ABSTRACT
Introduction
Nemaline myopathies (NM) represent a group of clinically and genetically heterogeneous congenital muscle disorders with the common denominator of nemaline rods on muscle biopsy. NEB and ACTA1 are the most common causative genes. Currently, available treatments are supportive.
Areas covered
We explored experimental treatments for NM, identifying at least eleven mainly pre-clinical approaches utilizing murine and/or human muscle cells. These approaches target either i) the causative gene or associated genes implicated in the same pathway; ii) pathophysiologically relevant biochemical mechanisms such as calcium/myosin regulation of muscle contraction; iii) myogenesis; iv) other therapies that improve or optimize muscle function more generally; v) and/or combinations of the above. The scope and efficiency of these attempts is diverse, ranging from gene-specific effects to those widely applicable to all NM-associated genes.
Expert Opinion
The wide range of experimental therapies currently under consideration for NM is promising. Potential translation into clinical use requires consideration of additional factors such as the potential muscle type specificity as well as the possibility of gene expression remodeling. Challenges in clinical translation include the rarity and heterogeneity of genotypes, phenotypes, and disease trajectories, as well as the lack of longitudinal natural history data and validated outcomes and biomarkers.
1. Introduction
Nemaline myopathies (NM) are a group of non-dystrophic neuromuscular disorders with the common denominator of nemaline bodies or rods, on muscle biopsy. Like other congenital myopathies (CM), the class of early-onset myopathies the condition belongs to, NM are both clinically and genetically heterogeneous [Citation1]. There are at least 12 known causative genes [Citation2,Citation3]. Whilst the predominance of rods in the context of muscle weakness is sufficient for the diagnosis, rods as such are not specific and may also occur in a number of acquired myopathies (for example, HIV-associated myopathies) [Citation4] and as an additional finding in other genetic CM (for example, RYR1-associated myopathies) [Citation5]. The incidence is estimated to be ~1:50,000 [Citation2,Citation6]. However, corresponding to what has been seen in other CM, this figure likely underestimates the incidence of NM, as milder forms may go unnoticed due to phenotypic heterogeneity, even within the same family [Citation2,Citation7,Citation8].
The spectrum of clinical phenotypes varies in terms of presentation, severity, and progression. For the majority of patients, onset is in childhood, although rare adult-onset forms have been recognized (excluding sporadic late-onset nemaline myopathy which is a different disease entity). Even in the rare cases with apparent adult-onset there have usually been some symptoms present in childhood [Citation9–11]. The severity of the disease includes presentations within the fetal akinesia deformation sequence at the most severe end of the spectrum, with lack of spontaneous movements at birth requiring immediate mechanical ventilation and reduced life expectancy [Citation12–14], to milder forms of NM compatible with ambulation and normal lifespan [Citation10,Citation11,Citation15]. The most common clinical manifestations involve hypotonia of congenital onset with (often disproportionate) bulbar and respiratory involvement in addition to axial and proximal muscle weakness, often with sparing of the extraocular muscles [Citation12,Citation16]. Some patients may have a more prominent distal phenotype and there may also be some overlap with the distal arthrogryposis spectrum [Citation17,Citation18]. A large proportion of patients require feeding support in the form of nasogastric, nasojejunal, or gastrostomy feeds, particularly in early life. These aspects may improve through childhood and with development, although some of the NM may be slowly progressive, particularly later in life [Citation2,Citation19–21]. Despite their marked clinical heterogeneity, NM may show distinct patterns of selective involvement on muscle MRI depending on genetic background, suggesting muscle MRI as a diagnostic tool and potential future biomarker [Citation22–24].
Nemaline rods that are seen on Gömöri trichome staining are the histological hallmark of NM. The rods are located in proximity to Z-lines and are considered to be derived from proteins involved in Z-line assembly and maintenance [Citation16,Citation25]. Both Z-lines and rods have a similar lattice structure and comprise similar proteins, which include α-actinin, actin, tropomyosin, myotilin, γ-filamin, cofilin-2, telethonin and nebulin [Citation16,Citation25]. The precise mechanisms of formation are uncertain, although they have been noted to occur in metabolic conditions (Complex 1 deficiency [Citation26]), certain infections [Citation4], inflammatory conditions [Citation27] and with some drugs (e.g. Zidovudine [Citation28])[Citation29]. Nemaline rods are, however, likely to be an epiphenomenon that does not explain all of the pathological mechanisms of NM [Citation16]. Other relevant mechanisms include altered thin filament calcium sensitivity and impaired thick and thin filament interactions resulting in an excitation-contraction disturbance, as well as more general disturbances in thin filament protein turnover [Citation7,Citation30].
The 12 known NM genes include NEB (nebulin), ACTA1 (alpha actin 1), TPM3 (alpha tropomyosin 3), TPM2 (beta tropomyosin or tropomyosin 2), TNNT1 (Troponin T1), KLHL40 (Kelch-like protein 40), KLHL41 (Kelch-like protein 41), CFL-2 (Cofilin 2), KBTBD13 (kelch repeat and BTB domain containing protein 13), LMOD3 (leiomodin3), MYPN (myopalladin), TNNT3 (troponin T3, fast skeletal type) [Citation2,Citation12,Citation16]. These genes are summarized in and the interaction of their protein products is depicted in . Other genes such as MYO18B (Myosin XVIIIB) and NEFL are associated but not accepted as causative as variants in these genes are not always associated with pathological diagnosis of NM [Citation2,Citation31–34]. Finally, there is a proportion of patients with clinical and pathological features of NM in whom no causative gene variant/s have yet been identified [Citation19].
Figure 1. Sarcomeric localisation of proteins involved in nemaline myopathies.
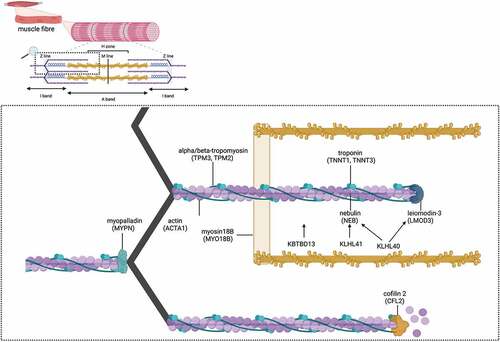
Table 1. Summary of NM genes.
The majority of NM genes encode proteins involved in the structural assembly and maintenance of the thin actin-based filament (TPM3, TPM2, NEB, ACTA1, TNNT1, CFL2) [Citation7]. Muscle fibers in most NM genes exhibit reduced force generation, most pronounced in NEB, ACTA1 and KLHL40-related forms [Citation30], but an increase in the force generated has been reported in exceptional cases [Citation35]. Many of the NM genes undergo alternative splicing, collectively producing many thousands of protein isoforms [Citation36–38].
The most common gene is NEB-NM, accounting for about 35% of NM [Citation2,Citation19,Citation39]. The entire translation of the NEB gene would result in a protein of 8,525 amino-acids, but alternative splicing means that proteins usually contain approximately 6,600 amino-acids [Citation36,Citation40]. The amino acid sequence of nebulin is highly conserved with homology between mice and humans [Citation36,Citation40]. All nebulin mRNAs contain either exon 143 or exon 144 but never both [Citation36,Citation40]. These exons define a super repeat region near the Z-disc anchorage region containing the binding site for kelch-like protein 40 (KLHL40) which in turn binds leiomodin 3 (LMOD3) [Citation36,Citation40,Citation41]. The majority of pathogenic NEB variants are autosomal recessive. In 2014, a study of a NM patient cohort identified 212 disease-causing NEB variants from 159 families. The majority (83%) of these variants were compound heterozygous. The most common types of variants were splice-site mutations (34%), followed by frameshift mutations (32%) caused by small (<20 bp) deletions or insertions, nonsense mutations (23%), and missense mutations (7%). Large deletions and duplications (>1 kb) were rare (4%) [Citation42]. However, novel dominant variants have been described since, for example in a family with an in frame ~100kb deletion with a distal phenotype [Citation18].
The second most common causative gene is ACTA1 which is reported in approximately 24–32% of NM patients [Citation2,Citation19]. The ACTA1 gene encodes alpha actin-1 which cross-links the barbed ends of the actin-based thin filaments of adjacent sarcomeres in the region of the Z-disks at the lateral border of the sarcomere [Citation43]. Disease-causing variants in ACTA1 are usually autosomal dominant, and mostly a de novo occurrence, although recessive changes have also been described [Citation12,Citation20,Citation44,Citation45]. More than 200 disease-causing ACTA1 variants have been identified to date, largely randomly distributed along the coding sequence [Citation20,Citation46]. After NEB and ACTA1, disease causing variants in TPM2, TPM3, KLHL40, KBTBD13, and LMOD3 are somewhat more common than in the other NM genes [Citation2,Citation19].
The current treatment approach for NM is supportive and in line with the general consensus on the standards of care for CM [Citation47]. There are, however, potential therapies at the pre-clinical stages of development targeting various disease mechanisms. The development of therapies in NM is challenging for several reasons: Firstly, the genetic heterogeneity of NM with consequent variation in pathophysiological disease mechanisms means that treatment targets are also somewhat variable. Secondly, there is considerable phenotypic heterogeneity with a paucity of natural history data. Thirdly, there is no consensus on outcome measures or disease biomarkers [Citation2]. The aim of this review is therefore to present the therapeutic options currently in development for NM, along with potential targets, taking into account current understanding of genetic and biological disease mechanisms. We describe therapeutic options categorized based on their mechanism of action. We also present the challenges and opportunities to therapy development associated with the various approaches.
