors of the core-shell particles during perfusion and circulation. The surface properties of micro-sized AOC particles may require modifications (such as antithrombotic coatings) for clinical use in the future. Deformability is another crucial property for micro-sized AOCs to circulate through micro-vessels; it is affected not only by the material rigidity, but also the shell thicknesses and shapes of the micro-sized AOCs. For instance, Fu et al. [Citation81] showed that concave PFOB/PLC microparticles could pass through a silicon channel, whereas spherical PFOB/PLC microparticles with the same composition and size could not. As the practical use of micro-sized AOCs also demands large quantities of the particles, another technical challenge concerns scaling up the current techniques in the laboratory to an industrial level. Future novel biomaterials and advanced fabrication techniques are expected to address these challenges.
6. Conclusion
In recent years, HBOCs, PFCs, POs, and OMBs have been the most representative types of AOCs manufactured in microparticles or microcapsules. Despite their shared similar sizes and structural features (such as core-shell structures), different types of AOCs had distinguishing behaviors for oxygen generation or release, and correspondingly were designed to work under different circumstances. Additional types of micro-sized HBOCs and PFOCs have recently emerged with the intention to mimic the morphology and physical properties of RBCs, which in turn would potentially provide them with favorable rheological properties. Thus, hRBCs and micro-sized AOCs were discussed in this review. The comparison between AOCs, RBCs of various species, and hRBCs provides insights for the design of novel AOCs in the future. In summary, the functionality of many current micro-sized AOCs is being improved and will continue to be improved to meet the needs of tissue engineering as biocompatible and high-performance oxygen carriers. In addition, scaled-up strategies and cost evaluations are required for the large-scale production of AOCs. It is expected that in the future micro-sized AOCs will be employed in a variety of practical uses (as are their nano-sized counterparts) in tissue engineering, blood transfusion alternatives, local oxygenation therapies, organ preservation, and so on.
Disclosure statement
No potential conflict of interest was reported by the author(s).
Additional information
Funding
Notes on contributors
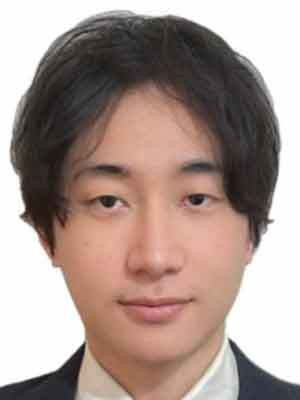
Qiming Zhang
Qiming Zhang received his B.S of chemical engineering from the University of California, Santa Barbara (2018) and his M.Eng of chemical and biomolecular engineering from Johns Hopkins University (2020). He is now pursuing his Ph.D degree in the University of Tokyo, chemical system engineering. He worked as a Ph.D candidate in Prof. Ito Taichi’s lab in the University of Tokyo, focusing on biomaterials and tissue engineering.
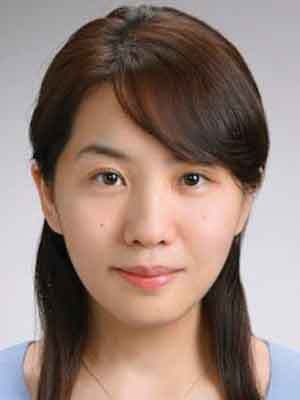
Natsuko F. Inagaki
Natsuko F Inagaki received her Ph.D. of Medicine (2011) from the University of Tokyo. She worked as a postdoctoral fellow at Institute of Molecular and Cellular Biosciences, the University of Tokyo and National Center for Global Health and Medicine. Now she is an assistant professor of Center for Disease Biology and Integrative Medicine, the University of Tokyo.
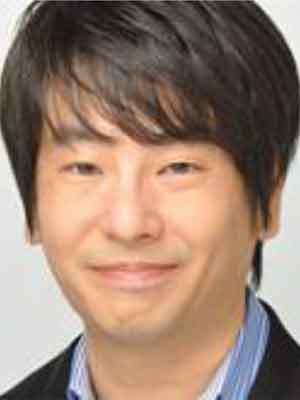
Taichi Ito
Taichi Ito received his B. Engineering (1996) and Ph.D. (2004) from the University of Tokyo. He worked as a postdoctoral fellow at department of chemical engineering, Massachusetts Institute of Technology. He became an assistant professor of Chemical Resources Laboratory, Tokyo Institute of Technology. Currently he is a professor of Center for Disease Biology and Integrative Medicine, Department of Chemical System Engineering, Department of Bioengineering, the University of Tokyo.
