ABSTRACT
Introduction
Rapid and accurate diagnostic approaches are essential for impeding the spread of infectious diseases. This review aims to summarize current progress of clustered regularly interspaced short palindromic repeats (CRISPR)‐associated (Cas) systems in the applications for diagnostics of infectious diseases including the ongoing COVID-19 epidemic.
Areas covered
In this review, we discuss class 2 CRISPR-Cas biosensing systems-based diagnostics in various emerging and reemerging infectious diseases, CRISPR-Cas systems have created a new era for early diagnostics of infectious diseases, especially with the discovery of the collateral cleavage activity of Cas12 and Cas13. We mainly focus on different CRISPR-Cas effectors for the detection of pathogenic microorganisms as well as provide a detailed explanation of the pros and cons of CRISPR-Cas biosensing systems. In addition, we also introduce future research perspectives.
Expert commentary
However, further improvement of newly discovered systems and engineering existing ones should be developed to increase the specificity, sensitivity or stability of the diagnostic tools. It may be a long journey to finish the clinical transition from research use. CRISPR-Cas approaches will emerge as more promising and robust tools for infectious disease diagnosis in the future.
1. Introduction
Infectious diseases are the most dangerous threats to human beings, accounting for 15% of total mortality worldwide [Citation1] and bringing an immense burden of economic loss and public distress [Citation2]. Infectious diseases are often caused by different pathogenic microorganisms, including bacteria, viruses, fungi and parasites [Citation3]. In the twenty-first century, some new infectious diseases emerged, such asswine flu, bird flu, Ebola virus, Zika viruses, MERS, and SARS, as well as the ongoing SARS-CoV-2 epidemic [Citation4–6]. Moreover, a few old diseases have been resurgent, including human papillomavirus (HPV), human immunodeficiency virus (HIV), Mycobacterium tuberculosis (TB) bacteria, dengue and malarial parasites [Citation7–9]. Unlike other diseases, infectious diseases can be widely spread among populations in a relatively short time; thus, it is crucial to rapidly ascertain the aetiologic pathogens and achieve early diagnosis of infected individuals.
‘Without diagnostics, medicine is blind.’ Recently, a variety of assays have been developed, such as pathogen culture, protein-based assays, and nucleic acid-based detection [Citation10]. Among these assays, pathogen culture is time consuming, while protein-based assays, especially antigen detection, require the preparation of antibodies. The fast, ultrasensitive, specific and reliable detection of pathogen nucleic acid sequences has become the most powerful tool for infectious disease diagnosis [Citation11]. This conventional technique is to isolate nucleic acids from infected biological samples and then detect nucleic acid signatures by polymerase chain reaction (PCR), QPCR, isothermal amplification or next-generation sequencing [Citation12–14]. Although these nucleic acid-based assays have been the most common and important approaches for disease diagnosis, PCR/QPCR and next-generation sequencing require sophisticated and expensive equipment and high-level personnel expertise. Low sensitivity and specificity limit the application of isothermal amplification.
The World Health Organization (WHO) has released the ‘ASSURED’ criteria for ideal diagnostic assays: affordable, sensitive, specific, user-friendly, rapid and robust, equipment-free and deliverable to end users [Citation15]. The WHO also emphasized that it is urgent to develop better diagnostic techniques for prioritizing infectious diseases. Recently, a novel nucleic acid-based assay, the clustered regularly interspaced short palindromic repeats (CRISPR)-associated (Cas) nuclease system, has emerged to promisingly affect the disease diagnostic field [Citation16].
The CRISPR locus was first discovered in Escherichia coli in 1987 [Citation17], and CRISPR-associated proteins were identified in 2002 [Citation18]. Subsequently, the function of the CRISPR-Cas system has been deeply explored, and the system evolved to implement a role in bacterial adaptive immunity [Citation19–21]. The application of genome editing and genetic engineering rapidly promoted the development of the CRISPR-Cas system, which revolutionized both research and clinical achievement [Citation22–24]. This technique has been exploited by numerous laboratories to edit the genomes and RNA of different species, including Caenorhabditis elegans [Citation25], filamentous fungi [Citation26], bacteria [Citation27,Citation28], rice [Citation29,Citation30], zebrafish [Citation31,Citation32], mice [Citation33–35] and humans [Citation36–38]. In addition, the CRISPR-Cas system has been harnessed for nucleic acid detection. The CRISPR/Cas9-based assay was first performed to detect Zika virus in 2016 [Citation39] and methicillin-resistant Staphylococcus aureus in 2017 [Citation40]. Nuclease-deactivated Cas9 (dCas9) loses the DNA cleavage activity of Cas9 nuclease and retains the binding ability to target DNA [Citation41], which facilitates tracking of specific nucleic acid loci and manipulation for nucleic acid detection by a dCas9/sgRNA-signal readout domain fusion [Citation42–44]. Later, the discovery of Cas12 [Citation45] and Cas13 [Citation46] further revolutionized the field of nucleic acid detection because these novel Cas proteins not only have the ability to recognize and target DNA/RNA cleavage but also have collateral cleavage activity on nontarget single-stranded DNA (ssDNA) [Citation47] or single-stranded RNA (ssRNA) [Citation48]. They have been widely applied in infectious and noninfectious disease diagnosis [Citation49–52]. Another protein, Cas14, seems to recognize and cleave target ssDNA without a rigid PAM sequence and has ‘collateral’ cleavage activity for ssDNA [Citation53]. CRISPR/Cas-based nucleic acid detection has engendered much excitement, and it is promising to develop low-cost, specific and sensitive diagnostics for infectious diseases. Unsurprisingly, discovery of the CRISPR-Cas system was awarded the Nobel Prize this year [Citation54].
