ABSTRACT
Background
Major Depressive Disorder (MDD) poses a significant challenge to global health, with current treatments often limited by efficacy and onset delays. This study explores the synergistic antidepressant-like effects of an NPY1R agonist and Ketamine, targeting their neurobiological interactions within the ventral hippocampus.
Research Design and Methods
Utilizing a preclinical model, this study administered Neuropeptide Y receptor 1 (NPY1R) agonist and Ketamine, both separately and in combination, through intracerebroventricular (icv) and intranasal (i.n.) routes. The Forced Swimming Test (FST) was employed to assess antidepressant-like activity, while in situ Proximity Ligation Assay and immunohistochemistry were used to examine NPY1R/TrkB heteroreceptor complexes and BDNF expression in the ventral dentate gyrus (DG), along with neurogenesis markers.
Results
The combined treatment significantly reduced immobility in the FST, indicative of enhanced antidepressant-like effects, correlated with increased formation of NPY1R/TrkB complex and brain-derived neurotrophic factor (BDNF) expression in the ventral DG. These molecular alterations were associated with increased neurogenesis.
Conclusions
The coadministration of an NPY1R agonist and Ketamine in a rodent model demonstrated potentiated antidepressant responses through synergistic neurobiological pathways, including TrkB signaling and hippocampal neurogenesis. This indicates a novel therapeutic strategy for MDD, warranting further clinical investigation to fully understand its implications.
1. Introduction
Major depressive disorder (MDD) stands as a profoundly pervasive and disabling condition, impacting over 300 million individuals globally, and imposes significant personal and economic challenges [Citation1–3]. The prevalence of recurrent depressive episodes among those diagnosed with MDD contributes to heightened suffering and escalates the risk of suicidal tendencies and mortality [Citation4,Citation5]. Notably, depression has a strong correlation with suicide, which ranks among the top three causes of death in individuals aged 15 to 44 years [Citation6] with around 800,000 suicides reported annually [Citation7,Citation8].
Concerns regarding early onset suicidal ideation, highlighted in FDA registration trials, are predominantly associated with children and adolescents, not extending to older patient populations. It’s critical to note that such early suicidal thinking has not been conclusively linked to an increased risk of completed suicide [Citation9]. The traditional treatment landscape for depression encompasses a variety of pharmacotherapies beyond selective Serotonin reuptake inhibitors (SSRIs), targeting multiple monoamine pathways. However, it’s important to acknowledge that the pathophysiology of depression extends beyond monoaminergic dysregulation, encompassing roles for neurotrophic factors like brain-derived neurotrophic factor (BDNF) and neuropeptides as well. Despite the initial action of antidepressants in blocking monoamine reuptake and boosting extracellular monoamine levels, these medications often require several weeks to months before their biological and therapeutic effects become apparent [Citation10,Citation11]. Approximately one-third of patients with depression respond to their first antidepressant, another third achieve remission after multiple trials, and the rest remain unresponsive, indicating treatment-resistant depression [Citation12,Citation13]. This scenario has fueled the pursuit of rapid-acting pharmacological interventions that employ different mechanisms, aiming to provide immediate relief for those inadequately served by existing treatments.
A crucial development in comprehending the neurobiology of depression has been the recognition of ketamine, an N-methyl-D-aspartate receptor (NMDAR) antagonist, for its rapid antidepressant properties. It has been observed that a single subanesthetic dose of intravenous (R,S)-ketamine can prompt a quick antidepressant response in individuals, including those with treatment-resistant depression, though the effects may diminish over time. Additionally, it’s important to acknowledge that not all individuals experience complete remission with ketamine; there exists a spectrum of responses, including partial and non-responders [Citation14–16]. It is important to note that (R,S)-ketamine is a racemic mixture, composed of equal parts of (R)-ketamine (arketamine) and (S)-ketamine. The enantiomer (S)-ketamine, also known as esketamine, along with (R)-ketamine, exhibits antidepressant properties. The clinical use of (R,S)-ketamine faces challenges due to its potential for recreational misuse and psychotomimetic effects, leading to a need for cautious prescription, especially for individuals with a history of substance abuse [Citation17,Citation18]. In 2019, esketamine, the (S)-enantiomer of ketamine, received FDA approval as a nasal spray specifically for treatment-resistant depression in both the United States and Europe [Citation19]. It is noteworthy, however, that while esketamine is the ‘officially’ approved form, ketamine itself, an FDA-approved medication for anesthesia, has been increasingly utilized off-label for depression treatment. This off-label use encompasses various forms, including intravenous and oral administration, in numerous proprietary and outpatient clinics. Despite this broader application, the use of esketamine remains under careful regulation due to potential adverse effects, such as hyperhidrosis, dissociation, urinary discomfort, and suicidal thoughts [Citation20–22]. Notably, (R)-ketamine has been observed to have more potent and longer-lasting antidepressant effects compared to esketamine in animal studies [Citation23,Citation24].
Recent research has increasingly highlighted the role of BDNF and its receptor, (neurotrophic receptor tyrosine kinase 2) TrkB, in the pathophysiology of mood disorders, where BDNF’s critical function includes mediating activity-dependent neuronal plasticity, enhancing neurite outgrowth, and stabilizing synapses through regulated signaling pathways [Citation25–29]. Three primary hypotheses have emerged to elucidate ketamine’s antidepressant mechanism, all highlighting the pivotal role of BDNF and/or TrkB signaling. The disinhibition hypothesis suggests ketamine blocks NMDA receptors on inhibitory neurons, leading to increased extracellular glutamate, activation of AMPA receptors, and subsequent BDNF release [Citation30,Citation31]. Another theory posits that ketamine’s blockade of NMDA receptors prevents calcium entry, thereby inducing a swift rise in BDNF protein synthesis [Citation32,Citation33]. A third perspective proposes that ketamine directly binds to the TrkB receptor, with affinity comparable to its NMDA receptor affinity [Citation34].
In particular, neuropeptides like Neuropeptide Y (NPY) play a pivotal role in mood regulation, influencing emotional processing and stress response systems within the brain, thereby presenting a promising target for therapeutic intervention in depressive disorders. NPY is a 36 amino acid peptide abundantly present in the brain and peripheral tissues, with significant concentrations in areas crucial for emotional regulation, such as the amygdala, nucleus accumbens, hypothalamic nuclei, cortex, and hippocampus. NPY is involved in various physiological processes, including modulation of feeding, blood pressure, learning and memory, circadian rhythms, neuroprotection, and stress and mood regulation [Citation35–38]. The relevance of NPY in depressive disorders has been highlighted in studies, such as those using Flinders Sensitive Line rats, a genetic model for depression, which showed reduced hippocampal NPY1 receptor (NPY1R) mRNA levels compared to controls [Citation39]. NPY’s potential in combating depression was also demonstrated in the forced swimming model, likely through NPY1R-mediated effects [Citation40,Citation41]. Moreover, postmortem studies have identified reduced levels of NPY expression in the hippocampal regions of individuals with depression, emphasizing a potential link between NPY dysregulation and the pathophysiology of mood disorders [Citation42]. Additionally, NPY treatment has been associated with increases in BDNF levels in models of neurodegeneration-induced hippocampal disorders, further highlighting its importance in the context of depression [Citation43–45]. This body of work suggests a dysregulation of the NPY-BDNF system in depressive states and suggests the upregulation of NPY-BDNF and NPY1R-TrkB signaling as promising avenues for antidepressant therapies.
These insights into BDNF-TrkB signaling mechanisms highlight the rationale for exploring ketamine, a rapid-acting antidepressant that modulates these neurotrophic pathways, in conjunction with NPY agonists, to potentially amplify neurogenesis and synaptic plasticity, offering a novel therapeutic strategy for depression beyond traditional monoamine-based approaches. This investigation specifically aims to assess the synergistic effects of combining an NPY1R agonist with Ketamine, focusing on modulating neurotrophic mechanisms within the ventral hippocampus – a region critically implicated in mood regulation. We hypothesize that this combination could enhance neurogenesis and exert rapid antidepressant effects, potentially overcoming the shortcomings of traditional SSRIs and offering a novel therapeutic avenue for patients with treatment-resistant depression. This study sets out to not only elucidate the underlying neurobiological mechanisms facilitated by this drug combination but also to contribute to the development of more effective and faster-acting antidepressant therapies.
2. Materials and methods
2.1. Animal housing and care
In our study, male Sprague-Dawley rats, obtained from CRIFFA in Barcelona, spanning a weight range of 200–250 grams and aged between 6–8 weeks, were accommodated with free access to both food and water. These animals were maintained under a precise 12-hour cycle of light and darkness, with environmental parameters such as temperature (22 ± 2°C) and relative humidity (55–60%) rigorously regulated. The design and execution of all experimental procedures involving these animals strictly complied with the EU Directive 2010/63/EU and Spanish legal requirements (Real Decreto 53/2013). The ethics committee of the University of Málaga, Spain (CEUMA 45–2022-A), provided full approval for the comprehensive care, treatment, and management protocols of the animals used in this research.