2. Results
2.1. Methods and search strategy
We performed a scoping review beginning with MEDLINE/Pubmed and EMBASE. Selected keywords were combined to create search strategies, with adjustments for each database. Keywords included but were not limited to ‘nemaline myopathy,’ ‘molecular therapy,’ ‘drug therapy,’ ‘gene therapy,’ ‘gene transcription,’ ‘exon skipping’ and ‘RNA splicing.’ The search was limited to English language publications and covered the year 2000 onwards, to include key papers focusing on the genomic structure of NEB, which was first identified in 2004 [Citation36]. Given the aim to comprehensively identify potential therapies, including those in development, conference abstracts were included. More focused searches were also conducted in each sub-category of therapeutic approaches. Particular attention was also given to disease mechanisms to identify potential future therapeutic targets. References from relevant articles were searched for inclusion of additional papers, which were not identified through the primary search strategy. Clinicaltrials.gov was also searched.
2.2. Summary of results
We identified 27 papers or conference abstracts trialing therapies in NM. All studies of therapeutic approaches in NM are at the preclinical stage of development and involve either mouse/zebrafish models in vitro and in vivo, or human tissue in vitro, with some human case reports and case series. Given the genetic heterogeneity of NM, some potential therapeutic mechanisms apply only to specific disease-causing variants, whereas others have a potentially broader application. The majority of studies focus on the disease-causing variations in the NEB and ACTA1 genes. Potential therapies for NM can be widely considered in terms of i) therapies targeting the underlying genetic defect, ii) therapies targeting genes implicated in the same pathway, iii) upstream therapies focusing on myogenesis, iv) therapies targeting the calcium regulation of muscle contraction and v) other pharmacological therapies. In considering potential therapeutic targets, it is also necessary to consider target muscle specificity and distribution and, given that the majority of NM are congenital in onset, the developmental changes affecting potential therapeutic targets. Therapeutic options and the level of evidence for each causative gene is summarized in .
Table 2. Treatment possibilities for each genotype and level of evidence.
2.3. Therapies targeting the underlying genetic defect
We have approached this by exploring Deoxyribonucleic acid (DNA) focused therapies and Ribonucleic acid (RNA) focused therapies separately. Mechanisms and concepts are explained in the sections below.
2.3.1. Therapies targeting DNA
2.3.1.1. Viral vector-mediated gene therapy
Gene therapy aims to correct the underlying genetic defect by introducing exogenous DNA into patient cells to target the defective cellular function. A major limitation of a viral-based gene transfer strategy is the restricted packaging capacity of viruses such as Adeno-Associated Virus (AAV) limited to about 5 kb. For this reason, large genes such as NEB and many of the other NM genes cannot be included in their entirety into the vector [Citation3,Citation48]. This limitation is not unique to NM and has therefore been addressed in other neuromuscular conditions: alternative approaches in Duchenne muscular Dystrophy have focused on the introduction of functional protein fragments or truncated proteins [Citation49]. In NM, the impact of an injected AAV-transported nebulin fragment (i.e. nebulin’s Z disk region and final super-repeat) on the sarcomeric structure and function has been studied in a Neb knockout (KO) murine model. This approach lead to increased Z-disk fragment expression in treated muscle, which was more pronounced in Neb KO mice than wild type. Interestingly, the inserted nebulin Z-disk fragment in wild-type mice was shown to compete with and displace the native full-length nebulin, resulting in decreased muscle force. In Neb KO mice, physiological cross-sectional areas, muscle weight, force production, and contractile kinetics were improved but not significantly. Biochemical data indicated that the progression of NM could be slowed in Neb KO mice as Z-disc width was reduced. Whilst exogeneous nebulin fragments seem to be well tolerated in nebulin-deficient mouse muscle, there were no clear functional benefits [Citation50]. The fact that this approach is possible with some positive results is encouraging. However, further work is required to establish efficacy before translation to clinical medicine.
The feasibility of an AAV gene therapy approach has been assessed for a nonsense disease-causing variant in exon 11 of TNNT1, causing a rare form of NM also known as Amish NM (reflective of its initial and most common reports), with other TNNT1 variants now reported in other populations as well [Citation51,Citation52]. The typical Amish NM presentation has a severe neonatal onset with axial hypotonia, hip and shoulder stiffness and tremors, followed by progressive muscle weakness, atrophy, and contractures. Median survival is 18 months with death commonly due to respiratory failure caused by a combination of thoracic rigidity, pectus carinatum, and restrictive lung disease [Citation53]. The TNNT1 gene is too large to be packaged into an AAV vector in its entirety, and as a consequence, microRNA cassettes have been used to silence the expression of the abnormal Troponin T within cardiac and fast type 2 myofibres. TNNT1 expression is then restricted to slow type 1 myofibres. Preliminary experiments have been conducted in wild-type mice, demonstrating that injection of the vector either intravenously or directly into muscle resulted in reduced protein expression in target locations of cardiac and fast type 2 muscle. This work is ongoing with plans to study the therapeutic efficacy of AAV vectors carrying TNNT1 in Tnnt1 -/- mice [Citation51].
2.3.1.2. CRISPR
Clustered Regularly Interspaced Short Palindromic Repeats (CRISPR) – associated systems (Cas) are tools capable of targeting and editing specific genes. In addition to repairing disease-causing variations in DNA, it can be used in complementary approaches such as genetic compensation through upregulation of surrogate splice isoforms or the deletion of large DNA domains [Citation54,Citation54]. Therefore, CRISPR-Cas9 may be useful to inactivate gain-of-function (dominant) variants as well as correcting other disease-causing variants [Citation54,Citation56,Citation57] and has potential for use in gene-targeted therapies in all NM genes.
Nebulin is arranged into simple repeats or super repeats, with the repeat modules containing conserved SDXXYK-actin-binding motifs. Some pathogenic NEB variants identified to date lead to disruption of the reading frame, predicted to cause mRNA instability, premature truncation, or complete absence of nebulin [Citation36]. One potential approach is to delete repeats that harbour the disease-causing variants with the anticipation that the exon will be skipped and other areas will remain in frame, leading to the production of a truncated nebulin protein that will be somewhat functional. A study is currently planned using CRISPR as a tool to delete exons coding a repeat area in the genome of a zebrafish model of NEB-NM modeling an exon skipping approach [Citation55].
2.3.2. Therapies targeting RNA
2.3.2.1. Antisense oligonucleotides and other approaches aimed at exon skipping
Antisense oligonucleotides can be used to induce exon skipping, although their use is variant specific. In a recent case report, a new homozygous pathogenic NEB variant has been identified affecting intron 144 of the NEB gene. As previously discussed, this is a critical exon, mutually exclusive with exon 143. This variant involves a C>G substitution in the intron, 119 base pairs downstream of exon 144, creating an in-frame deletion and a new donor splice site. It is thought that this new splice site competes with the naturally occurring exon 144 splice site and that the alteration in this critical region is thought to lead to the production of unstable mRNA or truncated nebulin, resulting in a changed amount and/or function of the produced nebulin isoforms [Citation58]. Theoretically, exon skipping could be used to bypass this variant, as exemplified by the experience in RYR1- related myopathy, where a pseudo-exon associated with an unstable RYR1 transcript was successfully removed in vitro [Citation3].
2.3.2.2. Spliceosome‐mediated RNA trans‐splicing (SMaRT)
Spliceosome‐mediated RNA trans‐splicing (SMaRT) corrects genetic variations at the post‐transcriptional level, in the mRNA sequence. The exon-exchange method uses SMaRT to exchange the endogenous mutated exon with an exogenous wild-type exon, carried by an engineered therapy molecule called the Pre-Trans-Splicing Molecule (PTM). SMaRT has already been successfully applied in several models of genetic diseases caused by recessive variants, where correcting a portion of the mutant mRNA pool may lead to a significant therapeutic effect [Citation59]. A PTM has been produced for NEB gene 3’ exon replacement and internal exon replacement in C2C12 and HeLa cell cultures, using NEB minigene pre-mRNA [Citation60] which was then used to achieve exon exchange in endogenous nebulin in mice myoblasts. Although preliminary results were successful, there was only weak trans-splicing. These are the first studies suggesting that nebulin pre-mRNA splicing can be reprogrammed and that endogenous nebulin can undergo exon-exchange [Citation61]. Work is ongoing to assess the efficacy of endogenous exon-exchange on abnormal nebulin transcripts in a zebrafish model [Citation62], representing the first attempt to correct a variant in nebulin pre-mRNA in vivo [Citation62]. These approaches offer promise but are still in very early stages of development.
2.4. Therapies targeting genes implicated in the same pathway
Approaches targeted at genes implicated in the same pathway do not intend to correct the primary cause of the disease, but aim to compensate for the lack of a specific protein by enhancing the expression of related genes. In Spinal Muscular Atrophy (SMA) such an approach targeting the paralogous gene SMN2 has been successful (example Nusinersen and Risdiplam) [Citation63,Citation64]. Approaches targeting DNM-2 in X-linked myotubular myopathy and centronuclear myopathy are also in development [Citation65–67]. A similar approach for NM has been investigated for ACTA1-NM [Citation46,Citation68]. In this context, it is useful to note that some NM genes are paralogues of one another (KLHL40 and KLHL41, TPM2 and TPM3, TNNT1 and TNNT3), indicating that treatments targeting one of these genes may also be useful for the paralog. The potential options offered by this approach are numerous, as there are over 200 paralogous genes identified for NM genes, and with alternative splicing, the number of protein products is correspondingly numerous. The Similarity Matrix of Proteins (SIMAP) database is a protein focused database that provides a comprehensive and up-to-date pre-calculation of the protein sequence similarity matrix, sequence-based features, and sequence clusters. In exploring this database for NM, there are approximately 80 SIMAP similar proteins, with 175 associated genes [Citation69].
2.4.1. Embryonic myosin light chain upregulation
A compensatory approach has been identified in an Acta1H40Y mouse model using an AAV-conducted myosin transgene (Myl4). The aim of this approach is to promote the expression of an embryonic isoform of Myosin Light Chain (i.e.MyLCAa/emb) to compensate for the defective interaction between myosin and actin filaments and restore dysfunctional sarcomere contractility [Citation68]. Some isoforms of MyLC are able to promote force production, where others have a limiting effect. MyLCa/emb is encoded by MYL4 and has been shown to increase the force generating capacity of myosin molecules [Citation70]. This approach has shown positive effects on contractile capacity, muscle mass, and intrinsic force-generating capacity in Acta1H40Y mice [Citation68]. However, in a Neb mouse model, this approach has been trialed but was unsuccessful (Unpublished data, J Ochala, H Granzier). It may theoretically be applicable to other forms of NM or even other neuromuscular conditions characterized by impaired myosin cross-bridge function.