In this review, we cover the applications of the CRISPR-Cas system for infectious disease diagnostics, particularly in COVID-19, mainly focusing on different CRISPR-Cas effectors for the detection of pathogenic microorganisms. We will also provide a detailed explanation of the pros and cons of CRISPR-Cas biosensing systems and introduce future research perspectives.
2. CRISPR-Cas biology
Three decades ago, CRISPR-Cas systems were identified in bacterial genomes, which were recently confirmed to be a type of adaptive immune system that fends off invasive genetic elements, such as viruses, bacteriophages and plasmids [Citation55]. This adaptive immune system is found in approximately half of bacteria and almost all archaea [Citation56]. Snippets of foreign DNA (35 to 45 nucleotides) are captured and inserted into the genomic CRISPR locus as a viral memory storehouse, which is a collection of unique interspaced 20–58 bp DNA segments separated by a 20–40 bp repeated sequence. The clusters of Сas genes encoding enzymes with nucleolytic activity are located downstream of the CRISPR repeat-spacer arrays. Several Cas proteins have been found to have other enzymatic activities, such as polymerase and helicase activities, which can be harnessed for nucleic acid manipulation. The precursor CRISPR RNA (crRNA) is transcribed from the CRISPR array and then trimmed into mature crRNAs by Cas proteins and other proteins. Mature crRNAs interact with one or more Cas nucleases to form a RNP complex that specifically destroys and degrades foreign DNA molecules by base‐pairing crRNAs with target nucleic acids. Finally, CRISPR-Cas systems protect against foreign invaders [Citation57–59]. Thus, the function of CRISPR/Cas-mediated adaptive immunity can be summarized into three processes: adaptation, crRNA maturation and interference [Citation60].
3. CRISPR-Cas classification
Classification of CRISPR-Cas systems is mainly based on evolutionary relationships. Some criteria have been considered for classification schemes, such as phylogenetic algorithms and clustering, architectural organization of Cas proteins, and phylogeny of Cas1 [Citation61,Citation62]. According to the published classification, CRISPR-Cas systems are partitioned into two major classes that include six types and forty-eight subtypes [Citation63]. Class 1 CRISPR-Cas systems can be subdivided into 3 types: types I, III and IV. They utilize interference machinery comprised of complexes of multiple Cas effectors and crRNA to cleave the target nucleic acid sequence. It has been reported that approximately 90% of CRISPR-Cas systems belong to class 1 systems, which are frequently discovered in fungi, with a small percentage identified in bacteria [Citation64]. Class 2 CRISPR-Cas systems also consist of 3 types: types II, V and VI. They are characterized by a single multi-function Cas effector together with crRNA for interference [Citation46]. Class 2 systems are commonly found in bacteria. Due to their simplicity and high efficiency, class 2 CRISPR-Cas systems have been widely utilized in genome editing and nucleic acid detection assays for human pathogens, especially CRISPR-Cas9, -Cas12a and -Cas13a.
3.1. Class 1 CRISPR-Cas system classification
Three types of class 1 CRISPR-Cas systems are type I, III and IV systems with different characteristic nucleases, including the enzymes Cas3, Cas10 and Cas8-like (csf1), respectively [Citation65–67]. Type I systems are composed of Cas protein complexes and a crRNA molecule. The protospacer adjacent motif (PAM) is recognized by the Cascade complex, Cas6 or Cas5 mediates crRNA unwinding and binding with target DNA, the Cas7 backbone stabilizes the R-loop, then Cas3 cleaves the target DNA [Citation68]. Type III systems comprise crRNA, Csm complexes (III-A systems), and Cmr complexes (III-B systems). Cas10 is the signature protein of type III systems, and Cas10 polymerase activity can generate cyclic oligo-(A) nucleotides (cOA), which bind with Csm6 and activate the RNase activity of Csm6, so they often cleave target RNA rather than DNA [Citation69,Citation70]. CRISPR-Cas type IV systems are found in plasmids, and the interference mechanisms are largely unknown [Citation71].