2.2. Preparation of drugs
For the experimental interventions, we sourced the NPY1R receptor agonist [Leu31, Pro34]NPY and the NPY1R antagonist BIBO3304 from Tocris Bioscience, Bristol, UK, and the TrkB antagonist (ANA-12, 5.06304) from Sigma Aldrich, St. Louis, MO, U.S.A. Ketamine was acquired from the University of Malaga’s animal facility. The methods for intranasal (i.n.) infusion and intracerebroventricular (i.c.v.) administration of these substances are elaborated in the supplementary materials. Solutions for i.c.v. administration were freshly prepared, with peptides dissolved in artificial cerebrospinal fluid (aCSF) to specific mM concentrations, while those for i.n. infusion were prepared in distilled water. We do not observe any nasal lesion/irritation in animals during intranasal delivery of the tested compounds.
2.3. Assessment of depression-like behavior
The assessment of depression-like behavior was conducted using the Forced Swim Test (FST) in naive animals, a well-established model for the preliminary screening of antidepressant potential, during two separate sessions [Citation46–48].
Behavioral and neurochemical analyzes were carried out on distinct groups of rats, with behavioral testing scheduled between 09:00 and 14:00 hours. Prior to testing, animals underwent a habituation process in the experimental room, under specific lighting conditions, and were then randomly divided into experimental groups for treatment. Icv or intranasal treatments were administered 24 hours before testing, with doses determined based on previously established protocols and effectiveness assessed through dose-response curves [Citation49–53]. We utilized the open field test in a separate cohort of animals to ensure that there were no locomotor effects following the treatments. Rats were individually placed and allowed to freely explore the open field over a 5 min period, scoring the distance moved, using the video tracking software Smart 2.5 (Panlab, SL). For the forced swim test, the total duration of floating (immobility) and swimming periods were scored during the 5 min test and analyzed using the Raton Time 1.0 software (Fixma S.L., Valencia, Spain). Floating in the water without struggling and only making movements necessary to keep its head above the water was regarded as immobility. Swimming was scored when they actively swam around the cylinder. Following swimming sessions, the rats were removed from the tank, carefully dried in heated cages, and then returned to their home cages. Behavioral experiments were carried out by observers blinded to all experimental conditions.
2.4. Analysis of co-localization through in situ proximity ligation assay
A different cohort of rats were randomly allocated into five experimental groups: (1) Control: distilled water; (2) NPY1R agonist-treated group receiving the NPY1R agonist [Leu31- Pro34]NPY (68 µg); (3) Ketamine-treated group receiving the Ketamine (10 mg/Kg); (4) NPY1R+Ketamine: group administered with both substances; (5) NPY1R+Ketamine+ANA-12: group treated with [Leu31- Pro34]NPY, Ketamine and the TrkB antagonist (ANA-12; 0,5 mg/kg µg). The doses indicated are based on previously published protocols [Citation49,Citation50]. Treatments were administered 24 hours before the experiments.
To explore GALR2-Y1R co-localization in the ventral dentate gyrus, the in situ proximity ligation assay (in situ PLA) method was utilized, specifically employing NaveniFlex Tissue GR Atto 647N, Navinci, Sweden. The procedure was performed on free-floating sections according to established protocols [Citation53,Citation54]. Brain slices were first conditioned with blocking buffer for one hour at 37°C inside a pre-warmed humidity chamber, followed by overnight incubation at 4°C with the primary antibodies at optimal concentrations. Utilized antibodies included goat anti-NPYY1R (1:200, sc -21,992, Santa Cruz Biotechnology INC, CA) and rabbit anti-TrkB (1:200, Sigma Aldrich, ZRB1281) and. Subsequently, the samples were rinsed three times, and a mixture of Navenibody goat and rabbit was applied for an hour at 37°C. Unbound probes were removed through washing, and Enzymes A and B were sequentially applied in a humidity chamber at 37°C for 60 and 30 minutes, respectively. After washing away excess connector oligonucleotides, the samples were treated with the rolling circle detection mixture (Enzyme C, Tex615) and incubated at 37°C for 90 minutes. The sections were then mounted and sealed with fluorescent mounting medium containing DAPI (Abcam, ab104139), stored at − 20°C, and analyzed later through confocal microscopy as previously outlined [Citation50,Citation55,Citation56].
2.5. Evaluation of hippocampal proliferation
Post-treatment described above, rats were administered two intraperitoneal (i.p.) injections of 5′-Bromo-2′-deoxyuridine (BrdU, Sigma, St. Louis, MO, U.S.A.) dissolved in a sterile 0.9% NaCl solution at a concentration of 15 mg/mL, at a dosage of 50 mg/kg body weight every two hours starting at 9:00 AM. BrdU procedure [Citation57,Citation58] is based on previously published protocols. Twenty-four hours after the treatments, rats were deeply anesthetized with pentobarbital (Mebumal, 100 mg/kg, i.p.) and transcardially perfused with 4% PFA (para-formaldehyde (wt./vol, Sigma Aldrich, St. Louis, MI, U.S.A.)). Using a Cryostat (HM550, Microm International, Walldorf, Germany) the brains were coronally sliced (30 μm-thick) through the ventral hippocampus (anterior in primates) (−5.20 to − 6.72 Bregma [Citation59]).
2.5.1. Immunohistochemistry
For the immunohistochemistry process, brain sections underwent a series of detailed preparation steps. Initially, they were subjected to a 90-minute incubation at 65°C in a saline sodium citrate buffer solution (pH 6; 10 nM sodium citrate) to facilitate antigen retrieval. This was followed by a 30-minute exposure to 0.6% hydrogen peroxide (H2O2) aimed at eliminating endogenous peroxidase activity. Subsequently, the sections were treated for 30 minutes in 2 M hydrochloric acid (HCl) to denature the DNA, before being neutralized in a 0.1 M sodium borate solution (pH 8). The sections were then incubated overnight at 4°C with a primary antibody specifically targeting BrdU (Abcam, ab152095, 1:1000), diluted in 2.5% donkey serum. After thorough washing in PBS, the sections were exposed to a biotinylated anti-rabbit IgG secondary antibody (1/200, Vector Laboratories) for 90 minutes, followed by amplification with ExtrAvidin peroxidase (Sigma, St. Louis, MO, U.S.A.) at a 1:100 dilution in darkness at room temperature for an hour. The immunolabeling was revealed using 0.05% diaminobenzidine (DAB; Sigma) and 0.03% H2O2 in PBS. Following several washes, the sections were mounted on gelatin-coated slides, dehydrated through a series of graded alcohols, and cover-slipped using DePeX mounting medium (Merck Life Science SLU, Darmstadt, Germany). The BrdU-labeled cells were quantified employing the optical fractionator technique in unbiased stereological microscopy (Olympus B×51Microscope, Olympus, Denmark), as described in earlier studies and further detailed in the supplementary material [Citation50,Citation60,Citation61].
2.5.2. Double immunofluorescence
For double immunofluorescence, the brain sections were first blocked and permeabilized according to well-established protocols, then incubated with primary antibodies targeting BrdU and DCX [Citation50,Citation53,Citation61–63]. This process began with an hour-long incubation in both blocking solution (5% goat serum) and permeabilization solution (0.3% Triton X-100 in PBS). Following this, the sections were incubated with a combination of rabbit anti-BrdU (Abcam, ab152095, 1:1000) and mouse anti-DCX (C-18, Santa Cruz, 1:500) primary antibodies for 24 hours at 4°C. Appropriate secondary antibodies, Donkey anti-mouse AlexaFluor 488 (Abcam, ab150105, 1:200) and Donkey anti-rabbit AlexaFluor 647 (Abcam, ab150075, 1:200), were then applied. After mounting on slides with a fluorescent mounting medium containing 4,’6-diamidino-2-phenylindole (DAPI) for nuclear detection (Abcam, ab104139), BRDU/DCX-double-stained cells in the dentate gyrus (DG) were quantified using z-scan confocal microscopy (Leica Stellaris 8) at 40X magnification. The entire septo-temporal axis of the hippocampus was assessed, examining at least four representative sections per animal, spaced 150 μm apart. Analysis was conducted in sequential scanning mode to prevent cross-bleeding between fluorescence channels, ensuring accurate colocalization by examining multiple optical planes of each cell on the z-axis. Z-stacks were created at 0.85 μm intervals through the 30 μm section to confirm precise double-labeling of BrdU-IR cells [Citation61,Citation64].