2.4.2. Cardiac alpha-actin upregulation
Dominant pathogenic ACTA1 variants lead to production of a deleterious protein [Citation46], while recessive variants can lead to complete absence of skeletal muscle alpha-actin protein [Citation3,Citation71]. In patients with recessive ACTA1-NM, the absence of skeletal muscle alpha-actin in some cases is compensated for by spontaneous upregulation of cardiac alpha-actin protein isoforms in skeletal muscle [Citation3]. The ACTC1 gene encodes cardiac alpha actin (ACTC) which differs from skeletal alpha-actin by only 4 amino acids [Citation71]. ACTC is the predominant actin isoform in fetal skeletal muscle, and it is down regulated by the time of birth [Citation71,Citation72]. Based on these observations, the effect of cardiac alpha-actin upregulation has been investigated in three mouse models of Acta1-NM; an Acta1 KO model and models of the dominant variants Acta1D286G and Acta1H40Y [Citation46,Citation71]. These experiments involved the production of transgenic mouse lines to create mice lacking Acta1 but expressing the Actc1 transgene [Citation71]. The Acta1 KO model showed improved survival to within the normal range and no gross pathological features of skeletal muscle on biopsy. These mice also appeared to have no impairment in grip strength, rotarod, or locomotor activity [Citation71]. In the most severely affected Acta1D286G models there was also improved survival and body-weight. While the Acta1H40Y model showed no improvement, it presented endogenously elevated levels of cardiac alpha actin in skeletal muscles [Citation46]. Although this approach appears promising, the variability of results between variants needs further delineation before translation into clinical practice. The translational potential of CRISPR-mediated ACTC1 over-expression is also being investigated as a therapy in ACTA1-NM, with the aim to enhance the transcription and expression of endogenous ACTC1 in several cell lines [Citation54].
2.5. Upstream therapies focusing on myogenic potential
2.5.1. Myostatin inhibition
Myostatin inhibition aims to increase muscle mass without correcting the underlying genetic defect. It could therefore function as a general therapy offering temporary symptomatic improvement for many forms of neuromuscular disorders [Citation2]. Myostatin, also known as growth/differentiation factor-8 (GDF-8), is a member of the tumor growth factor ß (TGF-ß) family. Myostatin binds to and signals through the activin type IIB receptor (ActRIIB), which downregulates several key processes related to myofiber hypertrophy. It also activates the TGF-β pathway, preventing progression through the cell cycle [Citation73–75]. Several points in this pathway may be therapeutically targeted. Therapeutic approaches focusing on myostatin include: i) monoclonal antibodies targeting myostatin, (ii) monoclonal antibodies targeting myostatin’s receptor AcvRIIB, (iii) AcvRIIB decoys, and (iv) follistatin overexpression functioning as a myostatin antagonist [Citation76]. A number of molecules, developed to block myostatin pathways, have been studied in other neuromuscular conditions and are currently undergoing pre-clinical and early clinical trials [Citation77–79]. Initial results from these studies did not show as much improvement as expected, and this was attributed to the down-regulation of all components of the myostatin signaling pathway in myopathies and in muscle atrophy in general [Citation76].
However, further efforts dedicated to this approach are ongoing. Three studies investigated different myostatin inhibitors in Acta1 and Neb murine models [Citation74,Citation80,Citation81]. In one of these, a myostatin inhibitor developed by Pfizer, mRK35, has been trialed in a Acta1D286G mouse model of NM. In this study, Acta1D286G and age matched wild type (WT) C57Bl/6 J (WT C57) mice were injected once per week with 10 mg/kg mRK35 until 6 months of age. The mRK35 treated Acta1D286G mice showed an overall increase in body weight, skeletal muscle mass, myofiber size, forelimb grip strength and extensor digitorum longus (EDL) muscle fiber force. Forelimb grip was preserved in untreated mice. These studies illustrate the potential of myostatin inhibition to promote potentially useful muscle growth in the context of Acta1 mutations [Citation74]. In the other two studies, a similar approach focused on a different point in the myostatin pathway, the ActRIIB receptor, in an Acta1H40Y mouse model. Acta1H40Y and WT mice were injected with the activin type IIB receptor, ActRIIB–mFc (Acceleron) to inhibit myostatin signaling. Treatment of Acta1H40Y mice produced significant improvement in body mass, muscle mass, quadriceps myofiber size, and survival, but other measurements of strength (forelimb grip strength, ex vivo measurements of contractile function) did not improve [Citation81]. ActRIIB–mFc (Acceleron) has also been investigated in a Neb knockout mouse model, but there was no response regarding weight gain, strength, myofiber size, or hypertrophic pathway signaling [Citation80]. Both of these compounds have been patented for use in other conditions [Citation82].
2.5.2. Insulin-like growth factor 1(IGF-1)
LMOD3 encodes leiomodin 3. Leiomodins are members of the tropomodulin protein family and localize to the thin filament[Citation83]. In addition to this structural role, leiomodin has a role in myoblast differentiation and proliferation [Citation84]. In an in vitro model using C2C12 myoblasts, knockdown of LMOD3 has been shown to impair myoblast differentiation and lead to apoptosis. Conversely, forced expression of LMOD3 in C2C12 myoblasts has been shown to lead to activation of the AKT (Protein kinase B) and extracellular signal-regulated kinase (ERK) differentiation and proliferation pathways [Citation84]. These pathways are initiated by IGF-1 [Citation85,Citation86]. They overlap with the myostatin pathway. In the C2C12 LMOD3 knockdown model described, administration of IGF-1 ameliorated some of the inhibitory effect on cell proliferation and is therefore a potential therapeutic option [Citation84]. One benefit of this strategy is the fact that recombinant human IGF-1 has already been commercially developed and is widely used in the pediatric population, for example in the context of IGF-1 deficiency [Citation87]. The issue with this approach is that IGF-1 promotes growth of almost every cell type, particularly; muscle, cartilage, bone, liver, kidney, nerve, skin, haematopoietic and lung cells. One potential solution to this challenging problem is suggested by a study where a skeletal muscle-specific enhancer was incorporated in the regulatory region of Igf1 which upregulates IGF1 expression and induces skeletal muscle hypertrophy in C2C12 cell colonies [Citation88].
2.6. Therapies targeting the calcium regulation of muscle contraction
Variation of Ca2+ concentration in the cytoplasm plays a key role in the contraction and relaxation of muscle fibers. Once the sarcolemma is depolarized following an action potential, calcium ions are released from the sarcoplasmic reticulum (SR), leading to the opening of calcium-sensitive calcium channels that accelerate the increase of Ca2+ concentration. Calcium ions then bind to troponin C which is part of a blocking complex of troponin and tropomyosin isoforms associated with actin. The binding of calcium triggers a conformational change in the troponin/tropomyosin complex, resulting in exposure of the myosin head binding site on the actin molecule. A cross bridge can then form between actin and myosin heads with muscle shortening driven by chemical energy from adenosine triphosphate ATP hydrolysis [Citation1,Citation89–91]. At low to mid frequencies of neuromuscular activation, intracellular SR Ca2+ release and the force produced increase proportionally, but at higher frequencies troponin is saturated with calcium and a given increase in calcium leads to diminishing increases in force [Citation92].
The troponin complex consists of troponin C, troponin I and troponin T [Citation91,Citation92]. The troponin I subunit binds to actin and inhibits actin-myosin interaction. Subunit T binds the troponin complex to the tropomyosin complex and is involved in Ca2+ mediated activation of actomyosin ATPase activity [Citation93,Citation94]. The troponin complex is considered to have a regulatory role and acts as a calcium sensor [Citation90]. Tropomyosin subtypes are noted to have a stabilizing role [Citation95]. The excitation-contraction coupling cycle is then terminated through sarco/endoplasmic reticulum Ca2+-ATPase mediated calcium re-uptake into the SR [Citation1,Citation96].
2.6.1. Fast troponin activators
Lower calcium sensitivity of force generation has been identified as a factor contributing to weakness in nebulin deficient muscle. Enhancing calcium sensitivity of force generation has therefore been proposed as a target to improve muscle function [Citation7]. Fast troponin activators act to increase troponin Ca2+ affinity, thus increasing myofibrillar Ca2+sensitivity. Compounds identified as fast troponin activators include Tirasemtiv (formerly known as CK-2017357) [Citation7], and structural analog CK-2127107 (CK-107), CK-2066260 and Reldesemtiv [Citation90,Citation97,Citation98]. They slow the rate of calcium release from the troponin complex which sensitizes the sarcomere to calcium, increasing fast skeletal muscle contractility [Citation98]. Pharmaceutical patents exist in the United States for Tirasemtiv and Reldesemtiv for their use in other conditions [Citation99,Citation100]. Tirasemtiv has been investigated more extensively for amyotropic lateral sclerosis and myasthenia gravis [Citation7,Citation90,Citation97,Citation101], providing useful information regarding safety and pharmacokinetics.
Four studies presented pre-clinical data on use of tirasemtiv and a structural analog of tirasemtiv, CK-2066260, in NEB and ACTA1-NM, including human muscle samples in vitro [Citation7,Citation102] and murine models in vivo and in vitro [Citation7,Citation102–104]. All studies identified that baseline force of contraction in NM muscle compared to control was most impaired at lower stimulation frequencies. These studies also showed that Tirasemtiv and CK-2066260 were most effective at lower stimulation frequencies in the range of 20–40 Hz [Citation7,Citation102,Citation103]. These findings have been replicated with the use of fast skeletal troponin activators in other conditions [Citation98]. Tirasemtiv and CK-2066260 did not significantly alter the magnitude of response of force-pCa (pCa50) curve compared to controls at other stimulation frequencies [Citation7,Citation102,Citation103]. However, when force deficit was calculated this reduced from approximately 70% without tirasemtiv to approximately 40% with tirasemitv in a Neb KO murine model using diaphragm muscle [Citation103].