3.2. Class 2 CRISPR-Cas system classification
Class 2 CRISPR-Cas systems have type II, V and VI systems with distinct characteristic nucleases, including the enzymes Cas9, Cas12/Cas14 and Cas13, respectively. Compared to the multiple Cas effectors of class 1 systems, class 2 systems require only a single multidomain protein to complex with crRNA for nucleic acid surveillance and cleavage.
Type II systems are the most well-known CRISPR-Cas systems, with four subtypes, which are characterized by the Cas9 protein [Citation72]. A dual RNA, tracrRNA and crRNA are essential for recruiting and guiding the Cas9 protein to cleave the desired DNA sequence. tracrRNA is complemented with the repeat regions of the pre-crRNA, while crRNA binds to complementary sequences of the target DNA. The crRNA-tracrRNA complex mediates Cas9 recognition of PAM sequences (5ʹ-NGG-3ʹ) on target DNA, and then the HNH and RuvC-like nuclease domains of Cas9 cleave double-stranded DNA and generate blunt ends [Citation46]. Recently, Jinek et al. reconstructed a single-guide RNA (sgRNA) to replace the crRNA-tracrRNA dual RNA [Citation73], which simplifies type II systems and promotes the application of CRISPR-Cas genome editing.
Type V systems are the largest group among all CRISPR-Cas systems, with 17 subtypes [Citation63]. In these systems, the Cas12 protein is one of the signature proteins, and the RuvC-like nuclease domain of Cas12 cuts the target dsDNA and leaves sticky ends [Citation45]. Type V systems consist only of a crRNA and the Cas protein, and the crRNA-Cas12 complex recognizes PAM sequences (5ʹ-(T)TTN-3ʹ) on target DNA [Citation74]. In another new subtype of type V systems, the Cas14 protein has been discovered and has only 400–700 aa. Cas14 can destroy ssDNA in the absence of T-rich PAM sequences [Citation53]. Collateral activity of both the Cas12 and Cas14 proteins has been found: once bound to target DNA, these proteins cut any adjacent non-target DNA.
Type VI systems are the most recently discovered systems, containing five derivative subtypes. Similar to type V, type VI does not require tracrRNA, and Cas13 is the characteristic protein that possesses two HEPEN RNase domains [Citation75]. crRNA-Cas13 recognizes protospacer flanking site (PFS) sequences and destroys the target ssRNA with a blunt-ended break; additionally, adjacent non-target RNA can be cleaved in a nonspecific manner [Citation76–78].
4. Infectious disease diagnostics via CRISPR-Cas systems
A number of CRISPR-based technologies used to detect nucleic acids of aetiologic pathogens have been reported. Early studies mainly focused on Cas9 or nuclease-dead Cas9 (dCas9) effectors of type II systems. When the collateral activity of type V (Cas12 and Cas14 effector) and VI (Cas13 effector) systems was exploited, CRISPR-Cas system-based diagnostics were rapidly developed.
4.1. Specific cleavage- or DNA binding-based diagnostics
4.1.1. CRISPR-Cas9 system-based diagnostics
CRISPR-Cas9 systems have been the most useful tools for genome editing and epigenetic control. Recently, some groups also reported that it is feasible to perform molecular diagnostics for infectious disease by using CRISPR-Cas9 systems.
Pardee et al. developed nucleic acid sequence-based amplification (NASBA)-CRISPR to discriminate American and African strains of Zika virus as well as detect Zika virus from plasma [Citation39]. The sensitivity of this technology can reach 1–3 fM. The principle of this approach is associated with toehold switch sensors and the specific cleavage ability of Cas9 (). Toehold switch sensors are synthetic riboregulators that control LacZ translation by binding RNA. In the absence of binding RNA, the hairpin structure of riboregulators impedes LacZ translation through sequestering the start codon and the ribosome-binding site. While the trigger RNA is complemented to the riboregulator, the start codon and the ribosome-binding site are exposed, and the translation of LacZ is initiated. Finally, LacZ catalyses the conversion of a yellow substrate to a purple product. Double-stranded DNA is generated by the NASBA reaction. When CRISPR-Cas9 cleaves dsDNA, the RNA product transcribed from cleaved dsDNA cannot activate the toehold switch sensor due to the lack of a sensor trigger sequence. In their research, they amplified Zika virus RNA using isothermal amplification, targeting the binding RNA of riboregulators.
