ABSTRACT
Introduction
In view of other candidate proteins from the cathepsin family of proteases holding great potential in being targeted during cancer therapy, the importance of Cathepsin B (CtsB) stands out as being truly exceptional. Based on its contribution to oncogenesis, its intimate connection with regulating apoptosis and modulating extracellular and intracellular functions through its secretion or compartmentalized subcellular localization, collectively highlight its complex molecular involvement with a myriad of normal and pathological regulatory processes. Despite its complex functional nature, CtsB is emerging as one of the few cathepsin proteases that has been extensively researched to yield tangible outcomes for cancer therapy.
Areas covered
In this article, we review the scientific literature that has justified or shaped the importance of CtsB expression in cancer progression, from the perspective of highlighting a paradigm that is rapidly changing from basic research toward a broader clinical and translational context.
Expert opinion
In doing so, we detail its maturation as a diagnostic marker through describing the development of CtsB-specific Activity-Based Probes, the rapid evolution of these toward a new generation of Prodrugs, and the evaluation of these in model systems for their therapeutic potential as anti-cancer agents in the clinic.
1. Introduction
The established regulation of the cathepsin (Cts) proteases in molecular cell biology is an area of research that has flourished over the recent 15 years. Whilst such proteases have always been recognized for their central role in lysosomal function, more recently, their effects outside of the cell, as architects of Extracellular Matrix (ECM) remodeling, have also become established. When coupled with some of their emerging intracellular functions, and the regulatory inter-relationships between these sub-cellular compartments, their collective biological effects and their amenability for therapeutic targeting are coming into focus in a number of disease pathologies, such as cancer [Citation1,Citation2].
The family of Cts proteases is composed of 15 members and which can be broadly sub-divided up into cysteine (B, C, F, H, K, L, O, S, V, Z/X, W), aspartic (D, E), or serine (A, G) proteases, from which some cysteine proteases have been reported to possess exopeptidase (B, H, Z/X, C) or endopeptidase (B, S, K, V, F, L) activities (reviewed in [Citation3] and [Citation4]). While normally resident in the lysosomes, the cathepsins can be secreted or leaked out from these under conditions of cathepsin overexpression, a drop in pH or upon alterations in the oxidation state of cells [Citation5–8]. Moreover, they can also be expressed as protein-isoforms that can localize to the mitochondria or nucleus and regulate cellular proliferation and fate (reviewed in [Citation9]). When taken with the enzymic activation of cysteine cathepsins being enhanced in a low pH-dependent manner, as with Cts-B, -K, and -L in vitro [Citation8,Citation10], these factors collectively highlight the importance of a number of key regulatory cues that dictate the spatio-temporal expression of cathepsins, and can originate from the metabolic programming of the host cells in which they reside [Citation8].
When viewed from a broader perspective, the deduction that most of the cathepsins have been described as being good therapeutic target proteins, particularly in the field of cancer research, is therefore not surprising. This viewpoint has been largely shaped by them being overexpressed or secreted from a number of cancer cell types, macrophages, and fibroblasts, as seen (for example) during gastric cancer development (reviewed in [Citation11]). Here, one other significant property being their enhanced proteolytic activation under relatively low pH conditions, which is synonymous with the hypoxic conditions that some tumors can thrive in [Citation6]. In this context, cysteine Cts-B, -L, -D, and -S justify their candidacy as good therapeutic targets as some of these have been reportedly overexpressed and secreted [Citation6] from a broad spectrum of cancers (reviewed in [Citation3]) and exhibit enhanced intracellular protease activity against substrate proteins from the pro-apoptotic pathway such as BID [Citation12,Citation13] under relatively low pH conditions [Citation8,Citation14,Citation15].
Consequently, some of the most promising anti-cathepsin targeting agents currently emerging have diversely taken the form of small-molecule inhibitors (SMIs) or peptide therapeutics, and both of which have demonstrated their effectivity and potential through their development as Activity-Based Probes (ABPs) for clinical diagnostic-testing purposes [Citation16–18]. Importantly, such diagnostic probes have also topically evolved toward the generation of Photo-Sensitization-ABPs (PS-ABPs) and Prodrugs, either of which can be activated upon the cleavage of a CtsB-specific target sequence that liberates a diagnostic or therapeutic agent [Citation19–21]. Such elaborate targeting strategies have been successfully conceived from structure-based findings, which are founded on the rationale that cathepsins are normally susceptible to inhibition, as seen between some cystatin inhibitor proteins and Cts family members, such as CtsB [Citation22–24]. Consequently, through the diligent extension of such studies, a prolific area of research with a strong clinical trajectory has developed, which is coming to fruition through the development and testing of novel cathepsin protease-targeting therapeutic agents in model systems.
Herein, we focus on the relatively well-characterized CtsB protein, through highlighting its intracellular, extracellular, and functional properties that are central to cancer progression. We contextualize the clinical significance of such research findings and describe recent progress focused on how such findings have been adapted and harnessed for ABP-based diagnostic assay development and then extended for anti-cancer Prodrug therapeutic design.
1.1. Cathepsin B-isoform protein expression and cancer
Unlike most of the cathepsin proteases that have restricted tissue expression, Cts-C, -B, -H, -K, -O, -L, and -Z/X are expressed with relative broadness and which have remained at the forefront of research through unveiling and defining general mechanisms for cathepsin protein expression [Citation25], activation, and substrate cleavage during normal cellular homeostasis or disease progression [Citation26]. From such studies, CtsB has emerged with heightened importance due to its enhanced genomic expression and function in tumor metastasis [Citation26,Citation27] during advanced gastric or breast cancer (BC) [Citation28,Citation29], and in patients with enhanced inter-pulmonary metastases [Citation30].
Under normal conditions, CtsB mRNA is ubiquitously expressed in a diverse range of tissues and highly expressed in liver, kidney, thyroid gland, and spleen as a protein of 339 aa from chromosome 8.p23.1 [Citation25,Citation31–33], and which can be up-regulated by IL-6 [Citation34] during inflammation, and by transcription factor activation as seen with SP1 [Citation35], USF [Citation36] and possibly p53 [Citation37,Citation38], either directly or indirectly upon p53-responsive miRNA expression (reviewed in [Citation39,Citation40]). More recently, CtsB expression was reportedly enhanced by transcription factor MZF1 in BC cells [Citation41] and the secretion of which is also enhanced during melanoma invasiveness in an Ets1-, SP1-, NF-kB- transcription factor-dependent manner [Citation42]. Consequently, its overexpression in disease is believed to contribute to the progression of hepatocarcinoma [Citation43], colorectal cancer [Citation44], esophageal cancer [Citation45], BC [Citation38,Citation46], lung cancer [Citation47], squamous cell carcinoma [Citation48], liver metastases [Citation49], gastric cancer [Citation50], glioblastoma [Citation51], and even osteoarthritis [Citation52], as confirmed by a number of CtsB-directed expression, antisense [Citation53], siRNA, and shRNA studies [Citation54]. However, robust CtsB expression may not strictly correlate with the classical stage-specific manner of cancer development, and may be a feature related to cancer type. This is largely based on a number of recent studies (reviewed in [Citation55]), where a significant number of patient colonic tumors expressed CtsB irrespective of their stage of development, whereas the remainder tested negative for CtsB expression [Citation56]. Conversely, another study addressing CtsB expression-trends in colorectal cancer reported that the expression of this protease contributed to colorectal cancer development and invasiveness, and reported enhanced expression of CtsB much earlier during cancer development, which declined during the later stages [Citation57,Citation58].
With these observations in mind, it is worth highlighting that most of these studies have been aimed at reporting the expression levels of full-length CtsB [Citation59]. However, based on a number of in-silico and experimental sources (reviewed in [Citation9]), full-length CtsB can also be co-expressed as a number of protein isoforms [Citation60–63], which lack the secretory signal (CB-2) or the secretory signal and part of the cathepsin pro-domain (CB2-3), as a result of the alternative splicing of the CtsB transcript. Importantly, expression profiling studies have linked CB-2 [Citation61,Citation64] (reviewed in [Citation9]) and CB2-3 overexpression to kidney, liver, colorectal cancers and alternatively to the progression of osteoarthritis [Citation65], thus highlighting their potential involvement in a diverse number of diseases and their growing exploitation as diagnostic markers. Furthering such elegant studies to also incorporate other cathepsin protease isoform members does offer greater potential in defining novel biomarkers, whilst also unveiling their mechanistic involvement in established disease-specific regulatory paradigms. This may be of great significance, particularly as Cts-C, -E, -L, -M, -S, and -W are also expressed as potential isoform proteins (reviewed in [Citation9]).
In summary, enhanced CtsB isoform expression is linked to the development, and progression of a number of cancer types and the key signaling pathways or transcription factors responsible for this are indeed being identified and functionally dissected. However, the intermolecular-regulatory relationships at the compartmentalization level (), and the precise biological function of each CtsB isoform protein, during cancer development or progression does remain to be explored more fully [Citation66].
Figure 1. The protein expression, trafficking, and targeted detection of cathepsin B (CtsB). Transcribed CtsB is translated and enters the secretory pathway and upon glycosylation resides in perinuclear lysosomes. Under specific cell stimulatory conditions or upon overexpression, CtsB can translocate to peripheral lysosomes and is secreted, where it can modulate extracellular matrix dynamics. Alternatively, CtsB can leak from lysosomes, process apoptotic proteins such as Bid and BAX which contribute to mitochondrial outer membrane permeabilization (MOMP) and apoptosis. CtsB can also reside in the nucleus of some cells as a result of it leaking from lysosomes as a mature protease, or after being expressed as a non-secreted CtsB isoform protein. Quench fluorescence Activity-Based probes (qABP), Photo-Sensitized ABPs (PS-ABP), or Prodrugs have been designed and tested to detect or target compartmentalized mature CtsB within the Extracellular Matrix (ECM) or within the intracellular endo-lysosomal compartments.
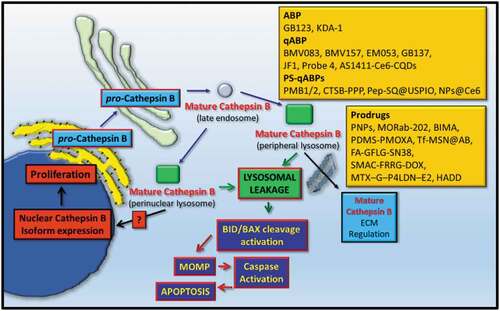
1.2. Cathepsin B subcellular localization and function
As touched upon, the expression of cathepsin proteases can alter the fate of cells and how they might respond to natural extracellular signaling cues, under normal conditions or during disease progression. Based on this line of thought, a number of interesting avenues of research have developed over the recent years, which have allowed a fuller picture to develop with regard to how cathepsins can modulate cancer progression based on their subcellular and extracellular localization (reviewed in [Citation3,Citation9]). Here, the main avenues of research have revolved around defining how the lysosomes lose cathepsin proteases to the cytoplasm (or nucleus) and how cathepsin protease secretion can be enhanced from the lysosome into the extracellular milieu ().