Mouse and human muscles treated with tirasemtiv or CK-2066260 include diaphragm, gastrocnemius, soleus, and EDL. In these studies, diaphragm, gastrocnemius and EDL consistently responded to tirasemtiv or CK-2066260 with increased force generation, mainly at lower stimulation frequencies [Citation7,Citation102–104]. However, the in vivo data in an Acta1 murine model involving chronic (4 weeks) oral administration of tirasemtiv suggest no effect on diaphragmatic function in terms of tidal volume, minute volume and respiratory rate [Citation102]. The in vivo data for mouse gastrocnemius muscle after acute administration of tirasemtiv, did demonstrate improvement using a footplate force measurement system. In this model, the largest effect of tirasemtiv was noted at 30 Hz where the gastrocnemius force deficit was reduced from 42% to 4% [Citation103].
The chronic administration of oral tirasemtiv in the murine Acta1 model also investigated the effect on overall muscle mass and gastrocnemius cross-sectional area and found no change [Citation7].
In general, it is promising that all of the above studies have shown consistent and comparable improvement although only in specific conditions relating to stimulation frequency, calcium concentration, muscle fibre type, muscle location, and sarcomere length. The stimulation frequency at which fast troponin activators exhibit maximal effect is likely the stimulation frequency of diaphragm muscle [Citation103] and it could be hypothesized that it is the stimulation frequency for day-to-day regulatory muscle action, an important and relevant consideration for NM patients. The current data does not allow for the identification of the potential summative effect of small increases in contractility in one muscle bundle being applied to all muscles of the body for which improvements in the ranges of 5–40% could be significant for patients [Citation7,Citation102–104]. Current data only relates to disease-causing variants in NEB and ACTA1, but the use of fast troponin activators could in principle be beneficial for other genes. It is also important to keep in mind that the numbers of human NM samples studied to date are small (5 samples) and from in vitro experiments [Citation7,Citation102,Citation103]. Two studies used an in vivo mouse model with more clinically translatable end points [Citation102,Citation103].
2.6.2. Calcium sensitisers
In addition to the fast troponin activators, three molecules that increase calcium sensitivity have been trialed in NM, Omecamtiv mecarbil, Levosimendan, and EMD 57033 [Citation105–107]. Omecamtiv mercarbil and levosimendan were originally developed for treatment of cardiac failure with EMD 5703 still in development [Citation108,Citation109]. In myocardial muscle these calcium sensitizers have been shown to increase force of contraction without increasing the intracellular Ca2+ transients or energy consumption [Citation109].
Omecamtiv mecarbil is a small molecule activator of Myh7. As Myh7 is identical in cardiac muscle and in slow type 1 skeletal muscle fibers it was hypothesized that it may be effective in NM muscle. It was trialed in an in vitro and in vivo Neb KO mouse model in soleus muscle fibers due to the slow type 1 fiber predominance of soleus. Similar to fast troponin activators, maximal effect on force generation to a magnitude of 27–37% greater than baseline occurred at 20 Hz, a low stimulation frequency [Citation107]. Similarly, EMD 57033 has been trialed in vitro in two human muscle samples with the TPM2 E41K ß-Tm variant compared to healthy controls. EMD 57033 was found to reverse the negative effects of the E41K β-Tm mutation on the relative force–pCa and relative stiffness–pCa relationships [Citation106]. Both have shown consistently positive results with promise for clinical treatments.
The effect of Levosimendan on muscle fiber contractility has been trialed in vitro in four human quadricep NEB-NM biopsy samples compared to three healthy control samples. Levosimendan was tested at a range of calcium concentrations, and on muscle samples with varying fast type 2 and slow type 1 fiber predominance and no effect on force was observed. There was also no effect on control human cardiac muscle in vitro [Citation105]. Although a small number of patients/samples, the results indicate that Levosimendan does not appear to be a promising option at this stage.
2.6.3. Therapies aimed at reducing calcium sensitivity
The TPM2 gene encodes beta tropomyosin and slow fibers are most affected. The pK7del variant in this gene has been reported in 8 NM patients from five unrelated families and in these individuals increased myofibrillar calcium sensitivity has been noted [Citation7,Citation102,Citation103]. This is in contrast to most other forms of NM where calcium sensitivity is reduced. These 8 patients presented with large joint contractures in childhood and slowly progressive muscle weakness in adulthood. It is hypothesized that progressive muscle contractures are likely to arise from a hyper-contractile phenotype due to increased Ca2+sensitivity of muscle contraction. Biopsies of two unrelated patients with TPM2 pK7del variant were used to investigate calcium force sensitivity relationships and identified consistently increased calcium sensitivity of force generation in type 1 and type 2 myofibers [Citation38]. It is therefore reasonable to consider that therapies that reduce myofibrillar calcium sensitivity may be beneficial [Citation38]. However, apart from the two patient samples investigated in the study above, there have been no further studies investigating this aspect in more detail.
Protein kinase A is an enzyme that phosphorylates troponin I leading to reduced calcium sensitivity [Citation110]. Protein kinase A is activated by cyclic AMP [Citation111] and this pathway could be explored for therapeutic options for specific genes to which this disease mechanism applies. Of note, this disease mechanism highlights the effects of different genetic variants on calcium regulation of muscle contraction within NM and the need to tailor treatment accordingly.
2.7. Other pharmacological therapies
2.7.1. Neuromuscular junction
Abnormal transmission at the neuromuscular junction (NMJ) has been reported in several CM [Citation114], including MTM1, DNM2, BIN1, RYR1, NEB, TTN, KLHL40, TPM2, and TPM3 gene related CM [Citation112–117]. Clinical phenotypes can be heterogeneous in these CM and whilst fatigable weakness may be non-specifically present, decrement on repetitive nerve stimulation is the most distinct neurophysiological finding. Increased jitter on stimulated single fiber electromyography may provide additional support but is a non-specific finding that can be seen several neuromuscular disorders [Citation114,Citation118].
Fragmented and abnormal NMJs with deregulated expression of acetylcholine receptor subunits were noted in an Acta1 KO mouse model. In addition, electrophysiological studies on the Acta1 KO mouse diaphragm showed a decremental response to repetitive stimulation [Citation119]. Pre-clinical research has identified the role of the E3 ubiquitin ligase Cullin-3, as essential for the development of the NMJ. Lack of Cullin-3 leads to accumulation of non-muscle α-actinins, as observed clinically with early-onset KBTBD13-NM [Citation120].
One case report of a KLHL40-NM described sustained benefit with pyridostigmine and deterioration of clinical symptoms on withdrawal [Citation115]. Positive responses to treatment with pyridostigmine have been reported in cases of NM due to variants in KLHL40, TPM2, TPM3, and also in RYR1-CM [Citation115,Citation117]. The exact mechanism leading to defective NMJ transmission in these CM is not completely understood. In other CM, such as centronuclear myopathies, the proposed mechanisms include paucity of synaptic vesicles and simplified post-synaptic clefts with acetylcholine receptor deficiency, leading to a defective pre- and post-synaptic NMJ and impaired neuromuscular transmission [Citation117,Citation121]. These are potential targets to improve NMJ transmission which could also be utilized in NM. Finally, it has to be noted that mild responses to pyridostigmine may occur non-specifically in a range of neuromuscular disorders, and are not unequivocal proof of NMJ involvement.
2.7.2. Tyrosine
The conversion of tyrosine into L-dopa is the rate-limiting step in the production of catecholamine neurotransmitters dopamine, norepinephrine, and epinephrine. L-tyrosine is the only metabolically active form of this amino acid in humans. Improvement in body weight and muscle strength with L-tyrosine supplementation has been reported in an Acta1 mouse knock out model [Citation122]. However in another study where Acta1D286G zebrafish and mice were given L-tyrosine supplementation, there was no improvement in physical abilities of these animals and also no improvement in energy metabolism, mechanical properties or muscle atrophy [Citation123]. A case report from 1989 described a father and son with NM who had dramatic and sustained improvement in sialorrhea and muscle strength with L-tyrosine, with worsening on discontinuation [Citation124]. A case series of 5 patients with NM (one with TPM3-NM and 4 with an unknown gene) reported clear improvement in sialorrhea with possible improvement in muscle strength, which was sustained for up to 5 years in those who continued treatment [Citation125,Citation126] and no deterioration was noted on discontinuation. L-tyrosine was well tolerated without any side effects and in all treated patients the improvement was within 72 hours. The improvement in mobility and reduction in drooling may also reflect increased central dopaminergic activity as the activation of noradrenergic and dopaminergic receptors are known to improve locomotion in mammals [Citation122,Citation125,Citation126]. A zebrafish Neb-NM model showed no effect. Further evidence is required at both pre-clinical and clinical stages to determine the efficacy and understanding of this possible therapeutic option.
2.7.3. L- carnitine
L-Carnitine is an amino acid produced from the essential amino acids lysine and methionine. It is a co-factor in the synthesis of several enzymes required for the conversion of free long-chain fatty acids to acylcarnitine, and their transport into the mitochondria where they enter the Krebs cycle. The absence of L-carnitine results in toxic accumulation of free fatty acids in the cytoplasm and energy failure. In patients with primary carnitine deficiencies and some secondary deficiencies such as organic acidurias, L-carnitine supplementation is known to improve muscle weakness. L-carnitine is also reported to improve exercise stamina in mitochondrial myopathies [Citation127]. There is a case report describing a single patient with ACTA1-NM and a carnitine deficiency who improved with carnitine supplementation [Citation128]. It is not clear if the two occurrences were coincidental as carnitine levels varied. There is also a recent report of a Tpm3 transgenic zebrafish model supplemented with L-carnitine that showed improvement in muscle endurance and swimming speed [Citation129], yet another zebrafish Neb-NM model showed no effect [Citation130]. These observations raise the hypothesis of whether abnormalities in carnitine metabolism could contribute to muscle weakness in NM [Citation128]. Again, further evidence is required at both pre-clinical and clinical stages to determine the efficacy and understanding of this possible therapeutic option.