References
- Trayhurn P. Oxygen—A critical, but overlooked nutrient. Front Nutr. 2019;6:10. doi: 10.3389/fnut.2019.00010
- Sarker M, Chen XB, Schreyer DJ. Experimental approaches to vascularisation within tissue engineering constructs. J Biomater Sci Polym Ed. 2015;26(12):683–25. doi: 10.1080/09205063.2015.1059018
- Vaupel P, Mayer A. Hypoxia in cancer: significance and impact on clinical outcome. Cancer Metast Rev. 2007;26(2):225–239. doi: 10.1007/s10555-007-9055-1
- Bäumler H. Künstliche Sauerstofftransporter können mehr als Sauerstoff liefern. Transfusionsmedizin. 2020;10(4):199–207. doi: 10.1055/a-1119-1796
- Goodnough LT, Bodner MS, Martin JW. Blood transfusion and blood conservation: cost and utilization issues. Am J Med Qual. 1994;9(4):172–183. doi: 10.1177/0885713X9400900408
- Gulliksson H, van der Meer PF. Storage of whole blood overnight in different blood bags preceding preparation of blood components: in vitro effects on red blood cells. Blood Transfus. 2009;7:210–215. doi: 10.2450/2009.0074-08
- Kim HW, Greenburg AG. Artificial oxygen carriers as red blood cell substitutes: a selected review and current status. Artif Organs. 2004;28(9):813–828. doi: 10.1111/j.1525-1594.2004.07345.x
- Khan F, Singh K, Friedman MT. Artificial blood: the history and current perspectives of blood substitutes. Discoveries. 2020;8:e104. doi: 10.15190/d.2020.1
- Amberson WR, Jennings JJ, Rhode CM. Clinical experience with hemoglobin-saline solutions. J Appl Physiol. 1949;1(7):469–489. doi: 10.1152/jappl.1949.1.7.469
- Sen Gupta A. Hemoglobin-based oxygen carriers: current state-of-the-art and novel molecules. Shock. 2019;52(1S):70–83. Lippincott Williams and Wilkins. doi: 10.1097/SHK.0000000000001009
- Sen Gupta A. Bio‐inspired nanomedicine strategies for artificial blood components. WIREs Nanomed Nanobiotechnol. 2017;9:10.1002. doi: 10.1002/wnan.1464
- Gould A. Clinical development of human polymerized hemoglobin as a blood substitute. World J Surg. 1996;20:1200–1207. doi: 10.1007/s002689900183
- Mohanto N, Park Y-J, Jee J-P. Current perspectives of artificial oxygen carriers as red blood cell substitutes: a review of old to cutting-edge technologies using in vitro and in vivo assessments. J Pharm Investig. 2022;53:153–190. doi: 10.1007/s40005-022-00590-y
- Cao M, Wang G, He H, et al. Hemoglobin-based oxygen carriers: potential applications in solid organ preservation. Front Pharmacol. 2021;12:760215. doi: 10.3389/fphar.2021.760215
- Kure T, Sakai H. Preparation of artificial red blood cells (hemoglobin vesicles) using the rotation–revolution mixer for high encapsulation efficiency. ACS Biomater Sci Eng. 2021;7(6):2835–2844. doi: 10.1021/acsbiomaterials.1c00424
- Tomita D, Kimura T, Hosaka H, et al. Covalent core–shell architecture of hemoglobin and human serum albumin as an artificial O 2 carrier. Biomacromolecules. 2013;14(6):1816–1825. doi: 10.1021/bm400204y
- Clark LC, Gollan F. Survival of mammals breathing organic liquids equilibrated with oxygen at atmospheric pressure. Science. 1966;152:1755–1756. doi: 10.1126/science.152.3730.1755
- Riess JG. Understanding the fundamentals of perfluorocarbons and perfluorocarbon emulsions relevant to In Vivo oxygen delivery. Artif Cells Blood Substitutes Biotechnol. 2005;33(1):47–63. doi: 10.1081/BIO-200046659
- Jahr JS, Guinn NR, Lowery DR, et al. Blood substitutes and oxygen therapeutics: a review. Anesth Analg. 2021;132(1):119–129. doi: 10.1213/ANE.0000000000003957
- Yu M, Dai M, Liu Q, et al. Oxygen carriers and cancer chemo- and radiotherapy sensitization: bench to bedside and back. Cancer Treat Rev. 2007;33(8):757–761. doi: 10.1016/j.ctrv.2007.08.002
- Vorob’ev SI. First- and second-generation perfluorocarbon emulsions. Pharm Chem J. 2009;43(4):209–218. doi: 10.1007/s11094-009-0268-1
- Goorha Y, Deb P, Chatterjee T, et al. Artificial blood. Med J Armed Forces India. 2003;59(1):45–50. doi: 10.1016/S0377-1237(03)80107-7
- Jaegers J, Haferkamp S, Arnolds O, et al. Deciphering the emulsification process to create an albumin-perfluorocarbon-(o/w) nanoemulsion with high shelf life and bioresistivity. Langmuir. 2022;38(34):10351–10361. doi: 10.1021/acs.langmuir.1c03388
- Okamoto W, Hasegawa M, Usui T, et al. Hemoglobin–albumin clusters as an artificial O 2 carrier: physicochemical properties and resuscitation from hemorrhagic shock in rats. J Biomed Mater Res B Appl Biomater. 2022;110(8):1827–1838. doi: 10.1002/jbm.b.35040
- Azuma H, Amano T, Kamiyama N, et al. First-in-human phase 1 trial of hemoglobin vesicles as artificial red blood cells developed for use as a transfusion alternative. Blood Adv. 2022;6:5711–5715. doi: 10.1182/bloodadvances.2022007977
- L’Heureux N, Dusserre N, Marini A, et al. Technology Insight: the evolution of tissue-engineered vascular grafts—from research to clinical practice. Nat Clin Pract Cardiovasc Med. 2007;4(7):389–395. doi: 10.1038/ncpcardio0930
- Bah MG, Bilal HM, Wang J. Fabrication and application of complex microcapsules: a review. Soft Matter Royal Society Chem. 2020;16:570–590. doi: 10.1039/C9SM01634A
- Luo T, Wang Z, He J, et al. Ultrasound-mediated destruction of oxygen and paclitaxel loaded dual-targeting microbubbles for intraperitoneal treatment of ovarian cancer xenografts. Cancer Lett. 2017;391:1–11. doi: 10.1016/j.canlet.2016.12.032
- Shiga T, Maeda N, Kon K. Erythrocyte rheology. Crit Rev Oncol Hematol. 1990;10(1):9–48. doi: 10.1016/1040-8428(90)90020-S
- Baskurt OK, Meiselman HJ. Hemodynamic effects of red blood cell aggregation. Indian J Exp Biol. 2007;45:25–31.