Figure 1. Schematic depiction of NASBACC detection. RNA extracted from viruses is amplified into dsDNA by NASBA reaction. When CRISPR-Cas9 cleaves the dsDNA, RNA product transcribed from the cleaved dsDNA can not activate the toehold switch sensor due to the lack of sensor trigger sequence. Otherwise, the toehold switch sensor is activated to initiate LacZ expression, which induces the color change of substrates
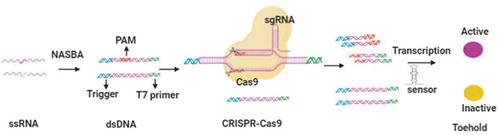
The CRISPR-typing PCR (ctPCR) technique was constructed by Wang and colleagues to detect two high-risk HPVs (HPV16 and 18) [Citation79]. First, universal primers are used to amplify target DNA (PCR1). Second, the Cas9 protein cleaves PCR1 products, while a tailing and T adaptor are ligated to the cleavage products. Third, the cleavage products are amplified with a pair of general-specific primers (PCR2), and PCR2 products are identified by gel readout or fluorescence ().
Figure 2. Schematic illustration of ctPCR detection. The DNA samples are amplified by PCR, and two sgRNAs are designed to bind with the different target DNA sequence. After the cleavage of Cas9-sgRNA, A tailing and T adaptor are ligated to cleavage products, then gel readout or fluorescence are detected by using PCR or qPCR
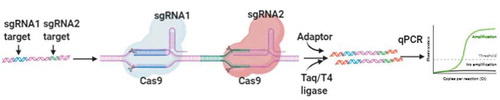
Huang et al. paired CRISPR-Cas9 with isothermal exponential amplification reaction (EXPAR) to establish the Cas-EXPAR approach [Citation80]. PAM-mers are designed to be complemented with the PAM sequence of single-stranded DNA or RNA, and CRISPR-Cas9 cuts single-stranded DNA molecules into short fragments (X-fragments) with the help of the PAM-mer. Then, the X-fragments hybridize with an EXPAR template that contains two complementary flanking regions of the X-fragment and a central NEase recognition site. X-fragments are cleaved by NEase and released from the EXPAR template by Vent (exo-) DNA polymerase. Moreover, X-fragments are cyclically amplified, and the products can be measured by QPCR. The technique has been used to identify DNA methylation and Listeria monocytogenes RNA with attomolar (aM) sensitivity.
Strand displacement amplification is an efficient isothermal DNA amplification tool that is significant for on-site diagnostics. Zhou and colleagues developed a CRISPR–Cas9-triggered nicking endonuclease (S. pyogenes Cas9H840A nickase)-mediated strand displacement amplification (CRISDA) strategy to detect nucleic acids [Citation81]. This nickase cuts only a single DNA strand, and two sgRNAs are designed to be complementary with each border of the dsDNA. Subsequently, the SDA reaction is initiated. Both biotin-labeled PNA and Cy5-labeled PNA probes are added to target the SDA amplicon. Finally, magnetic beads are used to capture the SDA amplicon, and the fluorescence intensity of Cy5 is measured. It has been shown that the sensitivity of CRISDA is up to the sub-attomolar level.
Finding low abundance sequences by hybridization (FLASH) was introduced by Quan and colleagues in 2019 [Citation82]. This technique has been harnessed for the detection of drug resistance genes in pneumonia-causing gram-positive bacteria and in the malaria parasite Plasmodium falciparum. The genomic DNA or cDNA of pathogens is treated with phosphatase and cut with a set of sgRNA-Cas9 complexes. These digested products are ligated to universal sequencing adapters. After amplification, the products are subjected to Illumina sequencing.
Recently, another group developed the CRISPR/Cas9-mediated lateral flow nucleic acid assay (CASLFA) based on CRISPR-Cas9 systems and a lateral flow assay [Citation83]. In this study, Listeria monocytogenes and hundreds of copies of African swine fever virus (ASFV) were identified with high specificity and accuracy in less than 1 h.
4.1.2. CRISPR-dCas9 system-based diagnostics
Nuclease-deficient Cas9 (dCas9) is engineered from Cas9, which possesses DNA-binding ability without cleavage activity. CRISPR-dCas9 has been utilized for transcription regulation, gene locus imaging and disease diagnostics.
Zhang et al. fused two halves of split luciferase with dCas9, and a pair of sgRNAs were designed to target DNA molecules [Citation84]. When these two parts of luciferase are adjacent to each other, the activity of split luciferase will be recovered. By using this approach, they established the identification of Mycobacterium tuberculosis DNA with high sensitivity and specificity. Similarly, the combination of CRISPR-dCas9 and split horseradish peroxidase successfully identified microRNAs [Citation85].