2.6. Evaluation of brain-derived neurotrophic factor (BDNF) induction in the ventral dentate gyrus
To assess the induction of Brain-Derived Neurotrophic Factor (BDNF) within the ventral dentate gyrus, a separate collection of free-floating brain sections underwent an antigen retrieval process. This involved a 90-minute incubation at 65°C in a saline sodium citrate buffer solution (pH 6; 10 nM sodium citrate), followed by a subsequent 30-minute exposure to 0.6% hydrogen peroxide (H2O2). After these preparatory steps, the brain slices were incubated at ambient temperature with a primary antibody specifically targeting BDNF (rabbit anti-BDNF, Chemicon, Sigma-Aldrich, AB1534SP, 1:500) diluted in 2.5% donkey serum. Following thorough washes in PBS, the sections were further incubated with a biotinylated anti-rabbit IgG secondary antibody (1:200, B8895, Sigma, St. Louis, MO, U.S.A.) for 90 minutes, and then treated with ExtrAvidin peroxidase (1:100, Sigma, St. Louis, MO, U.S.A.) in complete darkness for an additional hour. The visualization of BDNF-expressing cells was achieved through the application of 0.05% diaminobenzidine (DAB) and 0.03% H2O2 in PBS. The treated sections were then mounted on gelatin-coated slides, cover-slipped using DePeX mounting medium (Merck Life Science SLU, Darmstadt, Germany), and the presence of BDNF-positive cells was quantified employing the optical fractionator technique through unbiased stereological microscopy (Olympus B×51Microscope, Olympus, Denmark).
2.7. Statistical analysis
Data were exhibited as mean ± SEM, with the number of samples denoted in the figure legends. GraphPad PRISM 8.0 (GraphPad Software, La Jolla, CA, U.S.A.) was harnessed for data examination. One-way ANOVA was conducted followed by the Newman-Keuls posttest for comparative analysis, or Student’s unpaired t-test where necessary. Significance thresholds were defined as *p < 0.05, **p < 0.01, ***p < 0.001.
3. Results
3.1. Enhanced antidepressant-like response induced by NPY1R agonists and ketamine in the forced swim test
Combined Administration of NPY1R Agonist via Intracerebroventricular (icv) and Intranasal (i.n.) routes and Ketamine:
3.1.1. Icv administration of NPY1R agonist
In evaluating the antidepressant-like effects in the Forced Swim Test (FST), we analyzed the impact of administering a combination of icv NPY1R agonist and Ketamine. This analysis focused on the durations of immobility and swimming exhibited by the subjects. Notably, the concurrent administration of NPY1R agonist and Ketamine significantly reduced the time spent immobile (one-way ANOVA, F4,25 = 10.57, p < 0.001; ) in comparison to subjects receiving Ketamine alone (Newman-Keuls post-hoc test: p < 0.05), artificial cerebrospinal fluid (aCSF) (Newman-Keuls post-hoc test: p < 0.001), and NPY1R agonist in isolation (Newman-Keuls post-hoc test: p < 0.001). Additionally, this combination therapy significantly increased swimming activity (one-way ANOVA, F4,25 = 11.65, p < 0.001; ) relative to the same comparison groups: Ketamine alone (Newman-Keuls post-hoc test: p < 0.05), aCSF (Newman-Keuls post-hoc test: p < 0.001), and NPY1R agonist alone (Newman-Keuls post-hoc test: p < 0.001). The introduction of the NPY1R antagonist BIBP3226 reversed these observed effects, indicating its efficacy in modulating both immobility and swimming behaviors as demonstrated through post-hoc testing (p < 0.05 for both behaviors). visually depict the significant reductions in immobility and enhancements in swimming behaviors consequent to the treatments, showcasing the effectiveness of Ketamine and NPY1R agonist coadministration. No differences were observed between groups in locomotor parameters (Supplementary table S1). Statistical values for the forced swim test are presented in Supplementary Table S2.
Figure 1. Antidepressant-like actions of NPY1R agonist and ketamine co-administration in the forced swimming test (FST).
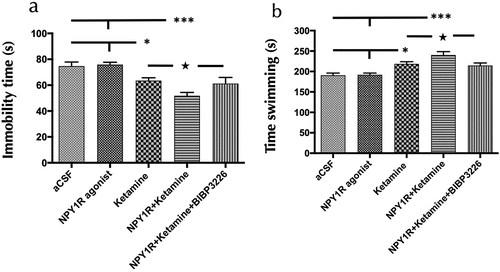
3.1.2. Intranasal administration of NPY1R agonist
Subsequently, the FST was administered following intranasal (i.n.) delivery of the NPY1R agonist in conjunction with Ketamine. The test, conducted 24 hours post-administration, aimed to identify depressive-like behaviors through measures of immobility and swimming over a 5-minute testing phase. This intranasal coadministration led to a pronounced reduction in immobility time (one-way ANOVA, F5,30 = 9.48, p < 0.001, ) when compared to control animals (Newman-Keuls post-hoc test: p < 0.001; ), NPY1R agonist alone (Newman-Keuls post-hoc test: p < 0.001; ), and Ketamine alone (Newman-Keuls post-hoc test: p < 0.05; ). Furthermore, there was a notable increase in swimming behavior following the combined treatment compared to controls (Newman-Keuls post-hoc test: p < 0.001; ), NPY1R agonist alone (Newman-Keuls post-hoc test: p < 0.001; ), and Ketamine alone (Newman-Keuls post-hoc test: p < 0.05; ). The addition of NPY1R antagonist BIBP3226 negated the combined treatment’s synergistic effects on both immobility and swimming (Newman-Keuls post-hoc test: p < 0.01 and p < 0.05, respectively; ), highlighting the NPY1R’s role in this context. Importantly, the involvement of Brain-Derived Neurotrophic Factor (BDNF) in these effects was confirmed through the administration of the TrkB antagonist ANA-12, which obstructed the synergistic effects on immobility and swimming induced by the coadministration of NPY1R agonist and Ketamine (Newman-Keuls post-hoc test: p < 0.001 for both; ). As previously noted, Ketamine administration alone was associated with decreased immobility and increased swimming compared to control and NPY1R agonist-treated animals (Newman-Keuls post-hoc test: p < 0.05 for both comparisons; ). However, NPY1R agonist administration in isolation showed no significant effects on FST outcomes compared with the control group, as depicted in . No differences were observed between groups in locomotor parameters (Supplementary table S3). Statistical values are presented in Supplementary Table S4.
Figure 2. Impact of intranasal NPY1R agonist and ketamine Co-administration on FST behavioral outcomes.
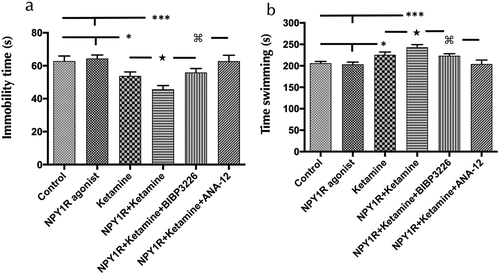
For subsequent experiments, we opted for the intranasal delivery of the NPY1R agonist, with the goal of enhancing the translational potential of our findings.
3.2. Coactivation of NPY1R and TrkB enhances NPY1R/TrkB heteroreceptor complexes
To elucidate the cellular mechanisms underlying the observed in vivo effects at the receptor level, we utilized the in situ proximity ligation assay (PLA) within the ventral hippocampal dentate gyrus (DG). This technique enabled us to examine the formation of NPY1R/TrkB heteroreceptor complexes following the coadministration of an NPY1R agonist and Ketamine. The PLA revealed positive red clusters within the subgranular and polymorphic layers of the ventral DG. A significant uptick in the density of these clusters was quantitatively confirmed after the combined treatment of NPY1R agonist and Ketamine compared to the control group (one-way ANOVA, F4, 20 = 20.71, p < 0.001), with subsequent post-hoc analyzes underscoring notable differences (p < 0.001 against both control and NPY1R agonist [Leu31, Pro34]NPY alone; p < 0.01 versus Ketamine alone) as illustrated in . The blockade effect of the TrkB antagonist ANA-12 (p < 0.001 in post-hoc analysis) pinpointed TrkB’s crucial role in this process. visually presents the PLA-positive red clusters in the ventral DG, with subpanels detailing the cluster density across various treatment conditions.
Figure 3. Visualization of NPY1R/TrkB heteroreceptor complex formation in the ventral hippocampal DG via proximity ligation assay.
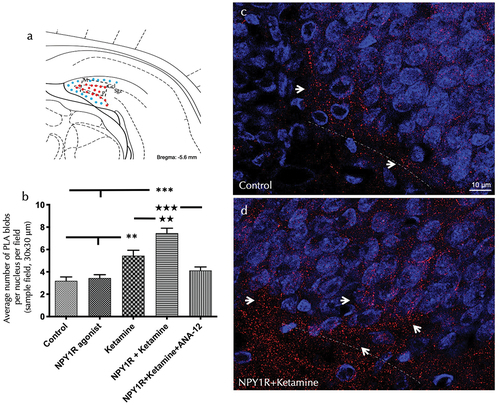
Further PLA quantification highlighted an enhanced density of PLA-positive red clusters following Ketamine administration alone, as compared to both control and NPY1R agonist treatments (Newman-Keuls post-hoc test: p < 0.01 for both comparisons), as shown in . Conversely, administration of the NPY1R agonist alone did not significantly affect the density of PLA-positive red clusters, as depicted in . Statistical values are presented in Supplementary Table S5.