- Ford J. Red blood cell morphology. Int J Lab Hematol. 2013;35(3):351–357. doi: 10.1111/ijlh.12082
- Diez-Silva M, Dao M, Han J, et al. Shape and biomechanical characteristics of human red blood cells in health and disease. MRS Bull. 2010;35(5):382–388. doi: 10.1557/mrs2010.571
- Marchi G, Bozzini C, Bertolone L, et al. Red blood cell morphologic abnormalities in patients hospitalized for COVID-19. Front Physiol. 2022;13:932013. doi: 10.3389/fphys.2022.932013
- Gijsen FJH, van de Vosse FN, Janssen JD. The influence of the non-Newtonian properties of blood on the flow in large arteries: steady flow in a carotid bifurcation model. J Biomech. 1999;32(6):601–608. doi: 10.1016/S0021-9290(99)00015-9
- Kim Y, Kim K, Park Y. Measurement techniques for red blood cell deformability: recent advances. Blood Cell Overview Stud Hematol. 2012;10:167–194. InTech.
- Nicolaides AN, Horbourne T, Bowers R, et al. Blood viscosity, red-cell flexibility, hæmatocrit, and plasma-fibrinogen in patients with angina. Lancet. 1977;310(8045):943–945. doi: 10.1016/S0140-6736(77)90886-8
- Noguchi H, Gompper G. Shape transitions of fluid vesicles and red blood cells in capillary flows. Proc Natl Acad Sci, USA. 2005;102:14159–14164. doi: 10.1073/pnas.0504243102
- Nader E, Skinner S, Romana M, et al. Blood rheology: key parameters, impact on blood flow, role in sickle cell disease and effects of exercise. Front Physiol. 2019;10:1329. doi: 10.3389/fphys.2019.01329
- Baskurt OK, Yalcin O, Meiselman HJ. Hemorheology and vascular control mechanisms. Clin Hemorheol Microcirc. 2004;30:169–178.
- Raj J, Kaapa P, Hillyard R, et al. Pulmonary vascular pressure profile in adult ferrets: measurements in vivo and in isolated lungs. Acta Physiol Scand. 1991;142(1):41–48. doi: 10.1111/j.1748-1716.1991.tb09126.x
- Parthasarathi K, Lipowsky HH. Capillary recruitment in response to tissue hypoxia and its dependence on red blood cell deformability. Am J Physiol Heart Circ Physiol. 1999;277(6):H2145–H2157. doi: 10.1152/ajpheart.1999.277.6.H2145
- Vadapalli A, Goldman D, Popel AS. Calculations of oxygen transport by red blood cells and hemoglobin solutions in capillaries. Artif Cells Blood Substitutes Biotechnol. 2002;30(3):157–188. doi: 10.1081/BIO-120004338
- Burger P, Korsten H, Verhoeven AJ, et al. Collection and storage of red blood cells with anticoagulant and additive solution with a physiologic pH. Transfusion (Paris). 2012;52(6):1245–1252. doi: 10.1111/j.1537-2995.2011.03472.x
- Shinar E, Prober G, Yahalom V, et al. WBC filtration of whole blood after prolonged storage at ambient temperature by use of an in-line filter collection system. Transfusion (Paris). 2002;42(6):734–737. doi: 10.1046/j.1537-2995.2002.00118.x
- Marschner S, Dimberg LY. Pathogen reduction technologies. In: Beth H, Shaz BH, Hillyer CD, Gil MR, editors. Transfusion medicine and hemostasis. Elsevier; 2019. p. 289–293.
- Hess JR. An update on solutions for red cell storage. Vox Sang. 2006;91(1):13–19. doi: 10.1111/j.1423-0410.2006.00778.x
- Pulliam KE, Joseph B, Morris MC, et al. Innate coagulability changes with age in stored packed red blood cells. Thromb Res. 2020;195:35–42. doi: 10.1016/j.thromres.2020.06.047
- Kohli N, Bhaumik S, Jagadesh S, et al. Packed red cells versus whole blood transfusion for severe paediatric anaemia, pregnancy-related anaemia and obstetric bleeding: an analysis of clinical practice guidelines from sub-Saharan Africa and evidence underpinning recommendations. Trop Med Int Health. 2019;24(1):11–22. doi: 10.1111/tmi.13173
- Kumar H, Gupta P, Mishra D, et al. Leucodepletion and blood products. Med J Armed Forces India. 2006;62(2):174–177. doi: 10.1016/S0377-1237(06)80064-X
- Gillio-Meina C, Cepinskas G, Cecchini EL, et al. Translational research in pediatrics ii: blood collection, processing, shipping, and storage. Pediatrics. 2013;131(4):754–766. doi: 10.1542/peds.2012-1181
- Gkoumassi E, Dijkstra-Tiekstra MJ, Hoentjen D, et al. Hemolysis of red blood cells during processing and storage. Transfusion (Paris). 2012;52(3):489–492. doi: 10.1111/j.1537-2995.2011.03298.x
- Said AS, Rogers SC, Doctor A. Physiologic impact of circulating RBC microparticles upon blood-vascular interactions. Front Physiol. 2018;8:1120. doi: 10.3389/fphys.2017.01120
- Kriebardis AG, Antonelou MH, Stamoulis KE, et al. Membrane protein carbonylation in non-leukodepleted CPDA-preserved red blood cells. Blood Cells Mol Dis. 2006;36(2):279–282. doi: 10.1016/j.bcmd.2006.01.003
- Kriebardis AG, Antonelou MH, Stamoulis KE, et al. Progressive oxidation of cytoskeletal proteins and accumulation of denatured hemoglobin in stored red cells. J Cell Mol Med. 2007;11(1):148–155. doi: 10.1111/j.1582-4934.2007.00008.x