2.7.4. Other potential pharmacological therapies
A large-scale chemical screen has been trialed in a Neb-NM zebrafish model. Ninety-six compounds were tested. Eight were initially identified as positive hits, but this was not repeated in duplicate testing and it was felt that this method did not contribute any additional therapeutic options [Citation131]. An N-ethyl-N-nitrosourea (ENU)-based genetic screen has also been performed in a Neb-NM mouse model (exon 55 deletion) to try to identify gene modifiers. No candidate therapies were identified.
3. Conclusion
There are limited treatment options currently available for NM, hence the unmet need for this group of patients. Despite this, there are drug targets and treatment options in development. Some options are very specific while others are more generic. The treatment options focusing on the causative gene hold significant promise, but others such as fast troponin activators, pyridostigmine, myostatin inhibitors may also offer improvement in appropriately selected cases. Combinations of treatments may also be used to complement one another.
4. Expert opinion
NM are challenging conditions from a therapeutic point of view, largely due to their significant clinical and genetic heterogeneity. Nevertheless, there are numerous drug targets that could be usefully exploited in any genotype or disease mechanism.
Clinical therapy development in these highly diverse conditions will need to take various considerations; in particular biochemical properties specific to the abnormal skeletal muscle and muscle fiber types, changes in treatment targets with growth and development, and avoidance of adverse effects on cardiac muscle, smooth muscle, or other organs.
The first level of specificity to consider relates to the muscle fibers type that need to be targeted: slow type 1 versus fast types 2A, 2X, and 2B [Citation132,Citation133]. For the majority of NM, fast, type 2 fibers are most affected () [Citation30,Citation90]. Sequence homology between fast and slow myosin and troponins is approximately 50%[Citation90].
A second level of specificity to consider relates to the need to target skeletal rather than cardiac striated muscle or smooth muscle: Fast skeletal muscle fibers have different myosin and troponin isoforms compared to cardiac and slow skeletal muscle. Smooth muscle does not express troponin [Citation90], whereas Troponin T, encoded by NM genes TNNT1 and TNNT3 are predominantly expressed in skeletal but not cardiac muscle [Citation93,Citation134]. Troponin C is encoded by the gene TNNC1 in both cardiac and skeletal muscle, whereas cardiac troponin T and I are encoded by different genes, TNNI3 and TNNT2 [Citation135], and have amino acid sequences different from their skeletal muscle counterparts with a discrepancy of up to 40%. LMOD3 is expressed predominantly in skeletal muscle although there is some cardiac expression [Citation83]. In many of the treatment approaches discussed, side effects from therapies also acting on cardiac and smooth muscle must be considered but, considering that majority of NM causative genes are only expressed in skeletal muscle (), these side effects are unlikely in gene-specific approaches [Citation16,Citation46]. However, cardiac side effects may be more likely with other approaches such as with drugs affecting calcium sensitivity and IGF-1 [Citation109,Citation136].
A third level of specificity to consider is related to the time-dependence of the potential mechanism of correction. Alternative splicing of NM genes throughout development is an important mechanism that should be investigated in more detail, as it may help better understand disease mechanisms and aid translational efforts. The best example for this consideration is illustrated by NEB age-dependent alternate-splicing: as previously explained, the splicing of exons 143 and 144 are mutually exclusive. Isoforms containing exon 144 are the default in myogenesis, while isoforms containing exon 143 are expressed from approximately 12 weeks gestation in humans, and very strongly expressed by 17 weeks [Citation40].
Another consideration is the protein levels needed to maintain a healthy neuromuscular phenotype: A nebulin knockout mouse model demonstrated that nebulin levels declined rapidly in the first weeks of life. Compared to controls, the average knockout nebulin levels at 3 weeks of age were reduced to 48%, to 4.8% at 5 weeks and to 2.2% at 6 months[Citation137]. In the nebulin mouse model described earlier, muscle weight assessments during growth revealed that all muscles were of lower mass than that of control muscle initially. However, from 15 to 25 weeks of age most had increased from baseline, and some muscles such as diaphragm were of equivalent mass to that of controls and soleus was even slightly heavier, implying hypertrophy [Citation138]. Although there was no data to indicate how the hypertrophied soleus muscle performed in terms of force generation and contractility, the observations on muscle hypertrophy may still be useful in the context of therapies aimed at increasing muscle growth such as anti-myostatin agents, IGF-1 or upregulation of IGF-1 in muscle.
Less specific approaches such as fast troponin activators, pyridostigmine, and myostatin inhibitors bypass these different pitfalls and allow for more straightforward clinical development as toxicology studies focusing on these compounds have already been conducted in other conditions. Nevertheless, their potential to constitute a real long-term disease-modifying approach remains largely to be demonstrated. In several other conditions, such as Duchenne muscular dystrophy, facio-scapulo humeral dystrophy and inclusion body myositis, the efficacy of myostatin inhibitors could not be demonstrated75. Similarly, L carnitine and pyridostigmine that were tested in clinical trials in spinal muscular atrophy, based on similar pre-clinical rationale, were not shown to be efficient.
The clinical translation of these different approaches will be challenging, given the rarity of patients, the broad phenotypic spectrum with heterogenous progression, and the lack of robust longitudinal natural history study (NHS) data. Considering that NM tends to be stable or slowly progressive over time, disease stabilization will be hard to demonstrate in a reasonable time frame, and statistical analysis will need to be designed on the demonstration of a significant improvement. This will be achievable with a reasonable number of patients only with very sensitive outcome measures. In this context, the conduct of an informative NHS is essential. In other conditions such as SMA [Citation138] or X linked myotubular myopathy [Citation139], well-designed longitudinal NHS have been key to demonstrate the sensitivity of the different outcomes, different patients trajectories, and design new clinical trials based on Bayesian statistics [Citation140], allowing the comparison of patients with their projected evolution rather than with the evolution of another patient.
Gene editing and gene replacement therapy constitute exciting approaches, and the potential of gene therapy to transform the phenotype of patients with congenital myopathy has recently been highlighted in X-linked myotubular myopathy. Nevertheless, gene therapy can also come with significant adverse reactions [Citation141], which are difficult to accept in the context of relatively stable conditions like congenital myopathies.
What is particularly exciting in the field of the NM is the fact that many of the approaches discussed in this review are still in development at present. Much of the data is currently only available from scientific conference presentations with the anticipation of substantial therapy development in coming years. Given the broad spectrum of mechanisms of action, combinations of drugs with individually demonstrated efficacy, could be considered [Citation142]. Taken together, these ongoing therapeutic developments hold promise for this still incurable form of myopathy.
Article highlights
Nemaline myopathies (NM) are a group of heterogeneous muscle disorders with nemaline bodies or rods on muscle biopsy as the common histopathological denominator.
There are at least 12 causative genes each with numerous disease-causing variants. Many genes exhibit alternative splicing with multiple protein isoforms produced. The most common causative genes are NEB and ACTA1.
All available data to date are preclinical or involve case reports/case series with data from mice, zebrafish, and humans.
AAV Gene therapies for NM involving expression of a Z-disc fragment have been trialed in a Neb knockout (KO) murine model; however, whilst the fragment was expressed, it did not lead to functional benefits. In Tnnt1 -/- mice, microRNA cassettes packaged in an AAV vector are being studied with the aim of silencing abnormal Troponin T production.
Viral vector gene therapies for NM are still in early preclinical phases of development and do not yet show clinical feasibility.
Spliceosome‐mediated RNA trans‐splicing (SMaRT) to generate exon exchange is promising, but still in very early stages of preclinical development.
Antisense oligonucleotides used in an exon skipping approach are theoretically possible, but none have been developed yet.
Additional work is ongoing in a zebrafish model to assess the efficacy of endogenous exon-exchange on abnormal Neb transcripts. CRISPR-mediated ACTC1 over-expression is being developed as a therapy in ACTA1-NM. This offers clinical promise, but results are not consistent.
Embryonic isoform of Myosin Light Chain upregulation may act to improve myosin contractility. There is encouraging data in Acta1 mice, but not in Neb mice.
Other approaches targeting the myostatin pathway have been investigated with mixed results in Neb and Acta1.
Fast troponin activators work by increasing calcium sensitivity of muscle contraction, a common disease mechanism in NM. Results consistently show improvement although only at lower frequencies of stimulation and limited to force and contractility, with limited evidence relating to functional effects, but could offer promise and improve quality of life for most NM patients.
Neuromuscular junction defects are implicated in some NM genotypes. There is evidence from case series that Pyridostigmine may be helpful.
L-carnitine and L-tyrosine have been explored as drugs to improve muscle function. Available data are limited to preclinical studies and case reports. Level of evidence is varied.
This box summarizes key points contained in the article.
Declaration of Interests
The authors have no relevant affiliations or financial involvement with any organization or entity with a financial interest in or financial conflict with the subject matter or materials discussed in the manuscript. This includes employment, consultancies, honoraria, stock ownership or options, expert testimony, grants or patents received or pending, or royalties.
Reviewer disclosures
Peer reviewers on this manuscript have no relevant financial or other relationships to disclose.
Acknowledgments
The figure was created using BioRender.com under monthly paid subscription. The research was supported by the NIHR Oxford Biomedical Research Centre. The views expressed are those of the author(s) and not necessarily those of the NHS, the NIHR or the Department of Health.
Additional information
Funding
References
- Jungbluth H, Treves S, Zorzato F, et al. Congenital myopathies: disorders of excitation–contraction coupling and muscle contraction. Nat Rev Neurol. 2018;14(3):151–167.
- Neuhaus SB, Wallgren-Pettersson C, Bönnemann CG, et al. 250th ENMC INTERNATIONAL WORkshop: clinical trial readiness in nemaline myopathy 6–8 September 2019, Hoofdorp, the Netherlands. Neuromuscul Disord. 2020;30(10):866–875.