3.3. NPY1R agonist and ketamine combination promotes cell proliferation of newborn neurons in the ventral hippocampus
Exploring the influence of the NPY1R/TrkB interaction, we investigated the impact of NPY1R agonist and Ketamine co-administration on cell proliferation in the adult ventral hippocampus, utilizing the thymidine analogue 5-Bromo-2’-deoxyuridine (BrdU) as indicated in . The co-administration markedly elevated the count of BrdU-immunoreactive (BrdU-IR) profiles within the subgranular zone (SGZ) of the dentate gyrus, surpassing that of the control, NPY1R agonist alone, and Ketamine alone groups (one-way ANOVA, F4,15 = 11.68, p < 0.001), with post-hoc assessments revealing significant disparities (p < 0.001 against control and NPY1R agonist; p < 0.05 against Ketamine) as detailed in . The introduction of ANA-12 effectively inhibited this proliferative effect (p < 0.001 in post-hoc analysis), indicating TrkB’s involvement in the synergistic action of NPY1R agonist-Ketamine on cell proliferation. The figure illustrates the proliferation surge in BrdU-positive cells within the SGZ, emphasizing the heightened proliferation rate 24 hours following the treatments.
Figure 4. Enhancing neurogenesis in the ventral dentate gyrus through intranasal Co-administration of NPY1R agonist and ketamine.
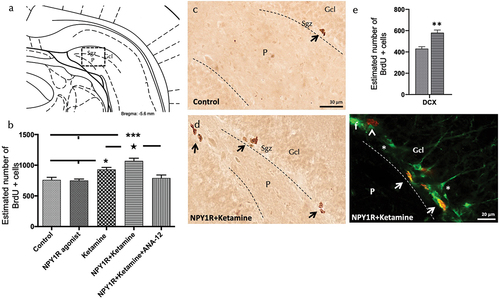
Additionally, the sole administration of Ketamine at a dose of 10 mg/kg prompted an increase in BrdU-positive cell numbers within the SGZ of the ventral hippocampus () in comparison to control and NPY1R-treated groups (Newman-Keuls post-hoc test: p < 0.01). In contrast, the NPY1R agonist, when administered intranasally on its own, did not significantly alter the count of BrdU-IR profiles, aligning with control group findings as displayed in . Statistical values are presented in Supplementary Table S5.
3.4. Identification of cellular types affected by increased proliferation after NPYY1R agonist and ketamine administration
Furthermore, we investigated the specific cellular types influenced by the coadministration of NPY1R agonist and Ketamine by quantifying BrdU-labeled cells that also expressed doublecortin (DCX), a marker of neuroblasts (). The results demonstrated a significant enhancement in the proliferation of PCNA+/DCX+ cells following the infusion of NPY1R agonist and administration of Ketamine (t-test, t = 4.67, df = 6; Control: 430 ± 19; NPY1R+Ketamine: 580 ± 26; p < 0.01) as depicted in , confirming the stimulated proliferation of neuroblasts.
3.5. Association of increased neuroblasts with enhanced brain-derived neurotrophic factor (BDNF) through coactivation of NPY1R agonist and ketamine
In an effort to delineate the mechanisms underpinning the observed increase in neuronal proliferation, we assessed the expression of Brain-Derived Neurotrophic Factor (BDNF) within the ventral hippocampal dentate gyrus (DG) subsequent to the administration of either the NPY1R agonist, Ketamine, or their combination. BDNF-positive cells were identified predominantly in the granular layer of the ventral hippocampus and to a lesser extent within the polymorphic layer (P) of the ventral DG (). Stereological quantification revealed a significant elevation in BDNF-positive cells following the combined treatment with NPY1R agonist and Ketamine compared to the control group (one-way ANOVA, F4, 15 = 12.88, p < 0.001, Newman-Keuls post-hoc test: p < 0.001), as well as compared to the administration of NPY1R agonist alone (Newman-Keuls post-hoc test: p < 0.001) or Ketamine alone (Newman-Keuls post-hoc test: p < 0.05) as shown in . Consistent with previous findings, the application of the TrkB antagonist ANA-12 completely nullified the increase induced by the combined treatment (Newman-Keuls post-hoc test: p < 0.001) in , underscoring the integral role of TrkB in mediating this effect.
Figure 5. Enhancing BDNF expression in the ventral dentate gyrus through Co-administration of NPY1R agonist and ketamine.
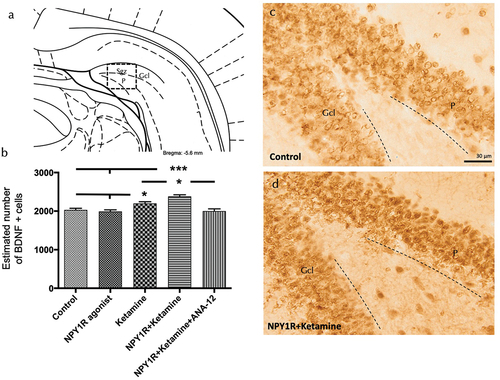
Moreover, Ketamine treatment alone was observed to augment the number of BDNF-positive cells within the granular zone of the ventral hippocampus () relative to the control and NPY1R agonist-treated groups (Newman-Keuls post-hoc test: p < 0.05). On the other hand, the NPY1R agonist infusion, when administered independently, did not exhibit a significant effect on the quantity of BDNF-positive cells in the ventral DG. Statistical values are presented in Supplementary Table S5.
4. Discussion
4.1. Overview of findings and comparison with previous studies
Our findings significantly enhance the current understanding of the neurobiological mechanisms underlying antidepressant effects, particularly regarding the interplay between NPY1R agonists and Ketamine. While previous studies have laid the groundwork for the rapid-acting antidepressant properties of Ketamine and have begun to explore the potential role of NPY in mood regulation [Citation14–16,Citation35–38], our study advances this knowledge by demonstrating a potential synergistic effect when combining these two treatment modalities. This synergy suggests a promising avenue toward enhancing antidepressant responses, especially in patients with treatment-resistant depression, a persistent clinical challenge in modern psychiatry [Citation12,Citation13]. Furthermore, in contrast to existing literature documenting side effects and safety concerns associated with Ketamine administration [Citation17,Citation18,Citation20–22] our study highlights the necessity for future research focused on optimized treatment regimes that minimize these risks while maximizing therapeutic benefits. Ultimately, by exploring the neurotrophic mechanisms within the ventral hippocampus implicated by coadministration of an NPY1R agonist and Ketamine, we lay a basis for the development of more effective and rapid antidepressant therapies, opening new pathways to overcome the limitations of traditional SSRIs [Citation10,Citation11,Citation25–29].
We observed a sustained antidepressant-like response induced by the intracerebroventricular (icv) administration of an NPY1R agonist in conjunction with Ketamine. This aligns with previous studies that document the efficacy of sub-anesthetic doses of Ketamine in reducing immobility time in the Forced Swim Test (FST) in rats [Citation52,Citation65,Citation66]. The observed enhancement in swimming behavior during the rat Forced Swim Test highlights the potential of swimming time as a metric to gain insights into the mechanisms driving antidepressant-like actions since is considered indicative of an active coping strategy, potentially mediated by serotonergic mechanisms. Notably, the reduction in immobility coupled with increased swimming activity may reflect serotonergic involvement, similar to the effects produced by selective serotonin reuptake inhibitors. This suggests intricate neurochemical interplays in the modulation of depressive-like behaviors [Citation67].
Our findings demonstrate the capability of our FST methodology to detect the antidepressant effects of Ketamine, supported by literature indicating amplified effects when combined with agents like Lithium or LY341495 [Citation68,Citation69], and similarly, NPY1R agonist with Galanin [Citation53,Citation63].