CRISPR-mediated DNA-FISH was created to detect 10 cfu/ml methicillin-resistant Staphylococcus aureus (MRSA) within 0.5 h by Kyeonghye Guk et al [Citation40]. CRISPR-Chip is a label-free nucleic acid-testing approach that is based on CRISPR-dCas9 and a graphene-based field-effect transistor (gFET). The dCas9/sgRNA complex (dRNP) is immobilized on the graphene surface, and the fixed dRNP recognizes its target DNA to regulate the electrical signal output in 15 min, with a sensitivity of 1.7 fM [Citation86].
4.2. Collateral cleavage-based diagnostics
4.2.1. CRISPR-Cas12 and -Cas14 system-based diagnostics
Cas12a (also named Cpf1), a class V Cas protein, was first discovered in 2015 [Citation45]. Cas12a can complex with crRNA to cut target DNA; on the other hand, it has collateral nonspecific ssDNA cleavage activity. Therefore, CRISPR-Cas12 systems are suitable for the application of genome manipulation and nucleic acid detection. According to the characteristics of Cas12a, the DNA endonuclease targeted CRISPR trans reporter (DETECTR) approach was created by using LbCas12a [Citation47]. Viral nucleic acids are extracted, and amplification is conducted with isothermal preamplification (PRA) or RT-PRA. Cas12a proteins targeting crRNA and single-stranded FQ-DNA reporters (a fluorophore and a quencher are linked to different sides of ssDNA) are mixed with DNA samples. The binding of the crRNA and target DNA induces Cas12a proteins to cut the target and nontarget DNA. Consequently, a strong fluorescent signal can be generated (). In this research, they utilized this strategy to detect two high-risk types of HPVs (HPV16 and 18). crRNAs specifically bound to the hypervariable loop V region of the L1 gene locus of HPV16 and 18. The subtypes of HPVs were effectively discriminated in cell culture and clinical samples. Other Cas12 proteins derived from different organisms have been used to develop the DETECTR approach for disease diagnostics, including FnCas12a, AsCas12a and AaCas12b.
Figure 3. Schematic illustration of nucleic acid detection based on Cas12 effectors. dsDNA molecules are amplified from RNA or DNA by using RT-RAP/RAP, RT-PCR/PCR or RT-LAMP/LAMP. The Cas12 effectors bind to target dsDNA and non-target ssDNA, then the ssDNA reporter can be destroyed and the fluorescence is generated. (A) DETECTR system. (B) HOLMES system. (C) HOLMESv2 system
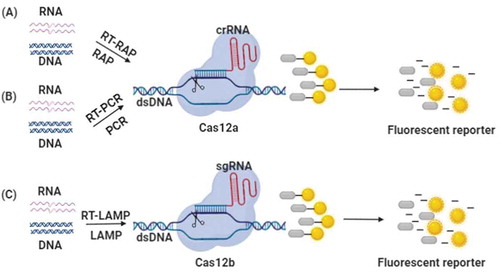
Li et al. built another time-effective and cost-effective nucleic acid detection technique based on CRISPR-Cas12a, named HOLMES (one-HOur Low-cost Multipurpose highly Efficient System), which was used to identify DNA viruses (e.g. pseudorabies virus (PRV)) and RNA viruses (e.g. Japanese encephalitis virus (JEV)). Together with PCR amplification, this technique can detect nucleic acids with a sensitivity of 1–10 aM [Citation49](). Later, this group further improved HOLMES to HOLMESv2, which is based on Cas12b rather than Cas12a. Compared to HOLMES, HOLMESv2 combines loop-mediated isothermal amplification (LAMP) amplification with CRISPR-Cas12b and integrates two separate steps of amplification and detection into one system(). HOLMESv2 can be used to discriminate SNPs, detect RNA and quantitate DNA methylation [Citation87]. Another Cas12b-mediated detection (CDetection) was developed to detect HPV16 DNA in human plasma with a sensitivity of 1 aM [Citation88].
Dai and colleagues created a CRISPR-Cas12a-based electrochemical biosensor (E-CRISPR) that relies on electrochemical signals rather than fluorescence readouts [Citation89]. A nonspecific ssDNA reporter is tagged with methylene blue and immobilized on a gold electrode. In the presence of the target DNA, CRISPR-Cas12a destroys the ssDNA reporter and results in the separation of the methylene blue tag as well as a lower electrochemical signal(). E-CRISPR has been applied for viral detection (e.g. HPV-16 and PB-19), with picomolar sensitivity.