Under normal conditions, the cathepsins come to reside in the perinuclear lysosomes following their passage through the late endosomes, where a drop in pH permits their intermolecular activation and pro-domain removal as with CtsB [Citation67], and which can also be mediated by CtsG [Citation68]. However, under certain disease pathologies, as in malignant BC progression [Citation69], CtsB comes to reside in peripheral lysosomes and in exocytic vesicles [Citation5] as a location believed to contribute to enhanced pro- and active-CtsB secretion. Here, one physiological trigger for this includes extracellular acidification and which can be simulated by exposing cells to conditioned media, as seen for BC cells [Citation5,Citation6]. Mechanistically, the expression of USP17 can also enhance Cts secretion, as reported for lysosomal trafficking of CtsD to the cell periphery in response to EGF signals, and offers clues about whether this process also modules CtsB protein trafficking [Citation70].
To complement such findings, Lysosomal Membrane Permeabilization (LMP) or Lysosomal Leakage (LL) is additional mechanisms by which the cathepsin proteases can exit lysosomes and enter the cytoplasm, where they can exhibit unique biological effects based on their nuclear and mitochondrial localization, for example. As an alternative mechanism, this may arise through the generation of a novel mitochondrial localization signal as a result of alternative transcriptional splicing events, and as reported for CtsB [Citation71] (reviewed in [Citation9]). In the context of cancer cells, the lysosomes are reported to be abnormal, larger and more fragile than normal, thus elevating their sensitivity to agents and mechanisms that can induce LMP through cathepsin overexpression and LAMP1/2 cleavage [Citation2,Citation72], reactive oxygen species [Citation73], metal ions [Citation74], inflammatory cytokine signals [Citation75], inhibition of sphingokinase-1 or S1P loss [Citation76,Citation77] and by lysosomotropic agents [Citation78], such as Siramesine [Citation79,Citation80], Riccardin D (RD-N [Citation81]), Nortriptyline [Citation82], or carbon black nanoparticles [Citation83]. Importantly, such agents have the potential for enhancing cathepsin-mediated cell death, as reported for CtsB nuclear relocalization, after RD-N mediated LMP induction, and by elevating DNA damage and suppressing BRCA1 activity [Citation81,Citation84]. Similarly, LL-derived CtsB has also been associated with nuclear sirtuin 1 degradation during the aging process of the mouse brain, giving rise to impairments in cognitive function [Citation85]. Alternatively, the loss in expression of CtsB can reduce proliferation and elevated IL-17A/SAA-induced HaCat keratinocyte differentiation [Citation86]. Such reports collectively support a central role for CtsB expression in cellular growth and differentiation in response to extracellular signals.
More recently, a growing biological feature of Cts proteases is their localization in the nucleus, as reported for Cts-D [Citation87], -B [Citation81], -H [Citation88], -L [Citation89–91], and more recently -S [Citation92] (reviewed in [Citation9,Citation12]). Among these nuclear cathepsins, CtsB has been reported to cleave BRCA1, in prostate cancer cells with the effect of sensitizing cells to the effects of Cisplatin [Citation81]. Through alternative transcriptional splicing, cytoplasmic CtsB expressed in the absence of its amino-terminal secretory signal (or CtsB derived from cellular LMP) has been reported to reside in the nucleus in proliferating thyroid cancer cells, where it can cleave histone protein H1 [Citation93]. Unlike other CtsB isoform proteins, which can also localize to the mitochondria [Citation94] and induce mitochondrial dysfunction [Citation95], these nuclear-resident CtsB molecules arrive at the nucleus in the absence of them encoding a bona fide nuclear-localization signal [Citation93]. Conceivably, leaked CtsB can be chaperoned into the nucleus, and the mechanism for which needs further elucidation, based on it potentially being harnessed for therapeutic targeting purposes, especially during chemoresistance (). Such a perspective is largely shaped by studies demonstrating the nuclear relocalization of Cts-L and -D, by their respective chaperones, the CUX1/Snail protein complex [Citation90,Citation96] and the protein BAT3 [Citation87], which can induce the proliferation or trans-differentiation of cells.
In summary, a number of potential underlying mechanisms and effects have been reported for the existence of nuclear cathepsin proteases, which could offer additional research avenues that can be exploited for compartment-specific therapeutic targeting and diagnostic strategies.
1.3. Cathepsin B regulation and substrate specificity
Broadly speaking, CtsB-specific substrates can be classified into two groups based on the localization of the protease. Whilst considerable focus has been directed at the effects of protease-directed extracellular remodeling during tumoral expansion, intracellular cathepsin expression can also contribute to this by cleaving a number of cancer-related substrate proteins, which modulate cellular proliferation and resistance to apoptosis (reviewed in [Citation12]). This may have particular relevance during the acidification of cells or their microenvironment, the latter of which may arise due to metabolic reprogramming of cancer cells [Citation97,Citation98], and which can collectively give rise to enhanced cathepsin expression, secretion [Citation7], and subcellular relocalization [Citation6].
Extracellularly, CtsB has been seen to act on modulating the architecture of the ECM through the cleavage of E-cadherin (as reported for human pancreatic cells [Citation99]) and other ECM components, such as Fibronectin, Collagen IV [Citation100], and Tenascin [Citation101], in bovine lens capsules, astrocytomas, or glioblastoma cells, and during tumor development, invasiveness, and progression. Here, the regulation of angiogenesis or tumor vascularization by cathepsin proteases has also gained considerable attention through their potential to be targeted as part of emerging therapeutic strategies. In this context, Cts-S and -L-mediated cleavage of COLXVIII to yield the anti-angiogenic factor endostatin has taken on a degree of importance during cancer progression and development [Citation102]. Additionally, CtsB expression can promote tumor angiogenesis [Citation103] through CD18 shedding from leukocytes [Citation104,Citation105] or negatively regulate angiogenesis, through inhibiting VEGF-A expression [Citation106]. As an area that requires further exploration, such reports collectively support the ever-growing connection between the cathepsins and cytokine (or receptor protein) shedding, and how such events modulate the ECM to favor tumoral expansion and its metastatic spread [Citation107].
Intracellularly, the importance of lysosomal factors in regulating cell fate emerged from the observations that lysosomal rupture induced cell death, hence this organelle being colloquially named the ‘suicide bag’ [Citation108,Citation109]. At the molecular level, Cts-B and -D were seen to cleave and activate BID [Citation110], following the leakage of these proteases form the lysosome prior to caspase-8 activation [Citation13,Citation111–113] . As this is a mechanism that requires the partial cleavage of BID to active ‘t-BID,’ it is likely to occur through a stringently regulated mechanism, which permits BID cleavage in the absence of complete BID degradation [Citation13,Citation114,Citation115]. One such mechanism could be the pH-dependent activation of CtsB. Here, at a normal intracellular pH, the cysteine cathepsins are relatively less active, which could account for why the partial cleavage of BID is permitted, and give rise to cellular apoptosis through the intrinsic pathway upon LMP induction [Citation13]. In support of these findings, BID cleavage has been mapped to Arg-65 or Arg-71, through the activity of Cts-B, -H, -L, -S, and -K, which occurs close to the preferred cleavage site of caspase-8 [Citation110]. Conversely, under lower pH conditions, LMP-derived or secreted cathepsins can exhibit enhanced activity, giving rise to the complete degradation of apoptosis-regulatory proteins, and certain extracellular ECM components [Citation6]. Here, Droga-Mazovec et al. (2008) reported the positive cleavage of BAK (along with Bcl-2, Bcl-xL, and Mcl-1 proteins) at pH 7.2 by Cts-B, -L, -S, and -K in vitro [Citation116] and Soond et al. (2021) reported the cleavage of the BAX protein, by CtsS at pH 7 and 5 [Citation92]. Whilst the cleavage of such protein substrates may alter cellular fate, the effects of CtsB and its lysosomal cleavage of the cell cycle inhibitor p27KIP are also worth mentioning as a contributing factor to tumor progression, in light of elevated cyclin B1 levels being reported in CtsB-deficient tumors, thus linking positive CtsB expression with cellular proliferation [Citation55].
Collectively, such findings highlight a pH-dependent mechanism for CtsB release, activation, and the cleavage of substrates that are central to determining cell fate and growth. Moreover, such fluctuations in pH may also have the likelihood of altering CtsB-directed substrate and therapeutic specificity [Citation117].
1.4. Cystatin-mediated inhibitory regulation of cathepsin B
Clearly, the emerging pathological significance of cathepsin proteases over the years has been established on their overexpression in certain disease states, leakage from the lysosomal compartment, and their subsequent ability to cleave key protein intermediates central to determining cell fate [Citation3]. Based on the evidence derived from subcellular compartmentalization of cathepsin proteases and substrate specificity, another key regulatory step connected with regulating the cathepsins originates from the actions of the reversible cystatin inhibitor proteins [Citation22,Citation118,Citation119].
As a superfamily of proteins, the cystatins can be subclassed into clans, families, and Type 1–3 subfamilies. Here, the Type 1-cystatins (∼100 aa, or stefins) are produced intracellularly [Citation120] and can also be secreted [Citation121]. Type 2-cystatins (∼115 aa) are exclusively secreted [Citation122], whereas the Type 3-cystatins are secreted into the blood and are of varying molecular weights and are also referred to as Kininogens [Citation118]. The combined data from a number of excellent structural studies has yielded valuable information about how cystatins modulate the cathepsins, whilst others have described how the cathepsin proteases are regulated by their inhibitory pro-domains [Citation23]. When combined, both schools of thought have unveiled a number of robust regulatory mechanisms, which highlighted the susceptibility of cathepsins to therapeutic targeting [Citation26].
Generally speaking, the cystatin inhibitors, which are reversibly bound to the active cathepsin proteins, can be displaced upon the recognition of substrates by the cathepsin protease [Citation26], as seen with stefin A displacement from CtsB [Citation24]. Here, CtsB is viewed as being unique in the sense that it encodes a ∼20 aa occluding loop motif [Citation123–128], from which residues H110 and R116 bind the main body of the enzyme through residues D22 and D224, an interaction that restricts access to the active site cleft and offers CtsB carboxypeptidase activity through residues H110 and H111 residues docking with the carboxyl group of the substrate. Mutation analysis of these key residues have been reported to enhance CtsB endopeptidase activity [Citation129]. The flexibility of the occlusion loop also offers regulatory function as deletion of aa 109–118 can abrogate CtsB exopeptidase activity, and yields a protease harboring endopeptidase activity alone [Citation130]. Collectively, these observations support a mechanism that involves occluding loop displacement from the active site cleft by the incoming substrate, thus allowing CtsB to act as an endopeptidase. Similarly, an alternative CtsB inhibitory step has also been suggested, which involves the insertion of the CtsB pro-domain into the active site cleft upon maintaining the inactive zymogenic form of CtsB [Citation23,Citation131,Citation132]. Importantly, this has been suggested to occur in competition with the occlusion loop, which competes more effectively at a lower pH [Citation133]. Collectively, full activation of CtsB through unveiling its active site has been proposed to occur upon the cleavage of the intermolecular pro-domain by other cathepsins during secretion [Citation131], upon a drop in intracellular pH [Citation133], exposure to glycosaminoglycans, or upon reaching the cell surface under decreased pH conditions and in a V-ATPase-dependent manner [Citation5,Citation7,Citation68,Citation134,Citation135].