4.2. The significance of non-invasive delivery methods
Given the potential limitations associated with icv administration, our exploration into the noninvasive intranasal delivery of NPY1R agonist presents a promising alternative. While intranasal delivery offers a targeted approach to CNS penetration, minimizing systemic exposure, it is important to acknowledge that systemic administration of NPY1R agonists requires careful consideration of their ability to cross the BBB and the potential for systemic adverse effects. Our focus on intranasal delivery was guided by the objective to leverage its direct CNS access while mitigating broader systemic distribution that could arise from other administration routes, thus aiming to reduce the likelihood of systemic side effects associated with NPY1R agonist use. Intranasal delivery presents an effective method for the direct transport of protein therapeutics into the CNS, adeptly circumventing the blood-brain barrier via two principal pathways. The initial pathway involves the absorption of the drug through the nasal epithelium into the submucosa, from where it can directly access the cerebrospinal fluid (CSF) or penetrate the CNS through volume transmission, facilitated by extracellular diffusion along perineuronal channels [Citation70,Citation71]. Alternatively, the drug may be taken up by primary olfactory neurons and transported intracellularly to the olfactory bulb, subsequently dispersing to further brain regions via neuronal intracellular mechanisms and volume transmission [Citation72]. This method not only validates our findings by showcasing an enhanced antidepressant-like response but also aligns with the efficiency of intranasal delivery of NPY1R and GALR2 agonists [Citation73]. Future investigations into the pharmacokinetics and safety profile of NPY1R agonists, particularly focusing on their metabolism, distribution, and excretion, are essential to fully elucidate their therapeutic potential and applicability for clinical use. Such studies will provide valuable insights into optimizing dosing strategies and minimizing risks, thereby facilitating the transition from preclinical research to clinical application. The role of BDNF highlighted by the blockade of effects by the TrkB antagonist ANA-12 [Citation51,Citation74] highlights the mechanistic foundation of these observations. Thus, the antidepressant-like effects of the Ketamine and NPY1R agonist were linked to neurotrophic mechanisms within the ventral hippocampus [Citation75,Citation76].
Acknowledging the potential need for chronic or intermittent administration of Ketamine and NPY1R agonists to sustain antidepressant effects highlights a pivotal area for future research, given the current absence of data supporting long-term efficacy without ongoing treatment strategies.
4.3. Cellular and molecular mechanisms underpinning the antidepressant-like effects
Our study delves into the cellular effects of NPY1R agonist and Ketamine coadministration, particularly focusing on the increase in NPY1R/TrkB heteroreceptor complexes in the ventral hippocampus. This suggests a synergistic interaction at the molecular level, potentially serving as a biomarker for drug target engagement. However, we acknowledge the necessity of exercising caution in the interpretation of the underlying mechanisms observed, particularly regarding the involvement of NPY1R-TrkB heteroreceptor complexes and downstream signaling pathways. While our data present compelling evidence, it is imperative to consider alternative interpretations and the inherent limitations of our findings. The absence of direct additional evidence necessitates a conservative approach to concluding definitive causal relationships. To definitively demonstrate the structural formation and functional interdependence of NPY1R-TrkB heteroreceptor complexes, more advanced experimental approaches are required. Techniques such as Co-Immunoprecipitation (CoIP), Fluorescence Resonance Energy Transfer (FRET), and Bioluminescence Resonance Energy Transfer (BRET) could offer direct evidence of these complexes. This endeavor aligns with our previous discoveries of GALR2/NPY1R heteroreceptor complexes in limbic brain regions, further supporting the significance of our approach [Citation50,Citation53,Citation56,Citation60–63]. By committing to these experimental validations, we aim to address the current limitations and enrich the robustness of our findings.
Further, the observed increase in cell proliferation and the specific enhancement in neuroblast cells underline the role of these complexes in mediating antidepressant effects, which is corroborated by the involvement of BDNF and its signaling through TrkB. Following the delivery of NPY1R agonist and Ketamine, an increased cell proliferation in the ventral dentate gyrus (DG) of the hippocampus was observed using the BrdU marker. We then sought to determine whether a specific cell subpopulation was more affected by NPY1R agonist and Ketamine coadministration. For this, out of the overall BrdU + cells quantified, we identified and quantified the number of cells co-expressing the neuroblast marker BrdU (BrdU+/DCX+ cells). This increase in cell proliferation supports the action of NPY1R-TrkB heteroreceptor complexes on neuroblasts [Citation77]. Similar to the role of ketamine, standard antidepressant drugs also engage adult hippocampal neurogenesis, contributing to their therapeutic effects. These medications have been shown to increase the density of newborn granule cells, stimulate cell proliferation, and promote the maturation of these cells within the ventral hippocampus. This process emphasizes a common neurobiological mechanism underlying the efficacy of both rapid-acting and traditional antidepressants, highlighting the critical role of neurogenesis in mediating antidepressant responses across different therapeutic modalities [Citation78–80].
4.4. The crucial role of BDNF in mood disorders and antidepressant effects
Finally, the significant correlation between BDNF levels, mood disorders, and the efficacy of antidepressant treatments emphasizes the fundamental role of neurotrophic factors. The evidence suggesting that TrkB in progenitor cells targets BDNF from dentate gyrus cells [Citation81–83] further highlights the intricate interplay between neurogenesis and mood regulation. Reduced levels of BDNF have been observed in postmortem samples from the brains of depressed patients, particularly within the hippocampus [Citation84,Citation85]. BDNF levels have also consistently been found to be reduced in brain samples of people who died as a result of suicide [Citation86,Citation87]. Conversely, standard antidepressant treatment has been shown to increase BDNF expression in human postmortem brain samples of depressed patients [Citation88]. BDNF signaling through TrkB is essential for the behavioral effects of standard antidepressants, with several studies investigating the specific brain regions and cell types where BDNF-TrkB signaling mediates these effects [Citation89,Citation90]. Notably, Ketamine enhances BDNF-TrkB signaling through three distinct mechanisms: by blocking NMDA receptors on inhibitory neurons, leading to increased AMPA receptor activation and BDNF release; inhibiting NMDA receptor-mediated calcium entry to boost BDNF synthesis; and possibly directly interacting with the TrkB receptor, indicating its versatile impact on neuroplasticity pathways [Citation30–34]. Evidence suggests that TrkB in progenitor cells is the target of BDNF released from dentate gyrus cells. Deleting BDNF from dentate gyrus cells or TrkB from progenitor cells of dentate granule neurons inhibits the effects of antidepressants on behavioral paradigms and induced neurogenesis [Citation91,Citation92].
4.5. Rationale for employing naive rat models in mood disorder research
The decision to utilize naive rat models in our investigation into the pathophysiological underpinnings of mood disorders was made with careful consideration of both the scope and the foundational nature of our study. Naive models offer a unique vantage point for dissecting the neurobiological and behavioral aspects of mood regulation, devoid of the confounding variables introduced by preexisting conditions or induced models of depression. This approach not only aids in the clear elucidation of the primary mechanisms that govern mood dynamics but also provides a critical baseline for the evaluation of prophylactic and therapeutic interventions [Citation40,Citation93,Citation94]. Furthermore, by establishing a robust baseline understanding of mood regulation mechanisms, our study lays the groundwork for subsequent research utilizing more complex models of depression. It is our contention that this methodological choice amplifies the potential for discovering novel insights into mood disorders, thereby enriching the field’s understanding and paving the way for the development of more targeted and effective therapeutic strategies in future investigations.
4.6. Limitations and directions for future research
We acknowledge the inherent limitation of relying on a single behavioral assay in neuropsychiatric research. While the FST is a widely accepted and validated model for assessing antidepressant-like activity, we recognize that the complexity of depressive disorders cannot be fully encapsulated by any single behavioral test. To enhance the robustness of our conclusions, future studies should incorporate a diverse array of behavioral tests, such as the Tail Suspension Test, the Novelty-Suppressed Feeding Test, and the Sucrose Preference Test, among others, to provide a more comprehensive evaluation of antidepressant efficacy. These additional tests would allow for the assessment of different aspects of depression-like behaviors, including despair, anhedonia, and anxiety-related responses. Our commitment to implementing these additional behavioral assays reflects our dedication to providing a multifaceted evaluation of the therapeutic potential of the treatments under investigation. Through this expanded approach, we aim to significantly enhance the robustness and interpretative power of our conclusions.
In light of our preclinical observations, it is crucial to contemplate the translational potential of these findings to the clinical treatment of depression in humans. While the outcomes from NPY1R agonists and Ketamine demonstrate significant therapeutic promise, addressing critical factors such as safety, feasibility, and potential challenges in clinical implementation is imperative prior to their application in therapeutic practices. Extrapolating these results to human patients will necessitate rigorous clinical trials to assess not only efficacy but also safety and tolerability of such interventions. Moreover, it is vital to consider optimal dosing, administration routes, and the possibility of side effects or interactions with other treatments. Addressing these factors will enable a more comprehensive evaluation of the clinical potential of our therapeutic strategies and pave the way for effective clinical implementation, thereby contributing to the advancement of treatment options for patients with depression.
5. Conclusions
This investigation reveals that the coadministration of an NPY1R agonist with Ketamine significantly enhances antidepressant-like effects in rodent models, suggesting a novel approach for treating MDD. This synergistic effect not only induced antidepressant-like actions but also induced neurobiological changes within the ventral hippocampus. While Ketamine is recognized for its rapid action, our findings propose that introducing NPY1R agonism could provide new pathways to overcome the limitations of current treatments, such as delayed efficacy and incomplete response rates. Given the impact of standard antidepressants, further exploration into combining NPY1R agonists with these treatments presents a promising strategy, especially for addressing treatment-resistant depression. Future research should aim to translate these preclinical insights into clinical applications, exploring the full potential of NPY1R agonist combinations to enhance antidepressant efficacy and offer new hope to patients with MDD.