- Jungbluth H, Muntoni F. Therapeutic aspects in congenital myopathies. Semin Pediatr Neurol. 2019;29:71–82.
- Madonia P, Wilson J, Bican O, et al. HIV, rods, and the muscles–a discussion about HIV-associated nemaline rod myopathy. J La State Med Soc. 2012;164(6):320–323.
- Monnier N, Romero NB, Lerale J, et al. An autosomal dominant congenital myopathy with cores and rods is associated with a neomutation in the RYR1 gene encoding the skeletal muscle ryanodine receptor. Hum Mol Genet. 2000;9(18):2599–2608.
- Wallgren-Pettersson C, Laing NG. Report of the 70th ENMC international workshop: nemaline myopathy. Neuromuscul Disord. Naarden, The Netherlands. 1999 11-13 June ;10(4–5):299–306.
- de Winter JM, Buck D, Hidalgo C, et al., Troponin activator augments muscle force in nemaline myopathy patients with nebulin mutations. J Med Genet. 2013;50(6):383–392. .
- Wallgren-Pettersson C, Pelin K, Nowak KJ, et al. Genotype-phenotype correlations in nemaline myopathy caused by mutations in the genes for nebulin and skeletal muscle alpha-actin. Neuromuscul Disord. 2004;14(8–9):461–470.
- Kao JC, Liewluck T, Milone M. A novel ACTA1 mutation causing progressive facioscapuloperoneal myopathy in an adult. J Clin Neurosci. 2018;53:261–262.
- Petrucci A, Primiano G, Savarese M, et al. Novel TNNT1 mutation and mild nemaline myopathy phenotype in an Italian patient. Neuromuscul Disord. 2021;31(6):532–538.
- Schatz UA, Weiss S, Wenninger S, et al. Evidence of mild founder LMOD3 mutations causing nemaline myopathy 10 in Germany and Austria. Neurology. 2018;91(18):e1690–e1694.
- Laitila J, Wallgren-Pettersson C. Recent advances in nemaline myopathy. Neuromuscul Disord. 2021;31(10):955–967.
- Lammens M, Moerman P, Fryns JP, et al. Fetal akinesia sequence caused by nemaline myopathy. Neuropediatrics. 1997;28(2):116–119.
- Feingold-Zadok M, Chitayat D, Chong K, et al. Mutations in the NEB gene cause fetal akinesia/arthrogryposis multiplex congenita. Prenat Diagn. 2017;37(2):144–150.
- Seferian AM, Malfatti E, Bosson C, et al. Mild clinical presentation in KLHL40-related nemaline myopathy (NEM 8). Neuromuscul Disord. 2016;26(10):712–716.
- Sewry CA, Laitila JM, Wallgren-Pettersson C. Nemaline myopathies: a current view. J Muscle Res Cell Motil. 2019;40(2):111–126.
- Davidson AE, Siddiqui FM, Lopez MA, et al. Novel deletion of lysine 7 expands the clinical, histopathological and genetic spectrum of TPM2-related myopathies. Brain. 2013;136(2):508–521.
- Kiiski KJ, Lehtokari V-L, Vihola AK, et al. Dominantly inherited distal nemaline/cap myopathy caused by a large deletion in the nebulin gene. Neuromuscul Disord. 2019;29(2):97–107.
- Amburgey K, Acker M, Saeed S, et al. A cross-sectional study of nemaline myopathy. Neurology. 2021;96(10):e1425–e1436.
- Sparrow JC, Nowak KJ, Durling HJ, et al. Muscle disease caused by mutations in the skeletal muscle alpha-actin gene (ACTA1). Neuromuscul Disord. 2003;13(7–8):519–531.
- Jungbluth H, Sewry CA, Brown SC, et al. Mild phenotype of nemaline myopathy with sleep hypoventilation due to a mutation in the skeletal muscle alpha-actin (ACTA1) gene. Neuromuscul Disord. 2001;11(1):35–40.
- Jungbluth H, Sewry CA, Counsell S, et al. Magnetic resonance imaging of muscle in nemaline myopathy. Neuromuscul Disord. 2004;14(12):779–784.
- Warman Chardon J, Díaz-Manera J, Tasca G, et al. MYO-MRI diagnostic protocols in genetic myopathies. Neuromuscul Disord. 2019;29(11):827–841.
- Jungbluth H. Myopathology in times of modern imaging. Neuropathol Appl Neurobiol. 2017;43(1):24–43.
- Luther PK. The vertebrate muscle Z-disc: sarcomere anchor for structure and signalling. J Muscle Res Cell Motil. 2009;30(5–6):171–185.
- Pula S, Urankar K, Norman A, et al. A novel de novo ACTA1 variant in a patient with nemaline myopathy and mitochondrial Complex I deficiency. Neuromuscul Disord. 2020;30(2):159–164.
- Voermans NC, Benveniste O, Minnema MC, et al. Sporadic late-onset nemaline myopathy with MGUS: long-term follow-up after melphalan and SCT. Neurology. 2014;83(23):2133–2139.
- Pezeshkpour G, Illa I, Dalakas MC. Ultrastructural characteristics and DNA immunocytochemistry in human immunodeficiency virus and zidovudine-associated myopathies. Hum Pathol. 1991;22(12):1281–1288.
- Jungbluth H, Voermans NC. Congenital myopathies: not only a paediatric topic. Curr Opin Neurol. 2016;29(5):642–650.
- Winter de JM, Joureau B, Lee E-J, et al., Mutation-specific effects on thin filament length in thin filament myopathy. Ann Neurol. 2016;79(6):959–969. .
- Agrawal PB, Joshi M, Marinakis NS, et al. Expanding the phenotype associated with the NEFL mutation. JAMA Neurol. 2014;71(11):1413–1420.
- Alazami AM, Kentab AY, Faqeih E, et al. A novel syndrome of Klippel-Feil anomaly, myopathy, and characteristic facies is linked to a null mutation in MYO18B. J Med Genet. 2015;52(6):400–404.
- Malfatti E, Böhm J, Lacène E, et al. A premature stop Codon in MYO18B is associated with severe nemaline myopathy with cardiomyopathy. J Neuromuscul Dis. 2015;2(3):219–227.
- Mihaylova V, Chablais F, Herenger Y, et al. Novel truncating mutations of MYO18B causing congenital myopathy in a Swiss patient. Neurol Genet. Internet]. 2020 [cited 2022 Apr 18];6. Available from. ;(4):e458.
- Jain RK, Jayawant S, Squier W, et al. Nemaline myopathy with stiffness and hypertonia associated with an ACTA1 mutation. Neurology. 2012;78(21):1704.
- Donner K, Sandbacka M, Lehtokari V-L, et al. Complete genomic structure of the human nebulin gene and identification of alternatively spliced transcripts. Eur J Hum Genet. 2004;12(9):744–751.
- Ilkovski B, Nowak KJ, Domazetovska A, et al. Evidence for a dominant-negative effect in ACTA1 nemaline myopathy caused by abnormal folding, aggregation and altered polymerization of mutant actin isoforms. Hum Mol Genet. 2004;13(16):1727–1743.
- Mokbel N, Ilkovski B, Kreissl M, et al. K7del is a common TPM2 gene mutation associated with nemaline myopathy and raised myofibre calcium sensitivity. Brain. 2013;136(2):494–507.
- Malfatti E, Romero NB. Nemaline myopathies: state of the art. Rev Neurol . 2016;172(10):614–619.
- Lam LT, Holt I, Laitila J, et al. Two alternatively-spliced human nebulin isoforms with either exon 143 or exon 144 and their developmental regulation. Sci Rep. 2018;8(1):1–10.
- Chu M, Gregorio CC, Pappas CT. Nebulin, a multi-functional giant. J Exp Biol. 2016;219(2):146–152.
- Lehtokari V-L, Kiiski K, Sandaradura SA, et al. Mutation update: the spectra of nebulin variants and associated myopathies. Hum Mutat. 2014;35(12):1418–1426.
- Henderson CA, Gomez CG, Novak SM, et al. Overview of the muscle cytoskeleton. Compr Physiol. 2017;7:891–944.
- Jungbluth H, Sewry C, Muntoni F. The congenital myopathies Rosenberg’s molecular and genetic basis of neurological and psychiatric disease. Elsevier. 2020: 451–461
- Friedman B, Simpson K, Tesi-Rocha C, et al. Novel large deletion in the ACTA1 gene in a child with autosomal recessive nemaline myopathy. Neuromuscul Disord. 2014;24(4):331–334.
- Ravenscroft G, McNamara E, Griffiths LM, et al. Cardiac α-actin over-expression therapy in dominant ACTA1 disease. Hum Mol Genet. 2013;22(19):3987–3997.
- Wang CH, Dowling JJ, North K, et al. Consensus statement on standard of care for congenital myopathies. J Child Neurol. 2012;27(3):363–382.
- Bulcha JT, Wang Y, Ma H, et al. Viral vector platforms within the gene therapy landscape. Signal Transduct Target Ther. 2021;6(1):53.
- Hollinger K, Chamberlain JS. Viral vector-mediated gene therapies. Curr Opin Neurol. 2015;28(5):522–527.
- Li F, Kolb J, Crudele J, et al., Expressing a Z-disk nebulin fragment in nebulin-deficient mouse muscle: effects on muscle structure and function. Skelet Muscle. 2020;10(1):2. .
- D’Ambrosio E, Sena-Esteves M, GrayEdwards H, et al. CONGENITAL MYOPATHIES 1 – NEMALINE: p.29 AAV gene therapy for TNNT1-associated Nemaline myopathy. Neuromuscul Disord. 2020;30:S56.
- Zambon AA, Abel F, Linnane B, et al. Troponin-T type 1 (TNNT1)-related nemaline myopathy: unique respiratory phenotype and muscle pathology findings. Neuromuscul Disord. 2022;32(3):245–254.
- Fox MD, Carson VJ, Feng H-Z, et al. TNNT1 nemaline myopathy: natural history and therapeutic frontier. Hum Mol Genet. 2018;27(18):3272–3282.