- Yoshida T, Prudent M, D’alessandro A. Red blood cell storage lesion: causes and potential clinical consequences. Blood Transfus. 2019;17:27–52. doi: 10.2450/2019.0217-18
- Sparrow RL. Red blood cell components: time to revisit the sources of variability. Blood Transfus. 2017;15(2):116–125. doi: 10.2450/2017.0326-16
- Forbes J, Anderson M, Anderson G, et al. Blood transfusion costs: a multicenter study. Transfusion (Paris). 1991;31(4):318–323. doi: 10.1046/j.1537-2995.1991.31491213295.x
- Modery-Pawlowski CL, Tian LL, Pan V, et al. Synthetic approaches to RBC mimicry and oxygen carrier systems. Biomacromolecules. 2013;14(4):939–948. doi: 10.1021/bm400074t
- Xiong Y, Steffen A, Andreas K, et al. Hemoglobin-based oxygen carrier microparticles: synthesis, properties, and in vitro and in vivo investigations. Biomacromolecules. 2012;13(10):3292–3300. doi: 10.1021/bm301085x
- Butler MF, Ng Y-F, Pudney PDA. Mechanism and kinetics of the crosslinking reaction between biopolymers containing primary amine groups and genipin. J Polym Sci A Polym Chem. 2003;41(24):3941–3953. doi: 10.1002/pola.10960
- Xiong Y, Liu ZZ, Georgieva R, et al. Nonvasoconstrictive hemoglobin particles as oxygen carriers. ACS Nano. 2013;7(9):7454–7461. doi: 10.1021/nn402073n
- Schakowski KM, Linders J, Ferenz KB, et al. Synthesis and characterisation of aqueous haemoglobin-based microcapsules coated by genipin-cross-linked albumin. J Microencapsul. 2020;37(3):193–204. doi: 10.1080/02652048.2020.1715498
- Duan L, Yan X, Wang A, et al. Highly loaded hemoglobin spheres as promising artificial oxygen carriers. ACS Nano. 2012;6(8):6897–6904. doi: 10.1021/nn301735u
- Anu Bhushani J, Anandharamakrishnan C. Electrospinning and electrospraying techniques: potential food based applications. Trends Food Sci Technol. 2014;38(1):21–33. doi: 10.1016/j.tifs.2014.03.004
- de Sousa Araújo E, Domingues Stocco T, Fernandes de Sousa G, et al. Oxygen-generating microparticles in chondrocytes-laden hydrogels by facile and versatile click chemistry strategy. Colloids Surf B Biointerfaces. 2021;205:111850. doi: 10.1016/j.colsurfb.2021.111850
- Khorshidi S, Karkhaneh A, Bonakdar S. Fabrication of amine-decorated nonspherical microparticles with calcium peroxide cargo for controlled release of oxygen. J Biomed Mater Res A. 2020;108(1):136–147. doi: 10.1002/jbm.a.36799
- Morais AIS, Wang X, Vieira EG, et al. Electrospraying oxygen-generating microparticles for tissue engineering applications. Int J Nanomedicine. 2020;15:1173–1186. doi: 10.2147/IJN.S237334
- Zhang M, Kiratiwongwan T, Shen W. Oxygen-releasing polycaprolactone/calcium peroxide composite microspheres. J Biomed Mater Res B Appl Biomater. 2020;108(3):1097–1106. doi: 10.1002/jbm.b.34461
- Ma T, Yang Y, Quan X, et al. Oxygen carrier in core-shell fibers synthesized by coaxial electrospinning enhances Schwann cell survival and nerve regeneration. Theranostics. 2020;10(20):8957–8973. doi: 10.7150/thno.45035
- Zhang L, Huang J, Si T, et al. Coaxial electrospray of microparticles and nanoparticles for biomedical applications. Expert Rev Med Devices. 2012;9(6):595–612. doi: 10.1586/erd.12.58
- Yoon J, Yang H-S, Lee B-S, et al. Recent progress in coaxial electrospinning: new parameters, various structures, and wide applications. Adv Mater. 2018;30(42):1704765. doi: 10.1002/adma.201704765
- Sawalha H, Schroën K, Boom R. Biodegradable polymeric microcapsules: preparation and properties. Chem Eng J. 2011;169(1–3):1–10. doi: 10.1016/j.cej.2011.02.078
- Sharipova A, Aidarova S, Mutaliyeva B, et al. The use of polymer and surfactants for the microencapsulation and emulsion stabilization. Colloids And Interfaces. 2017;1(1):3. doi: 10.3390/colloids1010003
- Fu X, Ohta S, Kamihira M, et al. Size-controlled preparation of microsized perfluorocarbon emulsions as oxygen carriers via the shirasu porous glass membrane emulsification technique. Langmuir. 2019;35(11):4094–4100. doi: 10.1021/acs.langmuir.9b00194
- Zarzar LD, Sresht V, Sletten EM, et al. Dynamically reconfigurable complex emulsions via tunable interfacial tensions. Nature. 2015;518(7540):520–524. doi: 10.1038/nature14168
- Nejati S, Karimi-Soflou R, Karkhaneh A. Influence of process parameters on the characteristics of oxygen-releasing poly (lactic acid) microparticles: a multioptimization strategy. Polym Adv Technol. 2021;32(2):829–841. doi: 10.1002/pat.5134
- Charcosset C, Limayem I, Fessi H. The membrane emulsification process—a review. J Chem Technol Biot. 2004;79(3):209–218. doi: 10.1002/jctb.969
- Chu L-Y, Xie R, Zhu J-H, et al. Study of SPG membrane emulsification processes for the preparation of monodisperse core–shell microcapsules. J Colloid Interface Sci. 2003;265(1):187–196. doi: 10.1016/S0021-9797(03)00350-3