Declaration of interests
The authors have no relevant affiliations or financial involvement with any organization or entity with a financial interest in or financial conflict with the subject matter or materials discussed in the manuscript. This includes employment, consultancies, honoraria, stock ownership or options, expert testimony, grants or patents received or pending, or royalties.
Reviewer disclosures
Peer reviewers on this manuscript have no relevant financial or other relationships to disclose.
Author contributions
C Arrabal-Gómez contributed significantly to the experimental execution, conception and design of the study. He also played a vital role in the drafting of the manuscript and provided critical revisions related to important intellectual content.
P Serrano-Castro was heavily involved in the analysis and interpretation of data, ensuring the accuracy and integrity of the work. He also contributed to the manuscript’s critical revisions and approved the final version for publication.
JA Sánchez-Pérez focused on data collection phases of the study, contributing to both the drafting and critical revision processes of the manuscript. He approved the final manuscript version.
N Garcia-Casares participated in the study’s design, particularly in the selection of methodological approaches for data analysis. She was also involved in drafting the manuscript and revising it critically for important intellectual content.
K Fuxe and D Borroto-Escuela played a key role in the conception and design of the study, especially in integrating the neurobiological and pharmacological aspects. They contributed to drafting the manuscript and critically revising it for significant intellectual content.
M Narváez was instrumental in the overall conception, design, and coordination of the study. He led the drafting of the manuscript and its critical revision for important intellectual content. As the corresponding author, he also took responsibility for the final approval of the version to be published and agreed to be accountable for all aspects of the work. All authors have read and approved the final manuscript and agree to be accountable for all aspects of the work, ensuring that questions related to the accuracy or integrity of any part of the work are appropriately investigated and resolved.
Availability of data and materials
The data that support the findings of this study are openly available in the Institutional repository of the University of Malaga (RIUMA) and from the corresponding author upon reasonable request.
Ethics approval and consent to participate
All experimental protocols were approved by the Local Animal Ethics, Care, and Use Committee for the University of Málaga, Spain (CEUMA 45–2022-A), and conducted in accordance with the EU Directive 2010/63/EU and Spanish Directive (Real Decretory 53/2013).
Supplementary EOTT-revised.docx
Download MS Word (150.1 KB)Supplementary material
Supplemental data for this article can be accessed online at https://doi.org/10.1080/14728222.2024.2342524
Additional information
Funding
References
- Catala-Lopez F, Genova-Maleras R, Vieta E, et al. The increasing burden of mental and neurological disorders. Eur Neuropsychopharmacol. 2013 Nov;23(11):1337–1339.
- McEwen BS, Bowles NP, Gray JD, et al. Mechanisms of stress in the brain. Nat Neurosci. 2015 Oct;18(10):1353–1363.
- Schramm E, Klein DN, Elsaesser M, et al. Review of dysthymia and persistent depressive disorder: history, correlates, and clinical implications. Lancet Psychiatry. 2020 Sep;7(9):801–812.
- Kessler RC, Petukhova M, Sampson NA, et al. Twelve-month and lifetime prevalence and lifetime morbid risk of anxiety and mood disorders in the United States. Int J Methods Psychiatr Res. 2012 Sep;21(3):169–184.
- Karch DL, Logan J, McDaniel D, et al. Surveillance for violent deaths–national violent death reporting system, 16 states, 2009. MMWR Surveill Summ. 2012 Sep 14;61(6):1–43.
- Aleman A, Denys D. Mental health: a road map for suicide research and prevention. Nature. 2014 May 22;509(7501):421–423. doi: 10.1038/509421a
- World Health O. Front Matter. Depression and other common mental disorders. World Health Org. 2017. p. 2–2.
- Fitzmaurice C, Akinyemiju TF, Barber RM, et al. Global Burden of Disease Cancer C. Global, regional, and national cancer incidence, mortality, years of life lost, years lived with disability, and disability-adjusted life-years for 29 cancer groups, 1990 to 2016: a systematic analysis for the global burden of disease study. JAMA Oncol. 2018 Nov 1: 4(11):1553–1568.
- Jick H, Kaye JA, Jick SS. Antidepressants and the risk of suicidal behaviors. JAMA. 2004 Jul 21;292(3):338–343. doi: 10.1001/jama.292.3.338
- Morilak DA, Frazer A. Antidepressants and brain monoaminergic systems: a dimensional approach to understanding their behavioural effects in depression and anxiety disorders. Int J Neuropsychopharmacol. 2004 Jun;7(2):193–218. doi: 10.1017/S1461145704004080
- Kudlow PA, Cha DS, McIntyre RS. Predicting treatment response in major depressive disorder: the impact of early symptomatic improvement. Can J Psychiatry. 2012 Dec;57(12):782–788. doi: 10.1177/070674371205701211
- Trivedi MH, Rush AJ, Wisniewski SR, et al. Evaluation of outcomes with citalopram for depression using measurement-based care in STAR*D: implications for clinical practice. Am J Psychiatry. 2006 Jan;163(1):28–40.
- Fagiolini A, Kupfer DJ. Is treatment-resistant depression a unique subtype of depression? Biol Psychiatry. 2003 Apr 15;53(8):640–648.
- Berman RM, Cappiello A, Anand A, et al. Antidepressant effects of ketamine in depressed patients. Biol Psychiatry. 2000 Feb 15;47(4):351–354. doi: 10.1016/S0006-3223(99)00230-9
- Zarate CA Jr., Singh JB, Carlson PJ, et al. A randomized trial of an N-methyl-D-aspartate antagonist in treatment-resistant major depression. Arch Gen Psychiatry. 2006 Aug;63(8):856–864.
- Krystal JH, Sanacora G, Duman RS. Rapid-acting glutamatergic antidepressants: the path to ketamine and beyond. Biol Psychiatry. 2013 Jun 15;73(12):1133–1141. doi: 10.1016/j.biopsych.2013.03.026
- Liao Y, Tang YL, Hao W. Ketamine and international regulations. Am J Drug Alcohol Abuse. 2017 Sep;43(5):495–504. doi: 10.1080/00952990.2016.1278449
- Duman RS. Ketamine and rapid-acting antidepressants: a new era in the battle against depression and suicide. F1000Res. 2018;7:659. doi: 10.12688/f1000research.14344.1
- Targum SD, Daly E, Fedgchin M, et al. Comparability of blinded remote and site-based assessments of response to adjunctive esketamine or placebo nasal spray in patients with treatment resistant depression. J Psychiatr Res. 2019 Apr;111:68–73. doi: 10.1016/j.jpsychires.2019.01.017
- Zimmermann KS, Richardson R, Baker KD. Esketamine as a treatment for paediatric depression: questions of safety and efficacy. Lancet Psychiatry. 2020 Oct;7(10):827–829. doi: 10.1016/S2215-0366(19)30521-8
- Turner EH. Esketamine for treatment-resistant depression: seven concerns about efficacy and FDA approval. Lancet Psychiatry. 2019 Dec;6(12):977–979. doi: 10.1016/S2215-0366(19)30394-3
- Kaur U, Pathak BK, Singh A, et al. Esketamine: a glimmer of hope in treatment-resistant depression. Eur Arch Psychiatry Clin Neurosci. 2021 Apr;271(3):417–429.
- Zanos P, Moaddel R, Morris PJ, et al. NMDAR inhibition-independent antidepressant actions of ketamine metabolites. Nature. 2016 May 26;533(7604):481–486. doi: 10.1038/nature17998
- Fukumoto K, Toki H, Iijima M, et al. Antidepressant potential of (r)-ketamine in rodent models: comparison with (S)-Ketamine. J Pharmacol Exp Ther. 2017 Apr;361(1):9–16.
- Mahar I, Bambico FR, Mechawar N, et al. Stress, serotonin, and hippocampal neurogenesis in relation to depression and antidepressant effects. Neurosci Biobehav Rev. 2014 Jan;38:173–192. doi: 10.1016/j.neubiorev.2013.11.009
- Eliwa H, Belzung C, Surget A. Adult hippocampal neurogenesis: Is it the alpha and omega of antidepressant action? Biochem Pharmacol. 2017 Oct 1;141:86–99. doi: 10.1016/j.bcp.2017.08.005
- Haroon E, Miller AH, Sanacora G. Inflammation, glutamate, and glia: a trio of trouble in mood disorders. Neuropsychopharmacology. 2017 Jan;42(1):193–215. doi: 10.1038/npp.2016.199
- Thorsell A, Mathe AA. Neuropeptide Y in alcohol addiction and affective disorders. Front Endocrinol. 2017;8:178. doi: 10.3389/fendo.2017.00178
- Castren E, Monteggia LM. Brain-derived neurotrophic factor signaling in depression and antidepressant action. Biol Psychiatry. 2021 Jul 15;90(2):128–136. doi: 10.1016/j.biopsych.2021.05.008
- Pothula S, Kato T, Liu RJ, et al. Cell-type specific modulation of NMDA receptors triggers antidepressant actions. Mol Psychiatry. 2021 Sep;26(9):5097–5111.