- Best H, Woodman K, Lek A, et al. MOLECULAR THERAPEUTIC APPROACHES: o.7Correcting neuromuscular pathogenic variants with CRISPR-Cas9 technology. Neuromuscul Disord. 2019;29:S39–S40.
- Viththiyapaskaran S. CONGENITAL MYOPATHIES 1–NEMALINE: p. 30 Multi-exon skipping as a potential therapy for nemaline myopathy in Zebrafish. Neuromuscul Disord [Internet]. 2020; Available from: https://www.nmd-journal.com/article/S0960-8966(20)30235-2/abstract.
- Liu W, Li L, Jiang J, et al. Applications and challenges of CRISPR-Cas gene-editing to disease treatment in clinics. Precis Clin Med. 2021;4(3):179–191.
- Babačić H, Mehta A, Merkel O, et al. CRISPR-cas gene-editing as plausible treatment of neuromuscular and nucleotide-repeat-expansion diseases: a systematic review. PLoS One. 2019;14(2):e0212198.
- Laflamme N, Lace B, Thonta Setty S, et al. A homozygous deep intronic mutation alters the splicing of nebulin gene in a patient with nemaline myopathy. Front Neurol. 2021;12:660113.
- Yang Y, Walsh CE. Spliceosome-mediated RNA trans-splicing. Mol Ther. 2005;12(6):1006–1012.
- Laitila J, Pelin K. P3.13 Reprogramming nebulin pre-mRNA splicing by spliceosome-mediated RNA trans-splicing. Neuromuscul Disord. 2010;20(9–10):644.
- Laitila J, Dowling JJ, Pelin K. P.9.3 Repair of mutant nebulin transcripts by exon exchange. Neuromuscul Disord. 2013;23(9–10):784.
- Berger A, Maire S, Gaillard M-C, et al. m RNA trans -splicing in gene therapy for genetic diseases. Wiley Interdiscip Rev RNA. 2016;7(4):487–498.
- Baranello G, Darras BT, Day JW, et al. Risdiplam in type 1 spinal muscular atrophy. N Engl J Med. 2021;384(10):915–923.
- Finkel RS, Mercuri E, Darras BT, et al. Nusinersen versus sham control in infantile-onset spinal muscular atrophy [Internet]. N Engl J Med. 2017;377(18):1723–1732.
- Tasfaout H, Lionello VM, Kretz C, et al. Single intramuscular injection of AAV-shRNA reduces DNM2 and prevents myotubular myopathy in mice. Mol Ther. 2018;26(4):1082–1092.
- Zhao M, Maani N, Dowling JJ. Dynamin 2 (DNM2) as cause of, and modifier for, human neuromuscular disease. Neurotherapeutics. 2018;15(4):966–975.
- Buono S, Ross JA, Tasfaout H, et al. Reducing dynamin 2 (DNM2) rescues DNM2 -related dominant centronuclear myopathy. Proc Natl Acad Sci U S A. 2018;115(43):11066–11071.
- Lindqvist J, Levy Y, Pati-Alam A, et al., Modulating myosin restores muscle function in a mouse model of nemaline myopathy. Ann Neurol. 2016;79(5):717–725. .
- Rattei T, Tischler P, Götz S, et al. SIMAP–a comprehensive database of pre-calculated protein sequence similarities, domains, annotations and clusters. Nucleic Acids Res. 2010;38(suppl_1):D223–6.
- Abdelaziz AI, Segaric J, Bartsch H, et al. Functional characterization of the human atrial essential myosin light chain (hALC-1) in a transgenic rat model. J Mol Med. 2004;82(4):265–274.
- Nowak KJ, Ravenscroft G, Jackaman C, et al. Rescue of skeletal muscle α-actin–null mice by cardiac (fetal) α-actin. J Cell Biol. 2009;185(5):903–915.
- Ilkovski B, Clement S, Sewry C, et al. Defining α-skeletal and α-cardiac actin expression in human heart and skeletal muscle explains the absence of cardiac involvement in ACTA1 nemaline myopathy. Neuromuscul Disord. 2005;15(12):829–835.
- Joulia-Ekaza D, Cabello G. Myostatin regulation of muscle development: molecular basis, natural mutations, physiopathological aspects. Exp Cell Res. 2006;312(13):2401–2414.
- Tinklenberg JA, Siebers EM, Beatka MJ, et al., Myostatin inhibition using mRK35 produces skeletal muscle growth and tubular aggregate formation in wild type and TgACTA1D286G nemaline myopathy mice. Hum Mol Genet. 2018;27(4):638–648. .
- Elkina Y, von Haehling S, Anker SD, et al. The role of myostatin in muscle wasting: an overview. J Cachexia Sarcopenia Muscle. 2011;2(3):143–151.
- Mariot V, Joubert R, Hourdé C, et al. Downregulation of myostatin pathway in neuromuscular diseases may explain challenges of anti-myostatin therapeutic approaches. Nat Commun. 2017;8(1):1859.
- Zhou H, Meng J, Malerba A, et al. Myostatin inhibition in combination with antisense oligonucleotide therapy improves outcomes in spinal muscular atrophy. J Cachexia Sarcopenia Muscle. 2020;11:768–782.
- Roche H-L. A study to investigate the safety and efficacy of RO7204239 in combination with risdiplam (RO7034067) in ambulatory children with spinal muscular atrophy [Internet]. Clinicaltrials.gov. 2022 [cited 2022 Apr 27]. Available from: https://clinicaltrials.gov/ct2/show/NCT05115110.
- Scholar Rock, Inc. Efficacy and safety of apitegromab in patients with later-onset spinal muscular atrophy treated with nusinersen or risdiplam (SAPPHIRE) [Internet]. Clinicaltrials.gov. 2022. [cited 2022 Apr 27]. Available from: https://clinicaltrials.gov/ct2/show/NCT05156320?term=apitegromab&draw=2&rank=1.
- Tinklenberg JA, Siebers EM, Beatka MJ, et al. Myostatin inhibition using ActRIIB-mFc does not produce weight gain or strength in the nebulin conditional KO mouse. J Neuropathol Exp Neurol. 2019;78(2):130–139.
- Tinklenberg J, Meng H, Yang L, et al. Treatment with ActRIIB-mFc produces myofiber growth and improves lifespan in the acta1 H40Y murine model of nemaline myopathy. Am J Pathol. 2016;186(6):1568–1581.
- Antagonist antibodies against GDF-8 and uses in treatment of ALS and other GDF-8 associated disorders [Internet]. JUSTIA patents. 2011. [cited 2022 Apr 21]. Available from: https://patents.justia.com/patent/7910107.
- Yuen M, Sandaradura SA, Dowling JJ, et al. Leiomodin-3 dysfunction results in thin filament disorganization and nemaline myopathy. J Clin Invest. 2014;124(11):4693–4708.
- Lin F-H, Wang A, Dai W, et al. Lmod3 promotes myoblast differentiation and proliferation via the AKT and ERK pathways. Exp Cell Res. 2020;396(2):112297.
- Zanou N, Gailly P. Skeletal muscle hypertrophy and regeneration: interplay between the myogenic regulatory factors (MRFs) and insulin-like growth factors (IGFs) pathways. Cell Mol Life Sci. 2013;70(21):4117–4130.
- Coolican SA, Samuel DS, Ewton DZ, et al. Themitogenic and myogenic actions of insulin-like growth factors utilizedistinct signaling pathways. J Biol. 1997;272:6653–6662.
- Grimberg A, DiVall SA, Polychronakos C, et al. Guidelines for growth hormone and insulin-like growth factor-i treatment in children and adolescents: growth hormone deficiency, idiopathic short stature, and primary insulin-like growth factor-I deficiency. Horm Res Paediatr. 2016;86(6):361–397.
- Zou Y, Dong Y, Meng Q, et al. Incorporation of a skeletal muscle-specific enhancer in the regulatory region of Igf1 upregulates IGF1 expression and induces skeletal muscle hypertrophy. Sci Rep. 2018;8(1):1–13.
- Gordon AM, Regnier M, Homsher E. Skeletal and cardiac muscle contractile activation: tropomyosin “Rocks and rolls. Physiology. 2001;16(2):49–55.
- Collibee SE, Bergnes G, Muci A, et al. Discovery of tirasemtiv, the first direct fast skeletal muscle troponin activator. ACS Med Chem Lett. 2018;9(4):354–358.
- Gehlert S, Bloch W, Suhr F. Ca2+-dependent regulations and signaling in skeletal muscle: from electro-mechanical coupling to adaptation. Int J Mol Sci. 2015;16(1):1066–1095.
- Gordon AM, Homsher E, Regnier M. Regulation of contraction in striated muscle. Physiol Rev. 2000;80(2):853–924.
- National Library of medicine. National Centre for Biotechnology Information TNNT1 gene [Internet]. National Centre for Biotechnology Information. 2022 [cited 2022 Apr 27]. Available from: https://www.ncbi.nlm.nih.gov/gene/7138.
- Gomes AV, Potter JD, Szczesna-Cordary D. The role of troponins in muscle contraction. IUBMB Life. 2002;54(6):323–333.
- Matyushenko AM, Shchepkin DV, Kopylova GV, et al. Unique functional properties of slow skeletal muscle tropomyosin. Biochimie. 2020;174:1–8.
- Abu-Abed M, Mal TK, Kainosho M, et al. Characterization of the ATP-binding domain of the Sarco(endo)plasmic Reticulum Ca 2+ -ATPase: probing nucleotide binding by multidimensional NMR. Biochemistry. 2002;41(4):1156–1164.
- Andrews JA, Miller TM, Vijayakumar V, et al. CK-2127107 amplifies skeletal muscle response to nerve activation in humans. Muscle Nerve. 2018;57(5):729–734.
- Russell AJ, Hartman JJ, Hinken AC, et al. Activation of fast skeletal muscle troponin as a potential therapeutic approach for treating neuromuscular diseases. Nat Med. 2012;18(3):452–455.