- Lai YT, Ohta S, Akamatsu K, et al. Size-dependent interaction of cells and hemoglobin-albumin based oxygen carriers prepared using the SPG membrane emulsification technique. Biotechnol Prog. 2015;31:1676–1684. doi: 10.1002/btpr.2170
- Lai YT, Sato M, Ohta S, et al. Preparation of uniform-sized hemoglobin-albumin microspheres as oxygen carriers by Shirasu porous glass membrane emulsification technique. Colloids Surf B Biointerfaces. 2015;127:1–7. doi: 10.1016/j.colsurfb.2015.01.018
- Fu X, Ohta S, Kawakatsu T, et al. Bioinspired perfluorocarbon-based oxygen carriers with concave shape and deformable shell. Adv Mater Technol. 2022;7(3):2100573. doi: 10.1002/admt.202100573
- Ohta S, Hashimoto K, Fu X, et al. Development of human-derived hemoglobin–albumin microspheres as oxygen carriers using shirasu porous glass membrane emulsification. J Biosci Bioeng. 2018;126(4):533–539. doi: 10.1016/j.jbiosc.2018.04.017
- Bao D, Zhang H, Liu X, et al. Preparation of monodispersed polymer microspheres by SPG membrane emulsification‐solvent evaporation technology. J Dispers Sci Technol. 2007;28(3):485–490. doi: 10.1080/01932690601108052
- Nakashima T, Shimizu M, Kukizaki M. Particle control of emulsion by membrane emulsification and its applications. Adv Drug Deliv Rev. 2000;45(1):47–56. doi: 10.1016/S0169-409X(00)00099-5
- Duncanson WJ, Lin T, Abate AR, et al. Microfluidic synthesis of advanced microparticles for encapsulation and controlled release. Lab Chip. 2012;12(12):2135. doi: 10.1039/c2lc21164e
- Upadhyay A, Dalvi SV. Microbubble formulations: synthesis, stability, modeling and biomedical applications. Ultrasound Med Biol. 2019;45:301–343. doi: 10.1016/j.ultrasmedbio.2018.09.022
- Castro-Hernández E, van Hoeve W, Lohse D, et al. Microbubble generation in a co-flow device operated in a new regime. Lab Chip. 2011;11(12):2023. doi: 10.1039/c0lc00731e
- Vutha AK, Patenaude R, Cole A, et al. A microfluidic device for real-time on-demand intravenous oxygen delivery. Proc Natl Acad Sci. 2022;119:e2115276119. doi: 10.1073/pnas.2115276119
- Ma R, Wu Q, Si T, et al. Oxygen and indocyanine green loaded microparticles for dual-mode imaging and sonodynamic treatment of cancer cells. Ultrason Sonochem. 2017;39:197–207. doi: 10.1016/j.ultsonch.2017.03.019
- Xu J, Yuan S, Tian J, et al. Ultrasound mediated delivery of oxygen and LLL12 loaded stimuli responsive microdroplets for the treatment of hypoxic cancer cells. Sci Rep. 2017;7(1):44908. doi: 10.1038/srep44908
- Liu G, Wu Q, Dwivedi P, et al. Hemoglobin-laden microcapsules for simulating oxygen dynamics of biological tissue. ACS Biomater Sci Eng. 2018;4(9):3177–3184. doi: 10.1021/acsbiomaterials.8b00830
- Sadek SH, Rubio M, Lima R, et al. Blood particulate analogue fluids: a review. Materials. 2021;14:2451. doi: 10.3390/ma14092451
- de Koker S, Hoogenboom R, de Geest BG. Polymeric multilayer capsules for drug delivery. Chem Soc Rev. 2012;41(7):2867. doi: 10.1039/c2cs15296g
- Ariga K, Yamauchi Y, Rydzek G, et al. Layer-by-layer nanoarchitectonics: invention, innovation, and evolution. Chem Lett. 2014;43(1):36–68. doi: 10.1246/cl.130987
- Doshia N, Zahra L, Bhaskar S, et al. Red blood cell-mimicking synthetic biomaterial particles. PNAS. 2009;106:21495–21499. doi: 10.1073/pnas.0907127106
- Shaillender M, Luo R, Venkatraman SS, et al. Layer-by-layer microcapsules templated on erythrocyte ghost carriers. Int J Pharm. 2011;415(1–2):211–217. doi: 10.1016/j.ijpharm.2011.06.011
- Guo J, Agola JO, Serda R, et al. Biomimetic rebuilding of multifunctional red blood cells: modular design using functional components. ACS Nano. 2020;14(7):7847–7859. doi: 10.1021/acsnano.9b08714
- She S, Li Q, Shan B, et al. Fabrication of red-blood-cell-like polyelectrolyte microcapsules and their deformation and recovery behavior through a microcapillary. Adv Mater. 2013;25(40):5814–5818. doi: 10.1002/adma.201302875
- Yu C, Qian D, Huang X, et al. Construction of biconcave hemoglobin-based microcapsules and electrochemical evaluation for its ability of oxygen carry. Sens Actuators B Chem. 2018;256:217–225. doi: 10.1016/j.snb.2017.09.166
- Gratton SEA, Pohlhaus PD, Lee J, et al. Nanofabricated particles for engineered drug therapies: a preliminary biodistribution study of PRINT™ nanoparticles. J Controlled Release. 2007;121:10–18. doi: 10.1016/j.jconrel.2007.05.027
- Merkel TJ, Jones SW, Herlihy KP, et al. Using mechanobiological mimicry of red blood cells to extend circulation times of hydrogel microparticles. Proc Natl Acad Sci. 2011;108:586–591. doi: 10.1073/pnas.1010013108