- Gerhard DM, Pothula S, Liu RJ, et al. GABA interneurons are the cellular trigger for ketamine’s rapid antidepressant actions. J Clin Invest. 2020 Mar 2;130(3):1336–1349. doi: 10.1172/JCI130808
- Autry AE, Adachi M, Nosyreva E, et al. NMDA receptor blockade at rest triggers rapid behavioural antidepressant responses. Nature. 2011 Jun 15;475(7354):91–95. doi: 10.1038/nature10130
- Kavalali ET, Monteggia LM. Targeting homeostatic synaptic plasticity for treatment of mood disorders. Neuron. 2020 Jun 3;106(5):715–726. doi: 10.1016/j.neuron.2020.05.015
- Casarotto PC, Girych M, Fred SM, et al. Antidepressant drugs act by directly binding to TRKB neurotrophin receptors. Cell. 2021 Mar 4;184(5):1299–1313 e19. doi: 10.1016/j.cell.2021.01.034
- Kask A, Harro J, von Horsten S, et al. The neurocircuitry and receptor subtypes mediating anxiolytic-like effects of neuropeptide Y. Neurosci Biobehav Rev. 2002 May;26(3):259–283.
- Kormos V, Gaszner B. Role of neuropeptides in anxiety, stress, and depression: from animals to humans. Neuropeptides. 2013 Dec;47(6):401–419. doi: 10.1016/j.npep.2013.10.014
- Zaben MJ, Gray WP. Neuropeptides and hippocampal neurogenesis. Neuropeptides. 2013 Dec;47(6):431–438. doi: 10.1016/j.npep.2013.10.002
- Reichmann F, Holzer P. Neuropeptide Y: a stressful review. Neuropeptides. 2016 Feb;55:99–109. doi: 10.1016/j.npep.2015.09.008
- Jimenez-Vasquez PA, Diaz-Cabiale Z, Caberlotto L, et al. Electroconvulsive stimuli selectively affect behavior and neuropeptide Y (NPY) and NPY Y(1) receptor gene expressions in hippocampus and hypothalamus of flinders sensitive line rat model of depression. Eur Neuropsychopharmacol. 2007 Mar;17(4):298–308.
- Redrobe JP, Dumont Y, Fournier A, et al. The neuropeptide Y (NPY) Y1 receptor subtype mediates NPY-induced antidepressant-like activity in the mouse forced swimming test. Neuropsychopharmacology. 2002 May;26(5):615–624.
- Goyal SN, Upadhya MA, Kokare DM, et al. Neuropeptide Y modulates the antidepressant activity of imipramine in olfactory bulbectomized rats: involvement of NPY Y1 receptors. Brain Res. 2009 Apr 17;1266:45–53. doi: 10.1016/j.brainres.2009.02.033
- Sharma A, Ren X, Zhang H, et al. Effect of depression and suicidal behavior on neuropeptide Y (NPY) and its receptors in the adult human brain: a postmortem study. Prog Neuropsychopharmacol Biol Psychiatry. 2022 Jan 10;112:110428. doi: 10.1016/j.pnpbp.2021.110428
- Corvino V, Marchese E, Podda MV, et al. The neurogenic effects of exogenous neuropeptide Y: early molecular events and long-lasting effects in the hippocampus of trimethyltin-treated rats. PLOS ONE. 2014;9(2):e88294. doi: 10.1371/journal.pone.0088294
- Cohen H, Liu T, Kozlovsky N, et al. The neuropeptide Y (NPY)-ergic system is associated with behavioral resilience to stress exposure in an animal model of post-traumatic stress disorder. Neuropsychopharmacology. 2012 Jan;37(2):350–363.
- Cohen H, Vainer E, Zeev K, et al. Neuropeptide S in the basolateral amygdala mediates an adaptive behavioral stress response in a rat model of posttraumatic stress disorder by increasing the expression of BDNF and the neuropeptide YY1 receptor. Eur Neuropsychopharmacol. 2018 Jan;28(1):159–170.
- Porsolt RD, Le Pichon M, Jalfre M. Depression: a new animal model sensitive to antidepressant treatments. Nature. 1977 Apr 21;266(5604):730–732. doi: 10.1038/266730a0
- Planchez B, Surget A, Belzung C. Animal models of major depression: drawbacks and challenges. J Neural Transm (Vienna). 2019 Nov;126(11):1383–1408. doi: 10.1007/s00702-019-02084-y
- Yankelevitch-Yahav R, Franko M, Huly A, et al. The forced swim test as a model of depressive-like behavior. J Vis Exp. 2015 Mar 2;(97). doi: 10.3791/52587.
- Serova L, Mulhall H, Sabban E. NPY1 receptor agonist modulates development of depressive-like behavior and gene expression in hypothalamus in SPS rodent PTSD model. Front Neurosci. 2017;11:203. doi: 10.3389/fnins.2017.00203
- Borroto-Escuela DO, Fores R, Pita M, et al. Intranasal delivery of galanin 2 and neuropeptide Y1 agonists enhanced spatial memory performance and neuronal precursor cells proliferation in the dorsal hippocampus in rats. Front Pharmacol. 2022;13:820210. doi: 10.3389/fphar.2022.820210
- Ribeiro ACR, Zhu J, Kronfol MM, et al. Molecular mechanisms for the antidepressant-like effects of a low-dose ketamine treatment in a DFP-based rat model for gulf war illness. Neurotoxicology. 2020 Sep;80:52–59. doi: 10.1016/j.neuro.2020.06.011
- Koike H, Chaki S. Requirement of AMPA receptor stimulation for the sustained antidepressant activity of ketamine and LY341495 during the forced swim test in rats. Behav Brain Res. 2014 Sep 1;271:111–115. doi: 10.1016/j.bbr.2014.05.065
- Borroto-Escuela DO, Pita-Rodriguez M, Fores-Pons R, et al. Galanin and neuropeptide Y interactions elicit antidepressant activity linked to neuronal precursor cells of the dentate gyrus in the ventral hippocampus. J Cell Physiol. 2021 May;236(5):3565–3578.
- Narváez M, Crespo-Ramírez M, Fores-Pons R, et al. Study of GPCR homo- and heteroreceptor complexes in specific neuronal cell populations using the in situ proximity ligation assay. In: Lujan R Ciruela F, editors. Receptor and ion channel detection in the brain. New York (NY): Springer US; 2021. p. 117–134.
- Narvaez M, Andrade-Talavera Y, Valladolid-Acebes I, et al. Existence of FGFR1-5-HT1AR heteroreceptor complexes in hippocampal astrocytes. Putative link to 5-HT and FGF2 modulation of hippocampal gamma oscillations. Neuropharmacology. 2020 Jun 15;170:108070. doi: 10.1016/j.neuropharm.2020.108070
- Diaz-Sanchez E, Lopez-Salas A, Mirchandani-Duque M, et al. Decreased medial prefrontal cortex activity related to impaired novel object preference task performance following GALR2 and Y1R agonists intranasal infusion. Biomed Pharmacother. 2023 May;161:114433. doi: 10.1016/j.biopha.2023.114433
- Abrial E, Betourne A, Etievant A, et al. Protein kinase C inhibition rescues manic-like behaviors and hippocampal cell proliferation deficits in the sleep deprivation model of mania. Int J Neuropsychopharmacol. 2014 Oct 31;18(2). doi: 10.1093/ijnp/pyu031
- Pilar-Cuellar F, Vidal R, Pazos A. Subchronic treatment with fluoxetine and ketanserin increases hippocampal brain-derived neurotrophic factor, beta-catenin and antidepressant-like effects. Br J Pharmacol. 2012 Feb;165(4b):1046–1057. doi: 10.1111/j.1476-5381.2011.01516.x
- Paxinos G, Watson C. The rat brain in stereotaxic coordinates: hard cover edition. AE Amsterdam, The netherlands: Elsevier; 2006.
- Narvaez M, Borroto-Escuela DO, Santin L, et al. A novel integrative mechanism in anxiolytic behavior induced by galanin 2/Neuropeptide Y Y1 receptor interactions on medial paracapsular intercalated amygdala in rats. Front Cell Neurosci. 2018;12:119. doi: 10.3389/fncel.2018.00119
- Mirchandani-Duque M, Barbancho MA, Lopez-Salas A, et al. Galanin and neuropeptide Y interaction enhances proliferation of granule precursor cells and expression of neuroprotective factors in the rat hippocampus with consequent augmented spatial memory. Biomedicines. 2022 Jun 1;10(6):1297. doi: 10.3390/biomedicines10061297
- Narvaez M, Millon C, Borroto-Escuela D, et al. Galanin receptor 2-neuropeptide Y Y1 receptor interactions in the amygdala lead to increased anxiolytic actions. Brain Struct Funct. 2015 Jul;220(4):2289–2301.