1.5. Cathepsin B is a cancer-specific diagnostic marker
Based on observations that CtsB overexpression can be correlated with cancer progression, such reports have paved the way for accurately quantifying CtsB levels for tumor identification, and cancer diagnostic or prognostic assay development purposes. Here, CtsB has been reported to be overexpressed in a diverse number of cancer types of the pancreas [Citation136], lung [Citation30], breast [Citation137], colon [Citation138], glioblastoma [Citation51], hepatocarcinoma [Citation66,Citation139], squamous cell carcinoma [Citation48], gliomas [Citation140], head and neck cutaneous squamous cell carcinoma (mHNcSCC [Citation141]), pediatric AML [Citation142], and malignant melanoma [Citation143]. Whilst such observations do highlight the potential for CtsB to be promisingly earmarked as a potential diagnostic marker, the robustness and accuracy of this test has seen developments through the co-detection of CtsB alongside other biomarkers of interest. For example, CtsB and CtsD transcripts and proteins were simultaneously detected in squamous cell carcinoma cells [Citation48], and liver metastases from colon adenocarcinomas [Citation49].
In a similar context, the co-detection of CtsB alongside cystatin proteins has also been exploited for their diagnostic potential and helped to validate cathepsin expression levels as reliable diagnostic markers. For example, CtsB and cystatin C ratios were quantified from colorectal cancer patients [Citation44] or sera from esophageal cancer patients [Citation144], and ratio levels found to be significantly higher in comparison to normal. Similar findings in support of CtsB levels being significantly higher than stefins A and B in hepatocellular carcinoma (HCC) tissues were also reported by Lin et al. (2016), supporting a correlation of CtsB expression and HCC progression whilst establishing the use of CtsB and stefins A and B as good diagnostic markers [Citation43]. In a more recent study, elevated CtsB and stefin A levels were reported for renal clear cell carcinoma tissue samples, thus validating the cancer-specific nature of such correlation-based testing regimens [Citation145].
Collectively, these diagnostic approaches do offer significant degrees of accuracy in adopting the use of CtsB (±cystatins or stefins) as diagnostic assays in a variety of cancer types. Whilst limitations in accuracy may arise from the abilities of cathepsins to degrade cystatin proteins, and for cathepsins (or cystatins) to be sub-compartmentalized in the cytoplasm and the nucleus (to varying degrees), an alternative repertoire of emerging Activity-Based Probe (ABP)-tests has been developed. Such rapidly emerging approaches are permitting the limitations arising from adopting the above classical approaches to be addressed, with greater speed and accuracy.
2. Cathepsin B can be detected and therapeutically targeted with ABP, qABP, PS-ABP, and fluorescent prodrug technology
Derived from co-crystallization structural studies of CtsB and stefin A [Citation24] and through targeting CtsB catalytic activity by the screening of peptide libraries and peptide cleavage studies, a number of useful diagnostic and therapeutic peptides or small-molecule inhibitors (SMIs) have been arrived at [Citation146,Citation147] . Importantly, due to the highly conserved structural nature of the cathepsin active sites, such approaches have successfully yielded or validated candidate therapeutics that can specifically distinguish between Cts-B, -D, -H, and -L, using site directed mutagenesis of the protease active- or substrate cleavage-sites [Citation148,Citation149].
Consequently, in a shift from the classical ELISA, RT-PCR, or IHC paradigms for detecting CtsB protease expression, emerging assays have incorporated the use of irreversible fluorescently labeled Activity-Based Probes (ABP) or substrates, with some very encouraging outcomes. Simply described, these are composed of a fluorescently labeled ‘warhead’ conjugate molecule, which can form a covalent bond with the active-site thiol group of the cysteine protease [Citation150], thus allowing its deactivation and detection (). As a good starting point, small-molecule probes linked to the warhead and fluorescent conjugates specific to cathepsins did have the outcome of validating a ‘proof of principle’ in being able to detect proteases using optical fluorescent imaging techniques such as Fluorescence Tomography (FT), Positron Emissions Tomography (PET), or Near InfraRed Fluorescence (NIRF). Such approaches have been developed and superseded by ABP substrates (), which offer greater sensitivity and specificity, seeing as the human genome encodes over 500 proteases [Citation151]. Such substrates were demonstrated to target CtsB by the protease recognizing a CtsB active site-specific peptide sequence, and which have also been referred to as ‘smart probes,’ particularly in the context of qABP (quench fluorescence-ABP) substrates (). However, in the absence of an active-group thiol-targeting warhead, such substrates do not inhibit CtsB and leave the targeted protease active [Citation16]. Nevertheless, such approaches have yielded successes, through the development of PS-qABP (photosensitized-qABP), which rely on the interplay between their three non-toxic components comprising two fluorophores linked through a cathepsin cleavage site. Following the cleavage of the agent, illumination at a particular wavelength of light can give rise to the generation of cytotoxic reactive oxygen species (ROS) from endogenous oxygen. Similarly, the advantages of targeting the cathepsins in such a manner also permit the delivery of Prodrugs to the site of the tumor and the cleavage products of which can also be fluorescently detected using FT/NIRF [Citation21]. Generally, PS-qABPs and Prodrugs can be generated through self-assembly or can be coupled to a carrier, which can enhance uptake of the Prodrug or release of the active drug (). Such a technology has been evaluated for efficacy in cell line and xenograft models, some of which are showing some very encouraging outcomes.
Figure 2. Utilization of Cathepsin B (CtsB) detection or activity in cancer diagnostic assays or therapy. Activated-Based Probes (ABP), designed with a warhead moiety (read arrowhead), a CtsB recognition/cleavage sequence (such as GFLG, white box), and a fluorescent excitable probe (green circle) allow CtsB detection. The fluorescent tag can also be quenched by an accompanying chemical moiety, as in qABP substrates (pale green circle). PS-qABPs are composed of an excitation-detectable fluorescent tag (green circle) and a specific chemical intermediate (yellow circle), which inflicts cytotoxic effects can be released upon photosensitization. Fluorescent Prodrugs contain a CtsB cleavage site, which determines the release of an active drug (yellow circle) and a fluorescent tag for detection purposes (green circle).
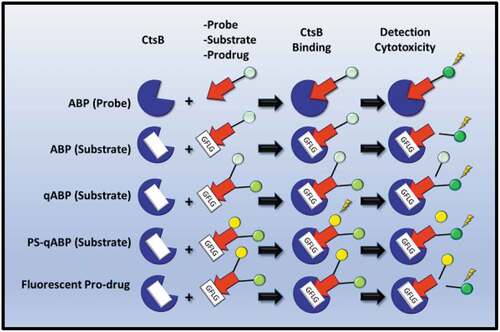
2.1. Detecting CtsB expression with ABPs
As described in for ABP Probes, visualization of GB123 in mice bearing human MDA MB-231 breast cancer tumors, followed by standardization of fluorescence against tumor weight [Citation152], saw the accumulation of GB123 at 12 hr post-injection (PI). In comparison to a commercially available substrate (ProSense 750), GB123 was shown to exhibit 10–12× detected signal strength at 24 hr (PI). At the tested dose (and as a potential drawback), GB123 was observed to only label a subpopulation of cathepsins, leaving most active enzymes intact and uninhibited. In C2C12-derived mouse tumors, which express higher cathepsin protease activities [Citation16], similar signals were obtained for GB138 in comparison to the commercial control, ProSense 680. In support of these encouraging findings, the specificity of GB138 was positively confirmed using gel-labeling experiments to detect Cts protease activity from GB138 treated tumor-derived whole-cell lysates. Analysis of tumors ex vivo showed a high uptake, whereas a low specific uptake was recorded in all organs except the lungs.
Table 1. Activity-Based Probes (ABPs) specific for detecting cathepsin proteases are highlighted. ABPs were tested for specific cathepsin protease detection using Gel-Labeling assays, cellular imaging approaches or in whole animal systems. The diseases that the ABPs have been tested for are also highlighted. NA-Not Applicable; Y-Yes; Mac-Macrophages; OA-Osteoarthritis; BC-Breast Cancer; K-Kidney; M-Mouse; AS-Atherosclerosis; KidC-Kidney Cancer.
The detection-potential of such agents has also been extended to other disease models such as atherosclerosis, where high levels of Cts-B and -S have been reported for macrophages derived from carotid plaques [Citation153]. Using Fluorescent Molecular Tomography, GB123 could detect atherosclerotic (AS) plaques in mice and was confirmed by ex vivo analysis of tissues and macrophage labeling experiments. Similarly, Cts-B and -S were detected in chondrocyte cells and human Osteoarthritis (OA) tissue samples, and late OA sera samples [Citation154]. Finally, taking a more cathepsin-specific approach, Bhuiyan et al. (2021) developed KD-1 (and cell-permeable derivative, KD-1-OMe) as CtsB-specific ABP probes, which inhibited the proliferation of highly metastatic MDA-MB-231 breast cancer cells and also detected CtsB in HEK293 cells [Citation155].
Collectively, such findings describe the development and partial evolution of ABP probes through the GB123 paradigm, and the application for CtsB detection in numerous disease and cell types, whilst emphasizing the power of such probes based on their cellular (and tissue) uptake, relative signal intensity, and cathepsin specificity. Moreover, such a technology has the demonstrated potential for detecting CtsB from serum samples in a rapid testing assay, whilst simultaneously demonstrating the inhibitory effects of some of these probes, as in the instance of KDA-1 [Citation155].
2.2. Detecting Cts B expression with qABP substrates
Moving forward, the approaches defined by the development of ABP probes yielded the generation of qABP probes and substrates [Citation19]. For the latter, detection of specific cathepsins based on their ability to cleave a specific peptide connecting two parts composed of a fluorescent tag and its quenching moiety, was permitted.
For example, Bender et al. (2015) developed BMV083 and BMV157, the latter of which gave optimized CtsS specificity upon altering the quencher group to sulfo-QSY21 [Citation156]. BMV157 (like EM503) could be used to visualize cathepsins in living cells and organisms, with the notable difference that EM503 had a broader range and better quenching abilities based on it using BODIPY-FL and the quencher BHQ-10. Whilst EM053 was not tested in mouse models for its effects on bone marrow-derived (BMD) cells and cancer proliferation, it could efficiently detect CtsS in living cells [Citation156]. In support of such findings, through adopting similar experimental approaches, GB137 was demonstrated to successfully detect Cts-S and -B from macrophages and carotid plaques in atherosclerosis mouse models, whilst also demonstrating general applicability of such an assay for diagnostic purposes in diseases other than cancer [Citation153]. Another promising example of a reagent with negligible toxicity was reported by Sun et al. (2019) for reagent JF1, which could be taken up by CtsB-expressing MCF7 cells. Importantly, JF1 could be used to visualize BC tumors, 10 minutes PI, demonstrating the rapid screening potential of this assay. Importantly, histological analysis of major organs showed zero toxicity following 1 week of treatment [Citation157]. Finally, Probe 4 [Citation158] and AS1411-Ce6-CQDs [Citation159] could penetrate prostate, breast, cervical, and kidney cancer cells and successfully detect intracellular CtsB.