- Chen K, Merkel TJ, Pandya A, et al. Low modulus biomimetic microgel particles with high loading of hemoglobin. Biomacromolecules. 2012;13(9):2748–2759. doi: 10.1021/bm3007242
- Alayash AI. Blood substitutes: why haven’t we been more successful? Trends Biotechnol. 2014;32(4):177–185. doi: 10.1016/j.tibtech.2014.02.006
- Chen J-Y, Scerbo M, Kramer G. A review of blood substitutes: examining the history, clinical trial results, and ethics of hemoglobin-based oxygen carriers. Clinics. 2009;64(8):803–813. doi: 10.1590/S1807-59322009000800016
- Li T, Jing X, Huang Y. Polymer/Hemoglobin assemblies: biodegradable oxygen carriers for artificial red blood cells. Macromol biosci. 2011;11:865–875. doi: 10.1002/mabi.201000469
- Klibanov AL, Maruyama K, Beckerleg AM, et al. Activity of amphipathic poly(ethylene glycol) 5000 to prolong the circulation time of liposomes depends on the liposome size and is unfavorable for immunoliposome binding to target. Biochim Biophys Acta - Biomembr. 1991;1062(2):142–148. doi: 10.1016/0005-2736(91)90385-L
- Sun J, Huang Y, Shi Q, et al. Oxygen carrier based on hemoglobin/Poly(L-lysine)- block -poly(L -phenylalanine) vesicles. Langmuir. 2009;25:13726–13729. doi: 10.1021/la901194k
- Wang S, Yuan F, Chen K, et al. Synthesis of hemoglobin conjugated polymeric micelle: a ZnPc carrier with oxygen self-compensating ability for photodynamic therapy. Biomacromolecules. 2015;16(9):2693–2700. doi: 10.1021/acs.biomac.5b00571
- Wu B, Sun Z, Wu J, et al. Nanoparticle-stabilized oxygen microcapsules prepared by interfacial polymerization for enhanced oxygen delivery. Angew Chem Int Ed. 2021;60:9284–9289. doi: 10.1002/anie.202100752
- Komatsu T. Albumin-Heme Oxygen Carriers. Hemoglobin-based oxygen carriers as red cell substitutes and oxygen therapeutics. Berlin, Heidelberg: Springer Berlin Heidelberg; 2013. p. 339–348. doi: 10.1007/978-3-642-40717-8_18
- Liu Y, Zhao X, Zhao C, et al. Responsive porous microcarriers with controllable oxygen delivery for wound healing. Small. 2019;15(21):1901254. doi: 10.1002/smll.201901254
- Zhang X, Chen G, Liu Y, et al. Black phosphorus-loaded separable microneedles as responsive oxygen delivery carriers for wound healing. ACS Nano. 2020;14(5):5901–5908. doi: 10.1021/acsnano.0c01059
- Wang Q, Gong J, Bai Q, et al. Hemoglobin coated oxygen storage metal-organic framework as a promising artificial oxygen carrier. J Mater Chem B. 2021;9:4002–4005. doi: 10.1039/D1TB00328C
- An HZ, Safai ER, Burak Eral H, et al. Synthesis of biomimetic oxygen-carrying compartmentalized microparticles using flow lithography. Lab Chip. 2013;13(24):4765. doi: 10.1039/c3lc50610j
- Lee H-Y, Kim H-W, Lee JH, et al. Controlling oxygen release from hollow microparticles for prolonged cell survival under hypoxic environment. Biomaterials. 2015;53:583–591. doi: 10.1016/j.biomaterials.2015.02.117
- Jung AR, Park YH, Kim GE, et al. Stem cell/oxygen-releasing microparticle enhances erectile function in a cavernous nerve injury model. Tissue Eng Part A. 2021;27(1–2):50–62. doi: 10.1089/ten.tea.2019.0240
- Zhu Y, Chian KS, Chan-Park MB, et al. Protein bonding on biodegradable poly(l-lactide-co-caprolactone) membrane for esophageal tissue engineering. Biomaterials. 2006;27(1):68–78. doi: 10.1016/j.biomaterials.2005.05.069
- Wu T, Zheng H, Chen J, et al. Application of a bilayer tubular scaffold based on electrospun poly (L-lactide-co-caprolactone)/collagen fibers and yarns for tracheal tissue engineering. J Mater Chem B. 2017;5:139–150. doi: 10.1039/C6TB02484J
- Engelhardt E-M, Micol LA, Houis S, et al. A collagen-poly(lactic acid-co-ɛ-caprolactone) hybrid scaffold for bladder tissue regeneration. Biomaterials. 2011;32(16):3969–3976. doi: 10.1016/j.biomaterials.2011.02.012
- Li Z, Guo X, Guan J. An oxygen release system to augment cardiac progenitor cell survival and differentiation under hypoxic condition. Biomaterials. 2012;33(25):5914–5923. doi: 10.1016/j.biomaterials.2012.05.012
- Khorshidi S, Karkhaneh A. A hydrogel/particle composite with gradient in oxygen releasing microparticle for oxygenation of the cartilage-to-bone interface: modeling and experimental viewpoints. Mater Sci Eng C. 2021;118:111522. doi: 10.1016/j.msec.2020.111522
- Touri M, Moztarzadeh F, Abu Osman NA, et al. Oxygen-releasing scaffolds for accelerated bone regeneration. ACS Biomater Sci Eng. 2020;6(5):2985–2994. doi: 10.1021/acsbiomaterials.9b01789
- Choi J, Hong G, Kwon T, et al. Fabrication of oxygen releasing scaffold by embedding H2O2-PLGA microspheres into alginate-based hydrogel sponge and its application for wound healing. Appl Sci (Switzerland). 2018;8(9):1492. doi: 10.3390/app8091492
- Mahaseth T, Kuzminov A. Potentiation of hydrogen peroxide toxicity: from catalase inhibition to stable DNA-iron complexes. Mutat Res/Rev Mutat Res. 2017;773:274–281. doi: 10.1016/j.mrrev.2016.08.006