Figure 4. Schematic illustration of E-CRISPR method. Nonspecific ssDNA reporter is tagged with methylene blue and immobilized on the gold electrode. In the presence of the target DNA, CRISPR-Cas12a destroy ssDNA reporter and result in the separation of methylene blue tag as well as the lower electrochemical signal
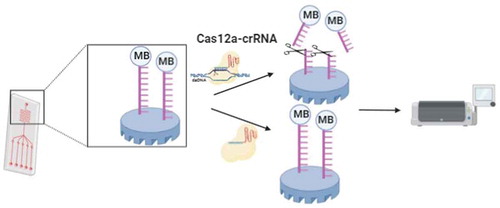
Cas14 proteins, novel type V proteins, have been found recently. They are smaller than other Cas proteins. Similar to Cas12, Cas14 contains a RuvC domain that cleaves target ssDNA in cis without a PAM and cuts nontarget ssDNA in trans. Although Cas14a-DETECTR has been used to detect SNPs and plant ssDNA viruses, no pathogen diagnosis has been reported [Citation90].
4.2.2. CRISPR-Cas13 system-based diagnostics
Cas13a (also named C2C2), a class VI Cas protein, was first discovered in 2015. The Cas13a-crRNA complex recognizes and cuts target RNA; on the other hand, it has collateral nonspecific ssRNA cleavage activity. Specific high-sensitivity enzymatic reporter unlocking (SHERLOCK) is dependent on the collateral activity of Leptotrichia shahii Cas13a (LshCas13a) [Citation48]. DNA amplification is performed by RPA or RT-RPA, and the products are then transcribed into RNA in vitro. Cas13a proteins targeting crRNA and single-stranded FQ-RNA reporters are mixed with amplified RNA. The binding of crRNA and target DNA activates Cas13a proteins to degrade the target and nontarget ssRNA. Consequently, the fluorescence intensity can be measured. SHERLOCK has been explored to detect Zika and Dengue viruses with attomolar sensitivity (eg. Zika, about 1.25 copies/μl; Dengue, 2 copies/μl). It is also easy to distinguish different pathogenic viruses and bacteria and human DNA genotypes and identify mutations in tumor DNA. This system has some drawbacks, such as the lack of quantitation, only one target detection in a single reaction and the need for fluorescence readout equipment.
Later, SHERLOCK was further developed to an advanced version with a multiplexed platform, which is termed SHERLOCKv2 [Citation91]. This method relies on the CRISPR-Cas12 and CRISPR-Cas13 systems, which contain four types of Cas proteins: AsCas12a, LwaCas13a, PsmCas13b and CcaCas13b. AsCas12a exhibits collateral nontarget ssDNA activity. LwaCas13a, CcaCas13b and PsmCas13b could destroy the three dinucleotide ssRNA reporters with AU, UC and GA, respectively. Then, these four reporters for Cas proteins are labeled with different fluorophores, and the targets are detected in the FAM, Cy5, HEX and TEX channels. SHERLOCKv2 qualitatively evaluates drug resistance genes and ZIKV and DENV RNA samples at 2 ~ 10 copies/μl concentrations. Additionally, the detection sensitivity is increased more than threefold by harnessing Csm6, an auxiliary CRISPR-associated enzyme that boosts the collateral activity of PsmCas13b and LwaCas13a. The SHERLOCKv2 system has been explored for visual colorimetric lateral-flow readouts based on FAM-biotin reporters. In this system, streptavidin binds to biotin, while gold nanoparticle-labeled anti-FAM antibodies bind to the FAM fluorophore of the reporter. Once the FAM-biotin reporters are degraded, anti-FAM antibodies will flow to the test line captured by secondary antibody in the lateral flow strips, and a visual colorimetric signal is generated. By using this SHERLOCKv2 lateral flow system, Zika and Dengue ssRNA viruses have been identified in patient bodily fluid samples.
To avoid complicated nucleic acid extraction, Myhrvold et al. combined SHERLOCK with heating unextracted diagnostic samples to obliterate nucleases (HUDSON) to detect nucleic acids directly from patient liquid biopsy samples in more than 2 h [Citation92]. This on-site strategy is very suitable for remote areas without laboratory diagnostic expertise and expensive equipment. In this system, heat and chemical reduction are used to lyse viral particles and inactivate ribonucleases from bodily fluids. After treatment, the urine or saliva is mixed with RPA reaction components. Subsequently, the procedure is finished with amplification and detection (). HUDSON-SHERLOCK can sensitively detect ZIKV nucleic acids with a visual readout. The authors also confirmed that the detection limit of this approach for viral particles was 90 aM in serum or whole blood, 20 aM in urine and 0.9 aM in saliva.