2.3. Harnessing CtsB expression for targeting cancer cells using PS-qABPs
As a significant and progressive development, PS-qABP and PDT have been utilized to target cell types over-expressing specific cathepsins using real-time imaging in living cells and in whole animals [Citation20].
As highlighted in , CtsB-cleaved PMB1/PMB2 releases phthalocyanine units, which become cytotoxic upon their irradiation with light at a wavelength of greater than 610 nm and the effects of which were sensitive to the cathepsin inhibitor CA-074Me [Citation160]. Prior to cathepsin-mediated cleavage, these PS-ABPs showed little background levels of fluorescence, and were readily taken up by cells [Citation160]. Alternatively, with CTSB-PPP, no dark toxicity or ex vivo vascular function was observed with concentrations of 6 µM and was taken up by live BMC cells quite readily after 60 minutes [Citation20]. Dose-dependent cytotoxicity and concomitant increases in the production of reactive-oxygen species (ROS) were observed with treated BMCs and upon their illumination with light sources of 671 nm. Similar trends and observations were also reported for Pep-SQ@USPIO using TNBC cells in mice bearing BC tumors using MRI/NIRF imaging. Here, following laser irradiation of Pep-SQ@USPIO, significant Tumor Inhibition Rates (TIRs), low Ki-67 expression, and high apoptosis rates were reported [Citation161]. Finally, NPs@Ce6 approached the use of such technology through innovatively linking photosensitizers to a CtsB-Paclitaxel Prodrug. This approach utilized a two-stage process that involved photochemical internalization and then chemo-photodynamic therapy. Here, therapeutic uptake was rapid, showed greater than 98% inhibition of human bladder cancer growth, and using bioinformatic approaches the authors demonstrated cell cycle inhibition, increased ROS production, and reduced cell metastasis and invasiveness [Citation162].
Table 2. Quenched Activity-Based Probes (qABPs) specific for detecting cathepsin proteases are highlighted. qABPs were tested for specific cathepsin protease detection using Gel-Labeling assays, cellular imaging approaches or in whole animal systems. The diseases that the qABPs have been tested for are also highlighted. NA-Not Applicable; Y-Yes; BMM-Bone Marrow Macrophages; Mac-Macrophages; M-Mouse; BC-Breast Cancer; AS-Atherosclerosis; PrC-Prostate Cancer; CerC-Cervical Cancer; KidC-Kidney Cancer.
Table 3. Photosensitization quenched Activity-Based Probes (PS-qABPs) carrying specific cleavage sequences for Cathepsin B (CtsB) are highlighted. PS-qABPs were tested for specific CtsB detection in cells in vitro, and in vivo using whole animal systems. IC50 concentrations are highlighted as are their abilities to inhibit tumor proliferation. The diseases that the qABPs have been tested for are also highlighted. NA-Not-Applicable; M-Mouse; H-Human; Lun-Lung; Liv-Liver; PTX-Paclitaxel; TNBC-Triple-Negative Breast Cancer; BladC-Bladder Cancer; Xeno-Xenografts.
Collectively, such assays highlight the viability of PS-qABP as a substrate for the detection and treatment of tumors, and which may also show great potential in treating cancer-derived metastases or chemo-resistant cells.
2.4. Harnessing CtsB expression for targeting cancer cells with Prodrugs
To date, excellent studies (and articles) have been published showing the development of anti-cathepsin therapeutics, as in the case of ‘DARPin’ technology-against non-active site directed CtsB detection in mouse BC models in vivo [Citation163], DOFA-which acts by targeting the occluding loop, thus obstructing substrate-active site access [Citation164], CA-074Me-which acts by blocking the substrate-binding site of CtsB and reported as far back as 1991 [Citation165], SMIs designed against CtsB using structure-based docking studies [Citation166] and the incorporation of cathepsin-derived cleavable target sequences within Prodrugs for CtsB-targeted release, and areas that have all developed over the last 3 years.
As seen from , there has been consistent progress in the latter of these areas, through developing drugs such as pro-Doxorubicin or Eribulin in forms that self-assemble or can be loaded onto nano-carriers. Here, nano-carriers were viewed to help improve localized Prodrug delivery, release, and inhibitory rates in cell lines or mouse xenograft models. As reported, Doxorubicin was reported to have shown the greatest IC50 effect at 9.11 µM and 0.86 µM against ovarian cancer cells [Citation167] and fibrosarcoma cells [Citation168], respectively. In xenograft mouse models, 90% tumor inhibition was reported for lung and liver cancers [Citation169] and up to 83.1% inhibition of colorectal cancers [Citation170]. From these findings, it is interesting to note the effects attributed to the choice of delivery nano-carrier, particularly in relation to the utilization of gold nanorods that gave up to 80% inhibition against lung and breast cancer development [Citation171]. In this context, these differences could have arisen due to nano-particle toxicity, non-specific or rapid (and inconsistent) release of therapeutics, and possible mass-production/scale-up inconsistencies during the nano-carrier preparations.
Table 4. Selected Prodrugs are highlighted along with specific cleavage sequences for Cathepsin B (CtsB). PS-qABPs drugs were tested for their inhibitory effects in vitro cellular systems and in whole-animal systems. DOX-Doxorubicin; MIS-Minimal Inhibitory Sequence; M-Mouse; NA-Not-Applicable; BC-Breast Cancer; FibS-Fibrosarcoma; TNBC-Triple-Negative Breast Cancer; ColC-Colon cancer; Card-Cardiomyocytes; PanC-Pancreatic Cancer; PrC-Prostate Cancer; LuC-Lung Cancer; Pan-Pancreatic Epithelial; Ova-Ovarian Cancer; CerC-Cervical Cancer; GasC-Gastrointestinal Cancer; Endo-Endothelial; LivC-Liver Cancer; NSCLC-Non-Small Cell Lung Cancer.
Using a novel approach, some Prodrugs showed greater effectiveness when administered in a combined therapeutic regimen (in models systems), as self-assembling therapeutics, which can (for example) help address issues associated with low targeting efficiency. In this context, the efficacy of PNP-Dox administered with Navitoclax showed an increase in BC tumor inhibition to 76.97% in comparison to PNP-Dox alone (47.76% inhibition) [Citation183]. Similarly, Eribulin/MORAb-202 [Citation184] was effective at an IC50 at 20 pM in a number of cancer cell lines and was seen to decrease further to 0.1 × 10−10 M, when co-administered with Farletuzumab against BC cells [Citation185]. Other combined therapeutic regimens included the co-treatment of Doxorubicin with anti-PD-L1 -antibody [Citation186] or -peptide [Citation187] therapeutics, which enhanced IC50 concentrations against colon and breast cancer development. Importantly, such approaches showed encouraging effects at concentrations with little in the way of side effects and non-specific toxicity or instability.
In summary, most of the studies report very encouraging outcomes based on cancer-specific efficacies, in a variety of in vitro or in vivo mouse model systems, the treatments for which can also be optimized and controlled based on the mode of therapeutic delivery.
3. Clinical and future directions for CtsB targeting
From scrutinizing the scientific milestones highlighted in , what is apparent is that the area of PS-ABP (and Prodrug) development appears to be flourishing ahead of the research areas that incorporate the design and development of SMI anti-CtsB therapeutics. Whilst both strategies are not mutually exclusive in their mode of action, they can independently offer alternative and effective targeting approaches [Citation188,Citation189], essential for yielding robust Theranostics for the clinic.
Keeping with PS-ABP development, and upon scrutinizing the clinical trials database for keywords such as ‘cathepsin B’ (www.clinicaltrials.gov), we do find limited entries, but one of which does have great relevance (NCT03386942). Here, a CtsB cleavable linker conjugating Farletuzumab and Eribulin for the treatment of solid tumors [Citation190] is utilized in the form of MORAb-202, which demonstrated promising anti-tumor activity in FRα-positive tumors at well-tolerated doses. Despite this encouraging output, the Maximum Tolerated Dose was not defined and so further studies are needed. From another completed study, LUMO15 (NCT01626066) was well tolerated for tumor detection in a cohort of BC patients, and the full outcomes of which are eagerly awaited. Encouragingly (from both studies) therapeutic and diagnostic agents tested for CtsB targeting are realistic scientific endeavors and which set strong precedents for such technological advancements to continue, particularly in the context of targeting other Cts proteases.
Underpinning such progressive and encouraging outcomes are the convincing basic research studies aimed at targeting cancer-specific CtsB expression, through adopting a number of in vitro and in vivo approaches, that utilize ABP-derived probes, substrates, and Prodrugs. Whilst some agents are readily taken up by cells and permit the detection of some tumor cells and their boundaries, other agents target cells or components of the ECM (such as CtsB) that collectively modulate direct tumor development from the stroma. Clearly, such hopeful and encouraging findings have surfaced through combining state-of-the-art in vitro and in vivo systems (), and which are seen as sufficiently robust in permitting anti-CtsB agents to be characterized fully in a variety of cancer contexts, allowing the evaluation of important parameters, such as specificity, side effects, penetrability, half-lives, and tumor inhibition.
Clearly, with such rapid developments occurring, it may also be within the scope of such studies to target the biological effects of compartmentalized CtsB isoform proteins, as future studies evolve toward selectively targeting specific isoform proteins, at the subcellular or extracellular levels. Bearing in mind that some cathepsins and their isoforms do have clear input to chemoresistance, the above targeting approaches do consequently take on broader significance in offering better targeting specificity.
4. Conclusions
Over the last 3 years, the cathepsin proteases and their clinical applications have made great gains in the field of cancer research. Whilst they were originally thought of as proteases that had an important function in cellular homeostasis, their biological effects have been furthered as important players in a number of key regulatory mechanisms that span cell proliferation, survival, differentiation, and ECM regulation. As a versatile member of this family of proteases, clearly cathepsin B has been seen to have gathered heightened importance based on the wealth of knowledge that has been gained from its utilization as a diagnostic and prognostic marker for a number of cancers, and from it being therapeutically targeted and tested in preliminary clinical trials. When taken together, CtsB is clearly a protein that is shaping the paradigms through which we view this family of proteases from a basic-research perspective and with the forethought of how such findings may translate with tangible effects in the clinical.
5. Expert opinion
As a leading cause of global morbidity, significant focus is currently being placed on guiding the early detection and diagnosis of cancer by organizations such as the World Health Organization (https://www.who.int/news-room/fact-sheets/detail/cancer). To support such aims, great strides have been collectively made over the last 3 years in the areas of developing the technology (and hardware) that permits the detection of cancers and their there effective treatments, as reliable predictors for patient prognosis.