- Gholipourmalekabadi M, Zhao S, Harrison BS, et al. Oxygen-generating biomaterials: a new, viable paradigm for tissue engineering? Trends Biotechnol. 2016;34(12):1010–1021. doi: 10.1016/j.tibtech.2016.05.012
- Mohseni-Vadeghani E, Karimi-Soflou R, Khorshidi S, et al. Fabrication of oxygen and calcium releasing microcarriers with different internal structures for bone tissue engineering: solid filled versus hollow microparticles. Colloids Surf B Biointerfaces. 2021;197:111376. doi: 10.1016/j.colsurfb.2020.111376
- Matsuki N, Ichiba S, Ishikawa T, et al. Blood oxygenation using microbubble suspensions. Eur Biophys J. 2012;41:571–578. doi: 10.1007/s00249-012-0811-y
- Pagureva N, Tcholakova S, Rusanova K, et al. Factors affecting the coalescence stability of microbubbles. Colloids Surf A Physicochem Eng Asp. 2016;508:21–29. doi: 10.1016/j.colsurfa.2016.08.012
- Katiyar A, Sarkar K. Stability analysis of an encapsulated microbubble against gas diffusion. J Colloid Interface Sci. 2010;343(1):42–47. doi: 10.1016/j.jcis.2009.11.030
- Reusser TD, Ramirez D, Benninger RK, et al. Designing oxygen microbubbles for treating tumor hypoxia. 2019 IEEE International Ultrasonics Symposium (IUS), Glasgow, Scotland: IEEE; 2019. p. 1338–1341.
- Liu W, Liu T, Zou M, et al. Aggressive man‐made red blood cells for hypoxia‐resistant photodynamic therapy. Adv Mater. 2018;30(35):1802006. doi: 10.1002/adma.201802006
- Cherwin A, Namen S, Rapacz J, et al. Design of a novel oxygen therapeutic using polymeric hydrogel microcapsules mimicking red blood cells. Pharmaceutics. 2019;11(11):583. doi: 10.3390/pharmaceutics11110583
- Dulińska I, Targosz M, Strojny W, et al. Stiffness of normal and pathological erythrocytes studied by means of atomic force microscopy. J Biochem Biophys Methods. 2006;66(1–3):1–11. doi: 10.1016/j.jbbm.2005.11.003
- Simoni J. Artificial oxygen carriers: scientific and biotechnological points of view. Artif Organs. 2009;33:92–96.
- Radisic M, Deen W, Langer R, et al. Mathematical model of oxygen distribution in engineered cardiac tissue with parallel channel array perfused with culture medium containing oxygen carriers. Am J Physiol Heart Circ Physiol. 2005;288(3):H1278–H1289. doi: 10.1152/ajpheart.00787.2004
- Qadir MMF, Álvarez-Cubela S, Weitz J, et al. Long-term culture of human pancreatic slices as a model to study real-time islet regeneration. Nat Commun. 2020;11(1):3265. doi: 10.1038/s41467-020-17040-8
- Shoemaker JT, Zhang W, Atlas SI, et al. A 3D cell culture organ-on-a-chip platform with a breathable hemoglobin analogue augments and extends primary human hepatocyte functions in vitro. Front Mol Biosci. 2020;7:568777. doi: 10.3389/fmolb.2020.568777
- Gerecht-Nir S, Radisic M, Park H, et al. Biophysical regulation during cardiac development and application to tissue engineering. Int J Dev Biol. 2006;50(2–3):233–243. doi: 10.1387/ijdb.052041sg
- Pretini V, Koenen MH, Kaestner L, et al. Red blood cells: chasing interactions. Front Physiol. 2019;10:945. doi: 10.3389/fphys.2019.00945
- Tibbles PM, Edelsberg JS. Hyperbaric-oxygen therapy. N Engl J Med. 1996;334:1642–1648. doi: 10.1056/NEJM199606203342506
- Leach RM, Rees PJ, Wilmshurst P. ABC of oxygen: hyperbaric oxygen therapy. Br Med J. 1998;317(7166):1140–1143. doi: 10.1136/bmj.317.7166.1140
- Behnke AR, Shaw LA. The use of oxygen in the treatment of compressed air illness. U S Nav Med Bull. 1937;35:61–73.
- Edwards ML. Hyperbaric oxygen therapy. Part 2: application in disease. J Vet Emerg Crit Care. 2010;20(3):289–297. doi: 10.1111/j.1476-4431.2010.00535_1.x
- Bean JW. Effects of oxygen at increased pressure. Physiol Rev. 1945;25(1):1–147. doi: 10.1152/physrev.1945.25.1.1
- John Kirby P, Snyder J, Schuerer DJ, et al. Essentials of hyperbaric oxygen therapy: 2019 review. Mo Med. 2019;116:176–179. doi: 10.1152/japplphysiol.90991.2008
- Raut MS, Maheshwari A. Oxygen supplementation in acute myocardial infarction: to be or not to be? Ann card anaesth. 2016;19(2):342–344. doi: 10.4103/0971-9784.179594
- Abuzaid A, Fabrizio C, Felpel K, et al. Oxygen therapy in patients with acute myocardial infarction: a systemic review and meta-analysis. Am j med. 2018;131(6):693–701. doi: 10.1016/j.amjmed.2017.12.027
- François Oko Pettis Edinguele W, Barberon B, Poussard J, et al. Middle-ear barotrauma after hyperbaric oxygen therapy: a five-year retrospective analysis on 2,610 patients. Undersea Hyperbaric Med. 2020;47:217–228. doi: 10.22462/04.06.2020.7
- Heyboer M, Wojcik SM, Grant WD, et al. Middle ear barotrauma in hyperbaric oxygen therapy. Undersea Hyperbaric Med. 2014;41:393–397.