- ≪ Back cytokinetics announces orphan drug designation for ck-2127107 for the treatment of spinal muscular atropHY [Internet]. Cytokinetics. 2017 [cited 2022 Apr 21]. Available from: https://ir.cytokinetics.com/news-releases/news-release-details/cytokinetics-announces-orphan-drug-designation-ck-2127107.
- Ab PG. EU/3/12/970: orphan designation for the treatment of amyotrophic lateral sclerosis Share 6-Ethynyl-1-(pentan-3-yl)-1H-imidazo[4,5-b]pyrazin-2(3H)-one (tirasemtiv) [Internet]. European medical agency. 2012 [cited 2022 Apr 21]. Available from: https://www.ema.europa.eu/en/medicines/human/orphan-designations/eu312970.
- Andrews JA, Cudkowicz ME, Hardiman O, et al. VITALITY-ALS, a phase III trial of tirasemtiv, a selective fast skeletal muscle troponin activator, as a potential treatment for patients with amyotrophic lateral sclerosis: study design and baseline characteristics. Amyotroph Lateral Scler Frontotemporal Degener. 2018;19(3–4):259–266.
- de Winter JM, Gineste C, Minardi E, et al., Acute and chronic tirasemtiv treatment improves in vivo and in vitro muscle performance in actin-based nemaline myopathy mice. Hum Mol Genet. 2021;30(14):1305–1320. .
- Lee E-J, Kolb J, Hwee DT, et al. Functional characterization of the intact diaphragm in a nebulin-based nemaline myopathy (NM) model-effects of the fast skeletal muscle troponin activator tirasemtiv. Int J Mol Sci. Internet]. 2019;20. doi: 10.3390/ijms20205008.
- Lee E-J, De Winter JM, Buck D, et al. Fast skeletal muscle troponin activation increases force of mouse fast skeletal muscle and ameliorates weakness due to nebulin-deficiency. PLoS One. 2013;8(2):e55861.
- de Winter JM, Joureau B, Sequeira V, et al. Effect of levosimendan on the contractility of muscle fibers from nemaline myopathy patients with mutations in the nebulin gene. Skelet Muscle. 2015;5(1):12.
- Ochala J, Li M, Ohlsson M, et al., Defective regulation of contractile function in muscle fibres carrying an E41K beta-tropomyosin mutation. J Physiol. 2008;586(12):2993–3004. .
- Lindqvist J, Lee E-J, Karimi E, et al. Omecamtiv mecarbil lowers the contractile deficit in a mouse model of nebulin-based nemaline myopathy. PLoS One. 2019;14(11):e0224467.
- Comín-Colet J, Manito N, Segovia-Cubero J, et al. Efficacy and safety of intermittent intravenous outpatient administration of levosimendan in patients with advanced heart failure: the LION-HEART multicentre randomised trial. Eur J Heart Fail. 2018;20(7):1128–1136.
- Brixius K, Reicke S, Reuter H, et al. Effects of the Ca2+ sensitizers EMD 57033 and CGP 48506 on myocardial contractility and Ca2+ transients in human ventricular and atrial myocardium. Z Kardiol. 2002;91(4):312–318.
- Matsuba D, Terui T, O-Uchi J, et al. Protein kinase A-dependent modulation of Ca2+ sensitivity in cardiac and fast skeletal muscles after reconstitution with cardiac troponin. J Gen Physiol. 2009;133(6):571–581.
- Krebs EG. The albert lasker medical awards. Role of the cyclic AMP-dependent protein kinase in signal transduction. JAMA. 1989;262(13):1815–1818.
- Nicolau S, Kao JC, Liewluck T. Trouble at the junction: when myopathy and myasthenia overlap. Muscle Nerve. 2019;60(6):648–657.
- Natera-de Benito D, Nascimento A, Abicht A, et al. KLHL40-related nemaline myopathy with a sustained, positive response to treatment with acetylcholinesterase inhibitors. J Neurol. 2016;263(3):517–523.
- Caldas VM, Heise CO, Kouyoumdjian JA, et al. Electrophysiological study of neuromuscular junction in congenital myasthenic syndromes, congenital myopathies, and chronic progressive external ophthalmoplegia. Neuromuscul Disord. 2020;30(11):897–903.
- Rodríguez Cruz PM, Sewry C, Beeson D, et al. Congenital myopathies with secondary neuromuscular transmission defects; A case report and review of the literature. Neuromuscul Disord. 2014;24(12):1103–1110.
- Munot P, Lashley D, Jungbluth H, et al. Congenital fibre type disproportion associated with mutations in the tropomyosin 3 (TPM3) gene mimicking congenital myasthenia. Neuromuscul Disord. 2010;20(12):796–800.
- Robb SA, Sewry CA, Dowling JJ, et al. Impaired neuromuscular transmission and response to acetylcholinesterase inhibitors in centronuclear myopathies. Neuromuscul Disord. 2011;21(6):379–386.
- Sanders DB, Stålberg EV. AAEM minimonograph #25: single-fiber electromyography. Muscle Nerve. 1996;19(9):1069–1083.
- Bogni C, Girard E, Poulard K, et al. CONGENITAL MYOPATHIES–NEMALINE MYOPATHIES: EP. 40 Neuromuscular junction defects in ACTA1-related nemaline myopathy. Neuromuscul Disord. 2021;31:S61–S62.
- Blondelle J, Tallapaka K, Seto JT, et al. Cullin-3 dependent deregulation of ACTN1 represents a new pathogenic mechanism in nemaline myopathy. JCI Insight. Internet]. 2019;5(10):e125665.
- Liewluck T, Shen X-M, Milone M, et al. Endplate structure and parameters of neuromuscular transmission in sporadic centronuclear myopathy associated with myasthenia. Neuromuscul Disord. 2011;21(6):387–395.
- Nguyen M-AT, Joya JE, Kee AJ, et al. Hypertrophy and dietary tyrosine ameliorate the phenotypes of a mouse model of severe nemaline myopathy. Brain. 2011;134(12):3516–3529.
- Messineo AM, Gineste C, Sztal TE, et al. L-tyrosine supplementation does not ameliorate skeletal muscle dysfunction in zebrafish and mouse models of dominant skeletal muscle α-actin nemaline myopathy. Sci Rep. 2018;8(1):11490.
- Kalita D. A new treatment for congenital nonprogressive nemaline myopathy. J Orthomol Med. 1989;4:70–74.
- Ryan MM, Sy C, Rudge S, et al. Dietary L-tyrosine supplementation in nemaline myopathy. J Child Neurol. 2008;23(6):609–613.
- Wallgren-Pettersson C, Laing NG. 109th ENMC International Workshop: 5th workshop on nemaline myopathy. Neuromuscul Disord. Naarden, The Netherlands. 2002 11th-13th October ;13(6):501–507.
- Gimenes AC, Bravo DM, Nápolis LM, et al. Effect of L-carnitine on exercise performance in patients with mitochondrial myopathy. Braz J Med Biol Res. 2015;48(4):354–362.
- Buxmann H, Schlösser R, Schlote W, et al. Congenital nemaline myopathy due to ACTA1-gene mutation and carnitine insufficiency: a case report. Neuropediatrics. 2001;32(5):267–270.
- Hsu P-J, Wang H-D, Tseng Y-C, et al. L-Carnitine ameliorates congenital myopathy in a tropomyosin 3 de novo mutation transgenic zebrafish. J Biomed Sci. 2021;28(1):8.
- Sztal TE, McKaige EA, Williams C, et al. Testing of therapies in a novel nebulin nemaline myopathy model demonstrate a lack of efficacy. Acta Neuropathol Commun. Internet]. 2018;6. Available from. ;(1). DOI:10.1186/s40478-018-0546-9.
- Qiu B, Ruston J, Granzier H, et al. Failure to identify modifiers of NEBULIN-related nemaline myopathy in two pre-clinical models of the disease. Biol Open. 2019;8:bio044867.
- Polla B, D’Antona G, Bottinelli R, et al. Respiratory muscle fibres: specialisation and plasticity. Thorax. 2004;59(9):808–817.
- Tirrell TF, Cook MS, Carr JA, et al. Human skeletal muscle biochemical diversity. J Exp Biol. 2012;215(15):2551–2559.
- National Library of Medicine. National Centre for Biotechnology Information gene TNNT3 [Internet]. National Centre for Biotechnology Information. 2022 [cited 2022 Apr 27]. Available from: https://www.ncbi.nlm.nih.gov/gene/7140.
- Lippi G, Montagnana M, Aloe R, et al. Highly sensitive troponin immunoassays: navigating between the scylla and charybdis. Adv Clin Chem. 2012;58:1–29.
- Obradovic M, Zafirovic S, Soskic S, et al. Effects of IGF-1 on the Cardiovascular System. Curr Pharm Des. 2019;25(35):3715–3725.
- Li F, Buck D, De Winter J, et al. Nebulin deficiency in adult muscle causes sarcomere defects and muscle-type-dependent changes in trophicity: novel insights in nemaline myopathy. Hum Mol Genet. 2015;24(18):5219–5233.
- Annoussamy M, Seferian AM, Daron A, et al. Natural history of Type 2 and 3 spinal muscular atrophy: 2-year NatHis-SMA study. Ann Clin Transl Neurol. 2021;8(2):359–373.
- Annoussamy M, Lilien C, Gidaro T, et al. X-linked myotubular myopathy. Neurology. 2019;92(16):e1852–e1867.
- Fouarge E, Monseur A, Boulanger B, et al. Hierarchical Bayesian modelling of disease progression to inform clinical trial design in centronuclear myopathy. Orphanet J Rare Dis. 2021;16(1):3.
- Horton RH, Saade D, Markati T, et al. A systematic review of adeno-associated virus gene therapies in neurology: the need for consistent safety monitoring of a promising treatment. J Neurol Neurosurg Psychiatry. 2022: 329431. doi: 10.1136/jnnp-2022-329431
- Servais L, Baranello G, Scoto M, et al. Therapeutic interventions for spinal muscular atrophy: preclinical and early clinical development opportunities. Expert Opin Investig Drugs. 2021;30(5):519–527.