Against this scientific backdrop, we have focused on the CtsB protease, regulation, biological effects, and as a family member of proteins that are responsible for cancer development and growth, metastasis, chemoresistance, or tumor vascularization. Over the last 20 years, huge developments have been reported in areas spanning molecular mechanisms and rational drug design, with the potential to exploit such findings for diagnostic and prognostic purposes as a foresight. As we report herein, such progress has been impressive and has developed harmoniously, with tangible outcomes that arise from bringing closer inputs from basic research and their clinical applications. Here, the true benefits of such outcomes will arise from the speed at which accurate diagnoses can be made, whether such reagents have the power to resolve the stage of cancer development and the speed with which cancer patients can be treated effectively and monitored in real time. As the CtsB targeting-paradigm outlines herein, such benefits are realistic aims and are achievable, as demonstrated through the development and clinical testing of specific CtsB-specific Prodrugs. In doing so, it defines a ‘molecular framework’ with ‘work flows’ that can be adopted by- or applied to- other cathepsin members with emerging pathological importance. Consequently, future developments of this area do hold much promise as we sit at a very exciting juncture in time, where findings from model systems are beginning to be expeditiously tested within the clinic.
Article highlights
We introduce the cathepsin proteases and the significance of their expression in cancer
Cathepsin B is described in the context of its subcellular compartmentalization and contribution to cancer progression
We then proceed to describe its substrate specificity and how this is modulated by cystatin inhibitors
We extend such findings to describe the development of small-molecule inhibitors and Activity-Based Probes
We develop the significance of these to incorporate how such approaches have been adapted to develop cathepsin Prodrugs and their testing in the clinic
Looking forward, we conclude that cathepsin B inhibition does offer a robust paradigm that will serve to develop novel diagnostic and therapeutic agents through targeting other cathepsin members
Declaration of interests
The authors have no relevant affiliations or financial involvement with any organization or entity with a financial interest in or financial conflict with the subject matter or materials discussed in the manuscript. This includes employment, consultancies, honoraria, stock ownership or options, expert testimony, grants or patents received or pending, or royalties.
Reviewer disclosures
Peer reviewers on this manuscript have no relevant financial or other relationships to disclose.
Additional information
Funding
References
- Cao M, Luo X, Wu K, et al. Targeting lysosomes in human disease: from basic research to clinical applications. Signal Transduct Target Ther. 2021 Nov 8;6(1):379.
- Eskelinen EL, Tanaka Y, Saftig P. At the acidic edge: emerging functions for lysosomal membrane proteins. Trends Cell Biol. 2003 Mar;13(3):137–145.
- Soond SM, Kozhevnikova MV, Zamyatnin AA Jr. ‘Patchiness’ and basic cancer research: unravelling the proteases. Cell Cycle. 2019 Aug;18(15):1687–1701.
- Olson OC, Joyce JA. Cysteine cathepsin proteases: regulators of cancer progression and therapeutic response. Nat Rev Cancer. 2015 Dec;15(12):712–729.
- Uhlman A, Folkers K, Liston J, et al. Effects of Vacuolar H(+)-ATPase inhibition on activation of cathepsin B and cathepsin L secreted from MDA-MB231 breast cancer cells. Cancer Microenviron. 2017 Dec;10(1–3):49–56.
- Rozhin J, Sameni M, Ziegler G, et al. Pericellular pH affects distribution and secretion of cathepsin B in malignant cells. Cancer Res. 1994 Dec 15;54(24):6517–6525.
- Rothberg JM, Bailey KM, Wojtkowiak JW, et al. Acid-mediated tumor proteolysis: contribution of cysteine cathepsins. Neoplasia. 2013 Oct;15(10):1125–1137.
- Jordans S, Jenko-Kokalj S, Kuhl NM, et al. Monitoring compartment-specific substrate cleavage by cathepsins B, K, L, and S at physiological pH and redox conditions. BMC Biochem. 2009 Sep 22;10:23.
- Soond SM, Kozhevnikova MV, Frolova AS, et al. Lost or Forgotten: the nuclear cathepsin protein isoforms in cancer. Cancer Lett. 2019 Oct 10;462:43–50.
- Turk B, Turk D, Turk V. Lysosomal cysteine proteases: more than scavengers. Biochim Biophys Acta. 2000 Mar 7;1477(1–2):98–111.
- Soond SM, Zamyatnin AA Jr. Helicobacter pylori and gastric cancer: a lysosomal protease perspective. Gastric Cancer. 2022 Mar;25(2):306–324.
- Soond SM, Kozhevnikova MV, Savvateeva LV, et al. Intrinsically connected: therapeutically targeting the cathepsin proteases and the Bcl-2 family of protein substrates as co-regulators of apoptosis. Int J Mol Sci. 2021 Apr 28;22(9):4669.
- de Castro MA, Bunt G, Wouters FS. Cathepsin B launches an apoptotic exit effort upon cell death-associated disruption of lysosomes. Cell Death Discov. 2016;2:16012.
- Turk B, Dolenc I, Turk V, et al. Kinetics of the pH-induced inactivation of human cathepsin L. Biochemistry. 1993 Jan 12;32(1):375–380.
- Turk B, Bieth JG, Bjork I, et al. Regulation of the activity of lysosomal cysteine proteinases by pH-induced inactivation and/or endogenous protein inhibitors, cystatins. Biol Chem Hoppe-Seyler. 1995 Apr;376(4):225–230.
- Blum G, von Degenfeld G, Merchant MJ, et al. Noninvasive optical imaging of cysteine protease activity using fluorescently quenched activity-based probes. Nat Chem Biol. 2007 Oct;3(10):668–677.
- Sanman LE, Bogyo M. Activity-based profiling of proteases. Annu Rev Biochem. 2014;83:249–273.
- Poreba M, Groborz K, Vizovisek M, et al. Fluorescent probes towards selective cathepsin B detection and visualization in cancer cells and patient samples. Chem Sci. 2019 Sep 28;10(36):8461–8477.
- Edgington LE, Verdoes M, Bogyo M. Functional imaging of proteases: recent advances in the design and application of substrate-based and activity-based probes. Curr Opin Chem Biol. 2011 Dec;15(6):798–805.
- Jain M, Bouilloux J, Borrego I, et al. Cathepsin B-cleavable polymeric photosensitizer prodrug for selective photodynamic therapy: in vitro studies. Pharmaceuticals (Basel). 2022 Apr 30;15(5):564.
- Chowdhury MA, Moya IA, Bhilocha S, et al. Prodrug-inspired probes selective to cathepsin B over other cysteine cathepsins. J Med Chem. 2014 Jul 24;57(14):6092–6104.
- Soond SM, Kozhevnikova MV, Townsend PA, et al. Cysteine cathepsin protease inhibition: an update on its diagnostic, prognostic and therapeutic potential in cancer. Pharmaceuticals (Basel). 2019 Jun 11;12(2):87.
- Podobnik M, Kuhelj R, Turk V, et al. Crystal structure of the wild-type human procathepsin B at 2.5 A resolution reveals the native active site of a papain-like cysteine protease zymogen. J Mol Biol. 1997 Sep 5;271(5):774–788.
- Renko M, Pozgan U, Majera D, et al. Stefin A displaces the occluding loop of cathepsin B only by as much as required to bind to the active site cleft. FEBS J. 2010 Oct;277(20):4338–4345.
- Chan SJ, San Segundo B, McCormick MB, et al. Nucleotide and predicted amino acid sequences of cloned human and mouse preprocathepsin B cDNAs. Proc Natl Acad Sci U S A. 1986 Oct;83(20):7721–7725.
- Turk V, Stoka V, Vasiljeva O, et al. Cysteine cathepsins: from structure, function and regulation to new frontiers. Biochim Biophys Acta. 2012 Jan;1824(1):68–88.
- Sloane BF, Dunn JR, Honn KV. Lysosomal cathepsin B: correlation with metastatic potential. Science. 1981 Jun 5;212(4499):1151–1153.
- Ebert MP, Kruger S, Fogeron ML, et al. Overexpression of cathepsin B in gastric cancer identified by proteome analysis. Proteomics. 2005 Apr;5(6):1693–1704.
- Bengsch F, Buck A, Gunther SC, et al. Cell type-dependent pathogenic functions of overexpressed human cathepsin B in murine breast cancer progression. Oncogene. 2014 Sep 4;33(36):4474–4484.
- Fujise N, Nanashim A, Taniguchi Y, et al. Prognostic impact of cathepsin B and matrix metalloproteinase-9 in pulmonary adenocarcinomas by immunohistochemical study. Lung Cancer. 2000 Jan;27(1):19–26.
- Mort JS, Buttle DJ. Cathepsin B. Int J Biochem Cell Biol. 1997 May;29(5):715–720.
- Galjart NJ, Gillemans N, Harris A, et al. Expression of cDNA encoding the human “protective protein” associated with lysosomal beta-galactosidase and neuraminidase: homology to yeast proteases. Cell. 1988 Sep 9;54(6):755–764.
- San Segundo B, Chan SJ, Steiner DF. Differences in cathepsin B mRNA levels in rat tissues suggest specialized functions. FEBS Lett. 1986 Jun 9;201(2):251–256.
- Ibrahim SA, El-Ghonaimy EA, Hassan H, et al. Hormonal-receptor positive breast cancer: IL-6 augments invasion and lymph node metastasis via stimulating cathepsin B expression. J Adv Res. 2016 Sep;7(5):661–670.
- Yan S, Berquin IM, Troen BR, et al. Transcription of human cathepsin B is mediated by Sp1 and Ets family factors in glioma. DNA Cell Biol. 2000 Feb;19(2):79–91.
- Yan S, Jane DT, Dufresne MJ, et al. Transcription of cathepsin B in glioma cells: regulation by an E-box adjacent to the transcription initiation site. Biol Chem. 2003 Oct-Nov;384(10–11):1421–1427.
- Saffari M, Dinehkabodi OS, Ghaffari SH, et al. Identification of novel p53 target genes by cDNA AFLP in glioblastoma cells. Cancer Lett. 2009 Jan 18;273(2):316–322.
- Sun T, Jiang D, Zhang L, et al. Expression profile of cathepsins indicates the potential of cathepsins B and D as prognostic factors in breast cancer patients. Oncol Lett. 2016 Jan;11(1):575–583.
- Soond SM, Savvateeva LV, Makarov VA, et al. Making connections: p53 and the cathepsin proteases as co-regulators of cancer and apoptosis. Cancers (Basel). 2020 Nov 22;12(11):3476.
- Soond SM, Kozhevnikova MV, Townsend PA, et al. Integrative p53, micro-RNA and cathepsin protease co-regulatory expression networks in cancer. Cancers (Basel). 2020 Nov 20;12(11):3454.
- Luan H, Mohapatra B, Bielecki TA, et al. Loss of the nuclear pool of ubiquitin ligase CHIP/STUB1 in breast cancer unleashes the MZF1-Cathepsin Pro-oncogenic Program. Cancer Res. 2018 May 15;78(10):2524–2535.
- Tripathi R, Fiore LS, Richards DL, et al. Abl and Arg mediate cysteine cathepsin secretion to facilitate melanoma invasion and metastasis. Sci Signal. 2018 Feb 20;11(518):518.