4.3. COVID-19 detection by CRISPR-Cas systems
Coronaviruses are the major aetiologic pathogens of livestock, pets and humans, causing respiratory and neurological diseases [Citation93]. Coronaviruses include less virulent species (HKU1, NL63, 229E and OC43) and more virulent species (MERS-CoV, SARS-CoV and SARS-CoV-2) [Citation94]. Currently, the ongoing outbreak of coronavirus disease 2019 (COVID-19) seriously threatens humanity [Citation95]. COVID-19 is caused by SARS-CoV-2, whose origin may be bats [Citation96]. To date, there are approximately 57 million SARS-CoV-2 infection cases and more than 1.3 million deaths worldwide. To prevent the rapid spread of the virus, early diagnostics are vital for reducing the risk of further transmission. The current diagnostic approaches rely on nucleic acid detection of COVID-19, such as PCR/QPCR and next-generation sequencing [Citation97,Citation98]. However, these techniques have some drawbacks, including low efficiency, false negatives, expensive instruments and high-level personnel expertise. It is difficult to catch up with the rapid rise in infectious patients, so faster, better and simpler diagnostic approaches are needed relatively urgently.
Feng Zhang et al. published a protocol to detect COVID-19 within 1 h by using CRISPR-based SHERLOCK [Citation99]. According to the protocol, RNA was isolated from patient samples, and an RPA mixture was added to amplify nucleic acids. Next, the viral RNA sequence was detected by CRISPR-Cas13, and visual readout can be achieved in a lateral flow dipstick. Ali and colleagues introduced an all-in-one dual CRISPR-Cas12a (termed ‘AIOD-CRISPR’) method for discrimination of coronavirus SARS-CoV-2 and HIV from human plasma samples with high sensitivity, as low as 1.2 copies DNA targets and 4.6 copies RNA targets. The fluorescent readout can be visualized under blue light and UV light [Citation100]. Other groups also applied Cas12-based DETECTR and Cas13a-based SHERLOCK for the detection of SARS-CoV-2 [Citation101–104]. Undoubtedly, CRISPR-Cas systems are promising tools for COVID-19 diagnostics.
5. Conclusion
Mature pathogen detection approaches include culture of pathogenic microorganisms, antigen detection, PCR amplification-based detection, next-generation sequencing and new CRISPR-based detection. All these methods have distinct characteristics, and the features are listed in . In this section, we will discuss the pros and cons of CRISPR-based diagnostic technologies. As I mentioned above, compared with conventional pathogen detection approaches, the advantages of CRISPR-Cas systems are obvious: high sensitivity, rapid turnover, high specificity, on-site operation, low cost, early diagnosis, and no requirement for expensive equipment or qualified personnel with high-level personnel expertise. In addition, the diversity of Cas proteins and the easy change in target-specific SgRNA expand the applicability of CRISPR-based detection.
Table 1. Comparison of CRISPR approach with other pathogen detection approaches
Nevertheless, some major drawbacks should be considered. The sequences recognized by CRISPR-Cas effector proteins are limited, which is a general problem in most CRISPR-Cas systems. A specific PAM sequence is essential for Cas9/dCas9 and Cas12a/b to bind with target dsDNA, while a PFS is required for the Cas13 effector. The ‘off‐target’ effect is another disadvantage of CRISPR-Cas systems, particularly Cas9, which leads to false negative or positive results, but online software can reduce the probability of off‐target effects. Cas proteins have the characteristic of nucleotide mismatch tolerance, and it causes nonspecific binding of templates by Cas proteins, which reduces the signal and the reliability of the test. On the other hand, mismatch tolerance may increase robustness to identify mutation-prone pathogens. Cas protein detection alone has lower sensitivity, and the combination of target nucleic acid amplification and CRISPR-Cas systems solves this issue. Similar to PCR-based assays, CRISPR-Cas systems rely on known DNA sequences, so they are less robust for unknown outbreaks and limit their application. Meanwhile, due to PCR-based assays are easier to set up and are familiar to us, major efforts should be done by companies and researchers who want to invest in this field to promote CRISPR-Cas systems.