- Liu Z, Barber C, Gupta A, et al. Imaging assessment of cardioprotection mediated by a dodecafluoropentane oxygen-carrier administered during myocardial infarction. Nucl Med Biol. 2019;70:67–77. doi: 10.1016/j.nucmedbio.2019.01.004
- Qin X, Zhou Y, Wang Y, et al. Preparation and characterization of protein-loaded PFC nanoemulsions for the treatment of heart diseases by pulmonary administration. Eur J Pharmaceut Sci. 2021;158:105690. doi: 10.1016/j.ejps.2020.105690
- Ma T, Wang Y, Qi F, et al. The effect of synthetic oxygen carrier-enriched fibrin hydrogel on Schwann cells under hypoxia condition invitro. Biomaterials. 2013;34(38):10016–10027. doi: 10.1016/j.biomaterials.2013.09.047
- Wang Y, Qi F, Zhu S, et al. A synthetic oxygen carrier in fibrin matrices promotes sciatic nerve regeneration in rats. Acta Biomater. 2013;9(7):7248–7263. doi: 10.1016/j.actbio.2013.03.024
- Kim HY, Kim SY, Lee HY, et al. Oxygen-releasing microparticles for cell survival and differentiation ability under hypoxia for effective bone regeneration. Biomacromolecules. 2019;20(2):1087–1097. doi: 10.1021/acs.biomac.8b01760
- Hwang SC, Hwang DS, Kim HY, et al. Development of bone regeneration strategies using human periosteum-derived osteoblasts and oxygen-releasing microparticles in mandibular osteomyelitis model of miniature pig. J Biomed Mater Res A. 2019;107(10):2183–2194. doi: 10.1002/jbm.a.36728
- Hunt SD, Elg F. Clinical effectiveness of hemoglobin spray (Granulox ®) as adjunctive therapy in the treatment of chronic diabetic foot ulcers. Diabet Foot Ankle. 2016;7:33101. doi: 10.3402/dfa.v7.33101
- Bai Q, Zheng C, Sun N, et al. Oxygen-releasing hydrogels promote burn healing under hypoxic conditions. Acta Biomater. 2022;154:231–243. doi: 10.1016/j.actbio.2022.09.077
- Chen H, Cheng Y, Tian J, et al. Dissolved oxygen from microalgae-gel patch promotes chronic wound healing in diabetes. Sci Adv. 2020;6(20):eaba4311. doi: 10.1126/sciadv.aba4311
- Dadkhah Tehrani F, Shabani I, Shabani A. A hybrid oxygen-generating wound dressing based on chitosan thermosensitive hydrogel and decellularized amniotic membrane. Carbohydr Polym. 2022;281:119020. doi: 10.1016/j.carbpol.2021.119020
- Nasralla D, Coussios CC, Mergental H, et al. A randomized trial of normothermic preservation in liver transplantation. Nature. 2018;557(7703):50–56. doi: 10.1038/s41586-018-0047-9
- Cypel M, Yeung JC, Liu M, et al. Normothermic ex vivo lung perfusion in clinical lung transplantation. N Engl J Med. 2011;364(15):1431–1440. doi: 10.1056/NEJMoa1014597
- Jägers J, Kirsch M, Cantore M, et al. Artificial oxygen carriers in organ preservation: dose dependency in a rat model of ex‐vivo normothermic kidney perfusion. Artif Organs. 2022;46(9):1783–1793. doi: 10.1111/aor.14264
- Spencer JA, Ferraro F, Roussakis E, et al. Direct measurement of local oxygen concentration in the bone marrow of live animals. Nature. 2014;508(7495):269–273. doi: 10.1038/nature13034
- Ellsworth ML. The red blood cell as an oxygen sensor: what is the evidence? Acta Physiol Scand. 2000;168(4):551–559. doi: 10.1046/j.1365-201x.2000.00708.x
- Liu Y, Wang Q, She P, et al. Chitosan-coated hemoglobin microcapsules for use in an electrochemical sensor and as a carrier for oxygen. Mikrochim Acta. 2016;183(11):2847–2854. doi: 10.1007/s00604-016-1908-2
- Ahn K-S, Lee JH, Park J-M, et al. Luminol chemiluminescence biosensor for glycated hemoglobin (HbA1c) in human blood samples. Biosens Bioelectron. 2016;75:82–87. doi: 10.1016/j.bios.2015.08.018
- Liu Y, Gong J, Wu W, et al. A novel bio-nanocomposite based on hemoglobin and carboxyl graphene for enhancing the ability of carrying oxygen. Sens Actuators B Chem. 2016;222:588–597. doi: 10.1016/j.snb.2015.08.101
- Willemen NGA, Hassan S, Gurian M, et al. Oxygen-releasing biomaterials: current challenges and future applications. Trends Biotechnol. 2021;39:1144–1159. Elsevier Ltd. doi: 10.1016/j.tibtech.2021.01.007