- Lin YY, Chen ZW, Lin ZP, et al. Tissue levels of Stefin A and Stefin B in hepatocellular carcinoma. Anat Rec (Hoboken). 2016 Apr;299(4):428–438.
- Zhang J, He P, Zhong Q, et al. Increasing cystatin C and cathepsin B in serum of colorectal cancer patients. Clin Lab. 2017 Feb 1;63(2):365–371.
- Tan G, Liu Q, Tang X, et al. Diagnostic values of serum cathepsin B and D in patients with nasopharyngeal carcinoma. BMC Cancer. 2016 Mar 19;16:241.
- Edgington-Mitchell LE, Bogyo M, Verdoes M. Live cell imaging and profiling of cysteine cathepsin activity using a quenched activity-based probe. Methods Mol Biol. 2017;1491:145–159.
- Abe S, Sukoh N, Ogura S, et al. Clinical indicators of malignancy of lung cancer. Hokkaido Igaku Zasshi. 1994 May;69(3):391–395.
- Featherston T, Marsh RW, van Schaijik B, et al. Expression and localization of cathepsins B, D, and G in two cancer stem cell subpopulations in moderately differentiated oral tongue squamous cell carcinoma. Front Med (Lausanne). 2017;4:100.
- Mehrotra S, Wickremesekera SK, Brasch HD, et al. Expression and localization of cathepsins B, D and G in cancer stem cells in liver metastasis from colon adenocarcinoma. Front Surg. 2018;5:40.
- Ma X, Wang Y, Fan H, et al. Genetic polymorphisms of Cathepsin B are associated with gastric cancer risk and prognosis in a Chinese population. Cancer Biomark. 2021;32(2):189–198.
- Breznik B, Limbaeck Stokin C, Kos J, et al. Cysteine cathepsins B, X and K expression in peri-arteriolar glioblastoma stem cell niches. J Mol Histol. 2018 Oct;49(5):481–497.
- Pozgan U, Caglic D, Rozman B, et al. Expression and activity profiling of selected cysteine cathepsins and matrix metalloproteinases in synovial fluids from patients with rheumatoid arthritis and osteoarthritis. Biol Chem. 2010 May;391(5):571–579.
- Krueger S, Haeckel C, Buehling F, et al. Inhibitory effects of antisense cathepsin B cDNA transfection on invasion and motility in a human osteosarcoma cell line. Cancer Res. 1999 Dec 1;59(23):6010–6014.
- Withana NP, Blum G, Sameni M, et al. Cathepsin B inhibition limits bone metastasis in breast cancer. Cancer Res. 2012 Mar 1;72(5):1199–1209.
- Bian B, Mongrain S, Cagnol S, et al. Cathepsin B promotes colorectal tumorigenesis, cell invasion, and metastasis. Mol Carcinog. 2016 May;55(5):671–687.
- Chan AT, Baba Y, Shima K, et al. Cathepsin B expression and survival in colon cancer: implications for molecular detection of neoplasia. Cancer Epidemiol Biomarkers Prev. 2010 Nov;19(11):2777–2785.
- Troy AM, Sheahan K, Mulcahy HE, et al. Expression of Cathepsin B and L antigen and activity is associated with early colorectal cancer progression. Eur J Cancer. 2004 Jul;40(10):1610–1616.
- Hirai K, Yokoyama M, Asano G, et al. Expression of cathepsin B and cystatin C in human colorectal cancer. Hum Pathol. 1999 Jun;30(6):680–686.
- Yan S, Sloane BF. Molecular regulation of human cathepsin B: implication in pathologies. Biol Chem. 2003 Jun;384(6):845–854.
- Mehtani S, Gong Q, Panella J, et al. In vivo expression of an alternatively spliced human tumor message that encodes a truncated form of cathepsin B. Subcellular distribution of the truncated enzyme in COS cells. J Biol Chem. 1998 May 22;273(21):13236–13244.
- Gong Q, Chan SJ, Bajkowski AS, et al. Characterization of the cathepsin B gene and multiple mRNAs in human tissues: evidence for alternative splicing of cathepsin B pre-mRNA. DNA Cell Biol. 1993 May;12(4):299–309.
- Berquin IM, Cao L, Fong D, et al. Identification of two new exons and multiple transcription start points in the 5’-untranslated region of the human cathepsin-B-encoding gene. Gene. 1995 Jul 4;159(2):143–149.
- Muntener K, Zwicky R, Csucs G, et al. The alternative use of exons 2 and 3 in cathepsin B mRNA controls enzyme trafficking and triggers nuclear fragmentation in human cells. Histochem Cell Biol. 2003 Feb;119(2):93–101.
- Hizel C, Ferrara M, Cure H, et al. Evaluation of the 5’ spliced form of human cathepsin B mRNA in colorectal mucosa and tumors. Oncol Rep. 1998 Jan-Feb;5(1):31–34.
- Lemaire R, Flipo RM, Migaud H, et al. Alternative splicing of the 5’ region of cathepsin B pre-messenger RNA in rheumatoid synovial tissue. Arthritis Rheum. 1997 Aug;40(8):1540–1542.
- Aggarwal N, Sloane BF. Cathepsin B: multiple roles in cancer. Proteomics Clin Appl. 2014 Jun;8(5–6):427–437.
- Mach L, Mort JS, Glossl J. Noncovalent complexes between the lysosomal protease cathepsin B and its propeptide account for stable, extracellular, high molecular mass forms of the enzyme. J Biol Chem. 1994 Apr 29;269(17):13036–13040.
- Dalet-Fumeron V, Guinec N, Pagano M. In vitro activation of pro-cathepsin B by three serine proteinases: leukocyte elastase, cathepsin G, and the urokinase-type plasminogen activator. FEBS Lett. 1993 Oct 18;332(3):251–254.
- Gocheva V, Joyce JA. Cysteine cathepsins and the cutting edge of cancer invasion. Cell Cycle. 2007 Jan 1;6(1):60–64.
- Lin J, McCann AP, Sereesongsaeng N, et al. USP17 is required for peripheral trafficking of lysosomes. EMBO Rep. 2022 Apr 5;23(4):e51932.
- Zwicky R, Muntener K, Csucs G, et al. Exploring the role of 5’ alternative splicing and of the 3’-untranslated region of cathepsin B mRNA. Biol Chem. 2003 Jul;384(7):1007–1018.
- Fehrenbacher N, Bastholm L, Kirkegaard-Sorensen T, et al. Sensitization to the lysosomal cell death pathway by oncogene-induced down-regulation of lysosome-associated membrane proteins 1 and 2. Cancer Res. 2008 Aug 15;68(16):6623–6633.
- Eaton JW, Qian M. Molecular bases of cellular iron toxicity. Free Radic Biol Med. 2002 May 1;32(9):833–840.
- Kurz T, Terman A, Gustafsson B, et al. Lysosomes and oxidative stress in aging and apoptosis. Biochim Biophys Acta. 2008 Nov;1780(11):1291–1303.
- Ullio C, Casas J, Brunk UT, et al. Sphingosine mediates TNFalpha-induced lysosomal membrane permeabilization and ensuing programmed cell death in hepatoma cells. J Lipid Res. 2012 Jun;53(6):1134–1143.
- Mora R, Dokic I, Kees T, et al. Sphingolipid rheostat alterations related to transformation can be exploited for specific induction of lysosomal cell death in murine and human glioma. Glia. 2010 Aug 15;58(11):1364–1383.
- Taha TA, Kitatani K, Bielawski J, et al. Tumor necrosis factor induces the loss of sphingosine kinase-1 by a cathepsin B-dependent mechanism. J Biol Chem. 2005 Apr 29;280(17):17196–17202.
- Dielschneider RF, Henson ES, Gibson SB. Lysosomes as oxidative targets for cancer therapy. Oxid Med Cell Longev. 2017;2017:3749157.
- Ostenfeld MS, Fehrenbacher N, Hoyer-Hansen M, et al. Effective tumor cell death by sigma-2 receptor ligand siramesine involves lysosomal leakage and oxidative stress. Cancer Res. 2005 Oct 1;65(19):8975–8983.
- Ostenfeld MS, Hoyer-Hansen M, Bastholm L, et al. Anti-cancer agent siramesine is a lysosomotropic detergent that induces cytoprotective autophagosome accumulation. Autophagy. 2008 May;4(4):487–499.
- Wang Y, Niu H, Hu Z, et al. Targeting the lysosome by an aminomethylated Riccardin D triggers DNA damage through cathepsin B-mediated degradation of BRCA1. J Cell Mol Med. 2019 Mar;23(3):1798–1812.
- Chung PED, Gendoo DMA, Ghanbari-Azarnier R, et al. Modeling germline mutations in pineoblastoma uncovers lysosome disruption-based therapy. Nat Commun. 2020 Apr 14;11(1):1825.
- Yuan X, Nie W, He Z, et al. Carbon black nanoparticles induce cell necrosis through lysosomal membrane permeabilization and cause subsequent inflammatory response. Theranostics. 2020;10(10):4589–4605.
- Bestvater F, Dallner C, Spiess E. The C-terminal subunit of artificially truncated human cathepsin B mediates its nuclear targeting and contributes to cell viability. BMC Cell Biol. 2005 Apr 4;6(1):16.
- Meng J, Liu Y, Xie Z, et al. Nucleus distribution of cathepsin B in senescent microglia promotes brain aging through degradation of sirtuins. Neurobiol Aging. 2020;96:255–266.
- Xu D, Wang J. Downregulation of cathepsin B reduces proliferation and inflammatory response and facilitates differentiation in human HaCaT keratinocytes, ameliorating IL-17A and SAA-induced psoriasis-like lesion. Inflammation. 2021 Oct;44(5):2006–2017.
- Bach AS, Derocq D, Laurent-Matha V, et al. Nuclear cathepsin D enhances TRPS1 transcriptional repressor function to regulate cell cycle progression and transformation in human breast cancer cells. Oncotarget. 2015 Sep 29;6(29):28084–28103.
- Yang Z, Liu Y, Qin L, et al. Cathepsin H-mediated degradation of HDAC4 for matrix metalloproteinase expression in hepatic stellate cells: implications of epigenetic suppression of matrix metalloproteinases in fibrosis through stabilization of class iia histone deacetylases. Am J Pathol. 2017 Apr;187(4):781–797.
- Goulet B, Baruch A, Moon NS, et al. A cathepsin L isoform that is devoid of a signal peptide localizes to the nucleus in S phase and processes the CDP/Cux transcription factor. Mol Cell. 2004 Apr 23;14(2):207–219.
- Fei Y, Xiong Y, Shen X, et al. Cathepsin L promotes ionizing radiation-induced U251 glioma cell migration and invasion through regulating the GSK-3beta/CUX1 pathway. Cell Signal. 2018;44:62–71.
- Goulet B, Sansregret L, Leduy L, et al. Increased expression and activity of nuclear cathepsin L in cancer cells suggests a novel mechanism of cell transformation. Mol Cancer Res. 2007 Sep;5(9):899–907.