- Narvaez M, Borroto-Escuela DO, Millon C, et al. Galanin receptor 2-neuropeptide Y Y1 receptor interactions in the dentate gyrus are related with antidepressant-like effects. Brain Struct Funct. 2016 Nov;221(8):4129–4139.
- Cohen H, Zohar J, Kaplan Z, et al. Adjunctive treatment with brexpiprazole and escitalopram reduces behavioral stress responses and increase hypothalamic NPY immunoreactivity in a rat model of PTSD-like symptoms. Eur Neuropsychopharmacol. 2018 Jan;28(1):63–74.
- Li N, Lee B, Liu RJ, et al. mTOR-dependent synapse formation underlies the rapid antidepressant effects of NMDA antagonists. Science. 2010 Aug 20;329(5994):959–964. doi: 10.1126/science.1190287
- Polis AJ, Fitzgerald PJ, Hale PJ, et al. Rodent ketamine depression-related research: finding patterns in a literature of variability. Behav Brain Res. 2019 Dec 30;376:112153. doi: 10.1016/j.bbr.2019.112153
- Detke MJ, Rickels M, Lucki I. Active behaviors in the rat forced swimming test differentially produced by serotonergic and noradrenergic antidepressants. Psychopharmacol (Berl). 1995 Sep;121(1):66–72. doi: 10.1007/BF02245592
- Rafalo-Ulinska A, Branski P, Palucha-Poniewiera A. Combined administration of (R)-ketamine and the mGlu2/3 receptor antagonist LY341495 induces rapid and sustained effects in the CUMS model of depression via a TrkB/BDNF-dependent mechanism. Pharmaceuticals (Basel). 2022 Jan 21;15(2):125. doi: 10.3390/ph15020125
- Liu RJ, Fuchikami M, Dwyer JM, et al. GSK-3 inhibition potentiates the synaptogenic and antidepressant-like effects of subthreshold doses of ketamine. Neuropsychopharmacology. 2013 Oct;38(11):2268–2277.
- Fuxe K, Dahlstrom AB, Jonsson G, et al. The discovery of central monoamine neurons gave volume transmission to the wired brain. Prog Neurobiol. 2010 Feb 9;90(2):82–100. doi: 10.1016/j.pneurobio.2009.10.012
- Borroto-Escuela DO, Agnati LF, Bechter K, et al. The role of transmitter diffusion and flow versus extracellular vesicles in volume transmission in the brain neural–glial networks. Philos Trans R Soc Lond B Biol Sci. 2015 Jul 5;370(1672):20140183. doi: 10.1098/rstb.2014.0183
- Jin Z, Han Y, Zhang D, et al. Application of intranasal administration in the delivery of antidepressant active ingredients. Pharmaceutics. 2022 Sep 28;14(10):2070. doi: 10.3390/pharmaceutics14102070
- Alvarez-Contino JE, Diaz-Sanchez E, Mirchandani-Duque M, et al. GALR2 and Y1R agonists intranasal infusion enhanced adult ventral hippocampal neurogenesis and antidepressant-like effects involving BDNF actions. J Cell Physiol. 2023 Jan 4;238(2):459–474. doi: 10.1002/jcp.30944
- Sun HL, Zhou ZQ, Zhang GF, et al. Role of hippocampal p11 in the sustained antidepressant effect of ketamine in the chronic unpredictable mild stress model. Transl Psychiatry. 2016 Feb 23;6(2):e741. doi: 10.1038/tp.2016.21
- Sabban EL, Serova LI. Potential of intranasal neuropeptide Y (NPY) and/or melanocortin 4 receptor (MC4R) antagonists for preventing or treating PTSD. Mil Med. 2018 Mar 1;183(suppl_1):408–412. doi: 10.1093/milmed/usx228
- Deyama S, Duman RS. Neurotrophic mechanisms underlying the rapid and sustained antidepressant actions of ketamine. Pharmacol Biochem Behav. 2020 Jan;188:172837. doi: 10.1016/j.pbb.2019.172837
- Perera TD, Coplan JD, Lisanby SH, et al. Antidepressant-induced neurogenesis in the hippocampus of adult nonhuman primates. J Neurosci. 2007 May 2;27(18):4894–4901. doi: 10.1523/JNEUROSCI.0237-07.2007
- Yamada J, Jinno S. Potential link between antidepressant-like effects of ketamine and promotion of adult neurogenesis in the ventral hippocampus of mice. Neuropharmacology. 2019 Nov 1;158:107710. doi: 10.1016/j.neuropharm.2019.107710
- Choi M, Lee SH, Chang HL, et al. Hippocampal VEGF is necessary for antidepressant-like behaviors but not sufficient for antidepressant-like effects of ketamine in rats. Biochim Biophys Acta. 2016 Jul;1862(7):1247–1254.
- Michaelsson H, Andersson M, Svensson J, et al. The novel antidepressant ketamine enhances dentate gyrus proliferation with no effects on synaptic plasticity or hippocampal function in depressive-like rats. Acta Physiol (Oxf). 2019 Apr;225(4):e13211.
- Duman RS, Monteggia LM. A neurotrophic model for stress-related mood disorders. Biol Psychiatry. 2006 Jun 15;59(12):1116–1127. doi: 10.1016/j.biopsych.2006.02.013
- Castren E, Kojima M. Brain-derived neurotrophic factor in mood disorders and antidepressant treatments. Neurobiol Dis. 2017 Jan;97(Pt B):119–126. doi: 10.1016/j.nbd.2016.07.010
- Hing B, Sathyaputri L, Potash JB. A comprehensive review of genetic and epigenetic mechanisms that regulate BDNF expression and function with relevance to major depressive disorder. Am J Med Genet B Neuropsychiatr Genet. 2018 Mar;177(2):143–167. doi: 10.1002/ajmg.b.32616
- Dwivedi Y. Involvement of brain-derived neurotrophic factor in late-life depression. Am J Geriatr Psychiatry. 2013 May;21(5):433–449. doi: 10.1016/j.jagp.2012.10.026
- Ray MT, Shannon Weickert C, Webster MJ. Decreased BDNF and TrkB mRNA expression in multiple cortical areas of patients with schizophrenia and mood disorders. Transl Psychiatry. 2014 May 6;4(5):e389. doi: 10.1038/tp.2014.26
- Youssef MM, Underwood MD, Huang YY, et al. Association of BDNF Val66Met polymorphism and brain BDNF levels with major depression and suicide. Int J Neuropsychopharmacol. 2018 Jun 1;21(6):528–538. doi: 10.1093/ijnp/pyy008
- Pandey GN, Ren X, Rizavi HS, et al. Brain-derived neurotrophic factor and tyrosine kinase B receptor signalling in post-mortem brain of teenage suicide victims. Int J Neuropsychopharmacol. 2008 Dec;11(8):1047–1061.
- Chen B, Dowlatshahi D, MacQueen GM, et al. Increased hippocampal BDNF immunoreactivity in subjects treated with antidepressant medication. Biol Psychiatry. 2001 Aug 15;50(4):260–265. doi: 10.1016/S0006-3223(01)01083-6
- Lucas G, Hendolin P, Harkany T, et al. Neurotrophin-4 mediated TrkB activation reinforces morphine-induced analgesia. Nat Neurosci. 2003 Mar;6(3):221–222.
- Monteggia LM, Barrot M, Powell CM, et al. Essential role of brain-derived neurotrophic factor in adult hippocampal function. Proc Natl Acad Sci U S A. 2004 Jul 20;101(29):10827–10832. doi: 10.1073/pnas.0402141101
- Adachi M, Barrot M, Autry AE, et al. Selective loss of brain-derived neurotrophic factor in the dentate gyrus attenuates antidepressant efficacy. Biol Psychiatry. 2008 Apr 1;63(7):642–649. doi: 10.1016/j.biopsych.2007.09.019
- Li Y, Luikart BW, Birnbaum S, et al. TrkB regulates hippocampal neurogenesis and governs sensitivity to antidepressive treatment. Neuron. 2008 Aug 14;59(3):399–412. doi: 10.1016/j.neuron.2008.06.023
- Heilig M, Soderpalm B, Engel JA, et al. Centrally administered neuropeptide Y (NPY) produces anxiolytic-like effects in animal anxiety models. Psychopharmacol (Berl). 1989;98(4):524–529. doi: 10.1007/BF00441953
- Redrobe JP, Dumont Y, Fournier A, et al. Role of serotonin (5-HT) in the antidepressant-like properties of neuropeptide Y (NPY) in the mouse forced swim test. Peptides. 2005 Aug;26(8):1394–1400.