- Soond SM, Savvateeva LV, Makarov VA, et al. Cathepsin S cleaves BAX as a novel and therapeutically important regulatory mechanism for apoptosis. Pharmaceutics. 2021 Mar 5;13(3):339.
- Tedelind S, Poliakova K, Valeta A, et al. Nuclear cysteine cathepsin variants in thyroid carcinoma cells. Biol Chem. 2010 Aug;391(8):923–935.
- Muntener K, Zwicky R, Csucs G, et al. Exon skipping of cathepsin B: mitochondrial targeting of a lysosomal peptidase provokes cell death. J Biol Chem. 2004 Sep 24;279(39):41012–41017.
- Baici A, Muntener K, Willimann A, et al. Regulation of human cathepsin B by alternative mRNA splicing: homeostasis, fatal errors and cell death. Biol Chem. 2006 Aug;387(8):1017–1021.
- Burton LJ, Henderson V, Liburd L, et al. Snail transcription factor NLS and importin beta1 regulate the subcellular localization of Cathepsin L and Cux1. Biochem Biophys Res Commun. 2017 Sep 9;491(1):59–64.
- Pouyssegur J, Marchiq I, Parks SK, et al. ‘Warburg effect’ controls tumor growth, bacterial, viral infections and immunity - Genetic deconstruction and therapeutic perspectives. Semin Cancer Biol. 2022 Nov;86(Pt2):334–346.
- Zhelev Z, Aoki I, Lazarova D, et al. A “weird” mitochondrial fatty acid oxidation as a metabolic “secret” of cancer. Oxid Med Cell Longev. 2022;2022:2339584.
- Gocheva V, Zeng W, Ke D, et al. Distinct roles for cysteine cathepsin genes in multistage tumorigenesis. Genes Dev. 2006 Mar 1;20(5):543–556.
- Guinec N, Dalet-Fumeron V, Pagano M. “In vitro” study of basement membrane degradation by the cysteine proteinases, cathepsins B, B-like and L. Digestion of collagen IV, laminin, fibronectin, and release of gelatinase activities from basement membrane fibronectin. Biol Chem Hoppe-Seyler. 1993 Dec;374(12):1135–1146.
- Mai J, Sameni M, Mikkelsen T, et al. Degradation of extracellular matrix protein tenascin-C by cathepsin B: an interaction involved in the progression of gliomas. Biol Chem. 2002 Sep;383(9):1407–1413.
- Veillard F, Saidi A, Burden RE, et al. Cysteine cathepsins S and L modulate anti-angiogenic activities of human endostatin. J Biol Chem. 2011 Oct 28;286(43):37158–37167.
- Joyce JA, Baruch A, Chehade K, et al. Cathepsin cysteine proteases are effectors of invasive growth and angiogenesis during multistage tumorigenesis. Cancer Cell. 2004 May;5(5):443–453.
- Fukuda S, Schmid-Schonbein GW. Regulation of CD18 expression on neutrophils in response to fluid shear stress. Proc Natl Acad Sci U S A. 2003 Nov 11;100(23):13152–13157.
- Nakao S, Zandi S, Sun D, et al. Cathepsin B-mediated CD18 shedding regulates leukocyte recruitment from angiogenic vessels. FASEB J. 2018 Jan;32(1):143–154.
- Im E, Venkatakrishnan A, Kazlauskas A. Cathepsin B regulates the intrinsic angiogenic threshold of endothelial cells. Mol Biol Cell. 2005 Aug;16(8):3488–3500.
- Kramer L, Turk D, Turk B. The future of cysteine cathepsins in disease management. Trends Pharmacol Sci. 2017 Oct;38(10):873–898.
- de Duve C. The lysosome turns fifty. Nat Cell Biol. 2005 Sep;7(9):847–849.
- Aits S, Jaattela M. Lysosomal cell death at a glance. J Cell Sci. 2013 May 1;126(Pt 9):1905–1912.
- Cirman T, Oresic K, Mazovec GD, et al. Selective disruption of lysosomes in HeLa cells triggers apoptosis mediated by cleavage of Bid by multiple papain-like lysosomal cathepsins. J Biol Chem. 2004 Jan 30;279(5):3578–3587.
- Garnett TO, Filippova M, Duerksen-Hughes PJ. Bid is cleaved upstream of caspase-8 activation during TRAIL-mediated apoptosis in human osteosarcoma cells. Apoptosis. 2007 Jul;12(7):1299–1315.
- Reiners JJ Jr., Caruso JA, Mathieu P, et al. Release of cytochrome c and activation of pro-caspase-9 following lysosomal photodamage involves Bid cleavage. Cell Death Differ. 2002 Sep;9(9):934–944.
- Conus S, Perozzo R, Reinheckel T, et al. Caspase-8 is activated by cathepsin D initiating neutrophil apoptosis during the resolution of inflammation. J Exp Med. 2008 Mar 17;205(3):685–698.
- Guicciardi ME, Deussing J, Miyoshi H, et al. Cathepsin B contributes to TNF-alpha-mediated hepatocyte apoptosis by promoting mitochondrial release of cytochrome c. J Clin Invest. 2000 Nov;106(9):1127–1137.
- Salvesen GS. A lysosomal protease enters the death scene. J Clin Invest. 2001 Jan;107(1):21–22.
- Droga-Mazovec G, Bojic L, Petelin A, et al. Cysteine cathepsins trigger caspase-dependent cell death through cleavage of Bid and antiapoptotic Bcl-2 homologues. J Biol Chem. 2008 Jul 4;283(27):19140–19150.
- Yoon MC, Solania A, Jiang Z, et al. Selective neutral pH inhibitor of cathepsin B designed based on cleavage preferences at cytosolic and lysosomal pH conditions. ACS Chem Biol. 2021 Sep 17;16(9):1628–1643.
- Turk V, Stoka V, Turk D. Cystatins: biochemical and structural properties, and medical relevance. Front Biosci. 2008 May 1;13:5406–5420.
- Breznik B, Mitrovic A, TL T, et al. Cystatins in cancer progression: more than just cathepsin inhibitors. Biochimie. 2019;166:233–250.
- Turk V, Bode W. The cystatins: protein inhibitors of cysteine proteinases. FEBS Lett. 1991 Jul 22;285(2):213–219.
- Abrahamson M, Barrett AJ, Salvesen G, et al. Isolation of six cysteine proteinase inhibitors from human urine. Their physicochemical and enzyme kinetic properties and concentrations in biological fluids. J Biol Chem. 1986 Aug 25;261(24):11282–11289.
- Ni J, Fernandez MA, Danielsson L, et al. Cystatin F is a glycosylated human low molecular weight cysteine proteinase inhibitor. J Biol Chem. 1998 Sep 18;273(38):24797–24804.
- Cygler M, Sivaraman J, Grochulski P, et al. Structure of rat procathepsin B: model for inhibition of cysteine protease activity by the proregion. Structure. 1996 Apr 15;4(4):405–416.
- Jia Z, Hasnain S, Hirama T, et al. Crystal structures of recombinant rat cathepsin B and a cathepsin B-inhibitor complex. Implications for structure-based inhibitor design. J Biol Chem. 1995 Mar 10;270(10):5527–5533.
- Musil D, Zucic D, Turk D, et al. The refined 2.15 A X-ray crystal structure of human liver cathepsin B: the structural basis for its specificity. EMBO J. 1991 Sep;10(9):2321–2330.
- Turk D, Podobnik M, Kuhelj R, et al. Crystal structures of human procathepsin B at 3.2 and 3.3 Angstroms resolution reveal an interaction motif between a papain-like cysteine protease and its propeptide. FEBS Lett. 1996 Apr 22;384(3):211–214.
- Turk D, Podobnik M, Popovic T, et al. Crystal structure of cathepsin B inhibited with CA030 at 2.0-A resolution: a basis for the design of specific epoxysuccinyl inhibitors. Biochemistry. 1995 Apr 11;34(14):4791–4797.
- Naudin C, Lecaille F, Chowdhury S, et al. The occluding loop of cathepsin B prevents its effective inhibition by human kininogens. J Mol Biol. 2010 Jul 30;400(5):1022–1035.
- Nagler DK, Storer AC, Portaro FC, et al. Major increase in endopeptidase activity of human cathepsin B upon removal of occluding loop contacts. Biochemistry. 1997 Oct 14;36(41):12608–12615.
- Illy C, Quraishi O, Wang J, et al. Role of the occluding loop in cathepsin B activity. J Biol Chem. 1997 Jan 10;272(2):1197–1202.
- Rozman J, Stojan J, Kuhelj R, et al. Autocatalytic processing of recombinant human procathepsin B is a bimolecular process. FEBS Lett. 1999 Oct 15;459(3):358–362.
- Pungercar JR, Caglic D, Sajid M, et al. Autocatalytic processing of procathepsin B is triggered by proenzyme activity. FEBS J. 2009 Feb;276(3):660–668.
- Quraishi O, Nagler DK, Fox T, et al. The occluding loop in cathepsin B defines the pH dependence of inhibition by its propeptide. Biochemistry. 1999 Apr 20;38(16):5017–5023.
- Sloane BF, Rozhin J, Krepela E, et al. The malignant phenotype and cysteine proteinases. Biomed Biochim Acta. 1991;50(4–6):549–554.
- Caglic D, Pungercar JR, Pejler G, et al. Glycosaminoglycans facilitate procathepsin B activation through disruption of propeptide-mature enzyme interactions. J Biol Chem. 2007 Nov 9;282(45):33076–33085.
- Fujimoto T, Tsunedomi R, Matsukuma S, et al. Cathepsin B is highly expressed in pancreatic cancer stem-like cells and is associated with patients’ surgical outcomes. Oncol Lett. 2021 Jan;21(1):30.
- Mitrovic A, Mirkovic B, Sosic I, et al. Inhibition of endopeptidase and exopeptidase activity of cathepsin B impairs extracellular matrix degradation and tumor invasion. Biol Chem. 2016 Jan 1;397(2):165–174.
- Jedeszko C, Sloane BF. Cysteine cathepsins in human cancer. Biol Chem. 2004 Nov;385(11):1017–1027.
- Wang SJ, Chao D, Wei W, et al. CD147 promotes collective invasion through cathepsin B in hepatocellular carcinoma. J Exp Clin Cancer Res. 2020 Jul 29;39(1):145.
- Ma K, Chen X, Liu W, et al. CTSB is a negative prognostic biomarker and therapeutic target associated with immune cells infiltration and immunosuppression in gliomas. Sci Rep. 2022 Mar 11;12(1):4295.
- Humphries F, Chang-McDonald B, Patel J, et al. Cathepsins B, D, and G are expressed in metastatic head and neck cutaneous squamous cell carcinoma. Front Oncol. 2021;11:690460.
- Pandey G, Bakhshi S, Kumar M, et al. Prognostic and therapeutic relevance of cathepsin B in pediatric acute myeloid leukemia. Am J Cancer Res. 2019;9(12):2634–2649.
- Kozlowski L, Rucinska M, Wojtukiewicz MZ. Metastases of malignant melanoma of unknown primary site: an important diagnostic and therapeutic problem. Pol Merkur Lekarski. 2000 Jul;8(49):486–488.
- Yan Y, Zhou K, Wang L, et al. Clinical significance of serum cathepsin B and cystatin C levels and their ratio in the prognosis of patients with esophageal cancer. Onco Targets Ther. 2017;10:1947–1954.
- Rudzinska-Radecka M, Frolova AS, Balakireva AV, et al. In silico, in vitro, and clinical investigations of cathepsin b and stefin A mRNA expression and a correlation analysis in kidney cancer. Cells. 2022 Apr 25;11(9):1455.
- Choe Y, Leonetti F, Greenbaum DC, et al. Substrate profiling of cysteine proteases using a combinatorial peptide library identifies functionally unique specificities. J Biol Chem. 2006 May 5;281(18):12824–12832.
- Schmitz J, Gilberg E, Loser R, et al. Cathepsin B: active site mapping with peptidic substrates and inhibitors. Bioorg Med Chem. 2019 Jan 1;27(1):1–15.
- Li YY, Fang J, Ao GZ. Cathepsin B and L inhibitors: a patent review (2010 - present). Expert Opin Ther Pat. 2017 Jun;27(6):643–656.
- Hasnain S, Hirama T, Huber CP, et al. Characterization of cathepsin B specificity by site-directed mutagenesis. Importance of Glu245 in the S2-P2 specificity for arginine and its role in transition state stabilization. J Biol Chem. 1993 Jan 5;268(1):235–240.
- Kato D, Boatright KM, Berger AB, et al. Activity-based probes that target diverse cysteine protease families. Nat Chem Biol. 2005 Jun;1(1):33–38.
- Lopez-Otin C, Overall CM. Protease degradomics: a new challenge for proteomics. Nat Rev Mol Cell Biol. 2002 Jul;3(7):509–519.
- Blum G, Weimer RM, Edgington LE, et al. Comparative assessment of substrates and activity based probes as tools for non-invasive optical imaging of cysteine protease activity. PLoS One. 2009 Jul 28;4(7):e6374.
- Abd-Elrahman I, Kosuge H, Wises Sadan T, et al. Cathepsin activity-based probes and inhibitor for preclinical atherosclerosis imaging and macrophage depletion. PLoS One. 2016;11(8):e0160522.
- Ben-Aderet L, Merquiol E, Fahham D, et al. Detecting cathepsin activity in human osteoarthritis via activity-based probes. Arthritis Res Ther. 2015 Mar 20;17:69.
- Bhuiyan AI, Rathod P, Ghoshal S, et al. Clickable, selective, and cell-permeable activity-based probe of human cathepsin B - Minimalistic approach for enhanced selectivity. Bioorg Chem. 2021;117:105463.
- Oresic Bender K, Ofori L, van der Linden WA, et al. Design of a highly selective quenched activity-based probe and its application in dual color imaging studies of cathepsin S activity localization. J Am Chem Soc. 2015 Apr 15;137(14):4771–4777.
- Sun N, Wang D, Yao G, et al. pH-dependent and cathepsin B activable CaCO3 nanoprobe for targeted in vivo tumor imaging. Int J Nanomedicine. 2019;14:4309–4317.
- Wang S, Vigliarolo BG, Chowdhury MA, et al. Design and synthesis of fluorogenic substrate-based probes for detecting Cathepsin B activity. Bioorg Chem. 2019;92:103194.
- Shen Y, Wu T, Wang Y, et al. Nucleolin-targeted ratiometric fluorescent carbon dots with a remarkably large emission wavelength shift for precise imaging of cathepsin b in living cancer cells. Anal Chem. 2021 Mar 2;93(8):4042–4050.
- Tam LKB, Yu L, Wong RCH, et al. Dual cathepsin b and glutathione-activated dimeric and trimeric phthalocyanine-based photodynamic molecular beacons for targeted photodynamic therapy. J Med Chem. 2021 Dec 9;64(23):17455–17467.
- Wang Y, Jiang L, Zhang Y, et al. Fibronectin-targeting and cathepsin B-activatable theranostic nanoprobe for MR/fluorescence imaging and enhanced photodynamic therapy for triple negative breast cancer. ACS Appl Mater Interfaces. 2020 Jul 29;12(30):33564–33574.
- Tan P, Cai H, Wei Q, et al. Enhanced chemo-photodynamic therapy of an enzyme-responsive prodrug in bladder cancer patient-derived xenograft models. Biomaterials. 2021;277:121061.
- Kramer L, Renko M, Zavrsnik J, et al. Non-invasive in vivo imaging of tumor-associated cathepsin B by a highly selective inhibitory DARPin. Theranostics. 2017;7(11):2806–2821.
- Schenker P, Alfarano P, Kolb P, et al. A double-headed cathepsin B inhibitor devoid of warhead. Protein Sci. 2008 Dec;17(12):2145–2155.
- Murata M, Miyashita S, Yokoo C, et al. Novel epoxysuccinyl peptides. Selective inhibitors of cathepsin B, in vitro. FEBS Lett. 1991 Mar 25;280(2):307–310.
- Raghav N, Singh M. SAR studies of some acetophenone phenylhydrazone based pyrazole derivatives as anticathepsin agents. Bioorg Chem. 2017 Dec;75:38–49.
- Kim J, Shim MK, Cho YJ, et al. The safe and effective intraperitoneal chemotherapy with cathepsin B-specific doxorubicin prodrug nanoparticles in ovarian cancer with peritoneal carcinomatosis. Biomaterials. 2021;279:121189.
- Herceg V, Bouilloux J, Janikowska K, et al. Cathepsin B-cleavable cyclopeptidic chemotherapeutic prodrugs. Molecules. 2020 Sep 18;25(18):4285.
- Zhang HJ, Zhao X, Chen LJ, et al. pH-driven targeting nanoprobe with dual-responsive drug release for persistent luminescence imaging and chemotherapy of tumor. Anal Chem. 2020 Jan 7;92(1):1179–1188.
- Shim MK, Park J, Yoon HY, et al. Carrier-free nanoparticles of cathepsin B-cleavable peptide-conjugated doxorubicin prodrug for cancer targeting therapy. J Control Release. 2018 Dec 11;294:376–389.
- Zhi X, Jiang Y, Xie L, et al. Gold nanorods functionalized with cathepsin B targeting peptide and doxorubicin for combinatorial therapy against multidrug resistance. ACS Appl Bio Mater. 2019 Dec 16;2(12):5697–5706.
- Ben-Nun Y, Fichman G, Adler-Abramovich L, et al. Cathepsin nanofiber substrates as potential agents for targeted drug delivery. J Control Release. 2017 Jul 10;257:60–67.
- Lee S, Song SJ, Lee J, et al. Cathepsin B-responsive liposomes for controlled anticancer drug delivery in hep G2 cells. Pharmaceutics. 2020 Sep 14;12(9):876.
- Cho H, Shim MK, Yang S, et al. Cathepsin B-overexpressed tumor cell activatable albumin-binding doxorubicin prodrug for cancer-targeted therapy. Pharmaceutics. 2021 Dec 29;14(1):83.
- Schnorenberg MR, Bellairs JA, Samaeekia R, et al. Activating the intrinsic pathway of apoptosis using BIM BH3 peptides delivered by peptide amphiphiles with endosomal release. Materials (Basel). 2019 Aug 12;12(16):2567.
- Ehrsam D, Porta F, Hussner J, et al. PDMS-PMOXA-nanoparticles featuring a cathepsin b-triggered release mechanism. Materials (Basel). 2019 Sep 3;12(17):2836.
- Luo Y, Sun X, Huang L, et al. Artemisinin-based smart nanomedicines with self-supply of ferrous ion to enhance oxidative stress for specific and efficient cancer treatment. ACS Appl Mater Interfaces. 2019 Aug 21;11(33):29490–29497.
- Jin X, Zhang J, Jin X, et al. Folate receptor targeting and cathepsin b-sensitive drug delivery system for selective cancer cell death and imaging. ACS Med Chem Lett. 2020 Aug 13;11(8):1514–1520.
- Saito K, Iioka H, Kojima C, et al. Peptide-based tumor inhibitor encoding mitochondrial p14(ARF) is highly efficacious to diverse tumors. Cancer Sci. 2016 Sep;107(9):1290–1301.
- Shim MK, Moon Y, Yang S, et al. Cancer-specific drug-drug nanoparticles of pro-apoptotic and cathepsin B-cleavable peptide-conjugated doxorubicin for drug-resistant cancer therapy. Biomaterials. 2020;261:120347.
- Narayanan P, Anitha AK, Ajayakumar N, et al. Poly-lysine dendritic nanocarrier to target epidermal growth factor receptor overexpressed breast cancer for methotrexate delivery. Materials (Basel). 2022 Jan 21;15(3):800.
- Wang W, Zhang X, Li Z, et al. Dendronized hyaluronic acid-docetaxel conjugate as a stimuli-responsive nano-agent for breast cancer therapy. Carbohydr Polym. 2021 Sep 1;267:118160.
- Kim J, Shim MK, Yang S, et al. Combination of cancer-specific prodrug nanoparticle with Bcl-2 inhibitor to overcome acquired drug resistance. J Control Release. 2021 Feb 10;330:920–932.
- Cheng X, Li J, Tanaka K, et al. MORAb-202, an antibody-drug conjugate utilizing humanized anti-human FRalpha farletuzumab and the microtubule-targeting agent eribulin, has potent antitumor activity. Mol Cancer Ther. 2018 Dec;17(12):2665–2675.
- Furuuchi K, Rybinski K, Fulmer J, et al. Antibody-drug conjugate MORAb-202 exhibits long-lasting antitumor efficacy in TNBC PDx models. Cancer Sci. 2021 Jun;112(6):2467–2480.
- Yang S, Shim MK, Kim WJ, et al. Cancer-activated doxorubicin prodrug nanoparticles induce preferential immune response with minimal doxorubicin-related toxicity. Biomaterials. 2021;272:120791.
- Moon Y, Shim MK, Choi J, et al. Anti-PD-L1 peptide-conjugated prodrug nanoparticles for targeted cancer immunotherapy combining PD-L1 blockade with immunogenic cell death. Theranostics. 2022;12(5):1999–2014.
- Korkmaz B, Lamort AS, Domain R, et al. Cathepsin C inhibition as a potential treatment strategy in cancer. Biochem Pharmacol. 2021;194:114803.
- Vizovisek M, Vidak E, Javorsek U, et al. Cysteine cathepsins as therapeutic targets in inflammatory diseases. Expert Opin Ther Targets. 2020 Jun;24(6):573–588.
- Shimizu T, Fujiwara Y, Yonemori K, et al. First-in-human phase 1 study of MORAb-202, an antibody-drug conjugate comprising farletuzumab linked to eribulin mesylate, in patients with folate receptor-alpha-positive advanced solid tumors. Clin Cancer Res. 2021 Jul 15;27(14):3905–3915.