6. Expert Opinion
Since CRISPR-Cas systems were discovered a decade ago, different Cas proteins and sg/crRNAs have been engineered to expand the application of CRISPR-Cas systems. As I mentioned, many challenges and limitations of CRISPR-Cas systems should be solved in the future, such as the specific PAM sequence or SPF, the ‘off‐target’ effect of CRISPR-Cas systems, and mismatch tolerance. Therefore, further improvement of newly discovered systems and engineering existing ones will undoubtedly increase the specificity, sensitivity or stability of the diagnostic tools. Moreover, Cas protein detection alone has lower sensitivity, it is difficult to confirm the pathogens by using CRISPR-Cas system only. Together with other technologies, they can be widely applied to identify various emerging and reemerging infectious diseases, new synthetic elements or engineering materials may also enhance the signal readout of diagnostic techniques together with CRISPR-Cas systems, which is expected to skip the step of nucleic acid amplification and further simplify the operation. Viral genomes are prone to mutate in response to various selection pressures, such as tissue and host tropism, host immune responses, and drug treatment. As CRISPR-Cas systems are sensitive to mutations, this implies accurate and balanced selection of the target sequence between highly conserved sequences, which, if spread out among the whole viral family, can be little informative among the viral type, or type-specific sequences that entail specific and narrow applications of the technology. If viruses mutate, a diagnostic method based on a single viral target is unwise. For SARS-CoV-2 variants, some molecular diagnostic tests that miss one viral target made this concept clear. To minimize false-negative results, therefore, it is strongly advisable to develop CRISPR-Cas systems detecting at least two targets in the viral genome. Multiplexing is another challenge of CRISPR-Cas-based detection. When pathogens are unknown in patient samples, several pathogens need to be detected in a single assay simultaneously. Although SHERLOCKv2 has provided the detection of 4 targets, this is quite limited, and more targets should be included in the clinic in the next step. CRISPR-Cas assays have superiority in cost and speed, for instance, the cost of SHERLOCK is less than 1 USD per test, and SHERLOCKv2 takes no more than 20 min. Through great efforts toward optimization, the cost and speed will be improved in the future. In addition to nucleic acid identification, CRISPR-Cas12a has been explored to detect other small molecules, including p-hydroxybenzoic acid and uric acid [Citation105]. It is possible that more modified CRISPR technologies will be created to test other molecules. Point-of-care testing is another goal that CRISPR-Cas-based diagnosis pursues, and the systems can be developed as portable microfluidic-based POC devices. Although CRISPR-Cas systems have been confirmed as encouraging strategies for molecule detection and disease diagnostics in research area, and the systems have been developed as novel and powerful approaches to detect the ongoing COVID-19 epidemic due to the advantages of the speed, the cost and the easy-use by some research groups, their accuracy and sensitivity should be carefully validated in real-world situations. Clinical samples are more complicated and lower quality, with multiple inhibiting factors and distinct interfering compounds, and real life is different from the controlled laboratory environment, false negative or false positive results must be paid more attention, other mature approaches will be performed to confirm the accuracy of results. It is uncertain whether CRISPR-Cas systems have the same outstanding performance as they display in laboratory settings. Therefore, it may be a long journey to finish the clinical transition from research use. In addition, the storage and transportation of CRISPR-based test kits at room temperature should also be given additional attention. Finally, automation and artificial intelligence can greatly strengthen the application of CRISPR-Cas systems in disease diagnostics. We do believe CRISPR-Cas approaches will emerge as more promising and robust tools for infectious disease diagnosis in the future.
Article highlights
CRISPR-Cas systems have been confirmed as encouraging strategies for molecule detection and infectious disease diagnostics.
The collateral activity of type V (Cas12 and Cas14 effector) and VI (Cas13 effector) systems was exploited and rapidly developed for infectious disease diagnostics.
Some groups also applied Cas12-based DETECTR and Cas13a-based SHERLOCK for the detection of SARS-CoV-2. It is significant to prevent the spread of COVID-19 epidemic.
Reviewer disclosures
Peer reviewers on this manuscript have no relevant financial or other relationships to disclose.
Declaration of interest
The authors have no relevant affiliations or financial involvement with any organization or entity with a financial interest in or financial conflict with the subject matter or materials discussed in the manuscript. This includes employment, consultancies, honoraria, stock ownership or options, expert testimony, grants or patents received or pending, or royalties.
Additional information
Funding
References
- WHO. Global health estimates 2016: disease burden by cause, age, sex, by country and by religion, 2000–2016. Geneva: World Health Organization; 2018.
- Chen H, Liu K, Li Z, et al. Point of care testing for infectious diseases. Clin Chim Acta. 2019 Jun;493:138–147.
- Hwang H, Hwang B-Y, Bueno J. Biomarkers in infectious diseases. Dis Markers. 2018;2018:1–2.
- Nii-Trebi NI. Emerging and neglected infectious diseases: insights, advances, and challenges. Biomed Res Int. 2017;2017:5245021.
- Weber DJ, Rutala WA, Fischer WA, et al. Emerging infectious diseases: focus on infection control issues for novel coronaviruses (severe acute respiratory syndrome-CoV and middle east respiratory syndrome-CoV), hemorrhagic fever viruses (Lassa and Ebola), and highly pathogenic avian influenza viruses, A(H5N1) and A(H7N9). Am J Infect Control. 2016 May 2;44(5 Suppl):e91–e100.
- Morens DM, Fauci AS. Emerging pandemic diseases: how we got to COVID-19. Cell. 2020 Sep 3;182(5):1077–1092.
- Banuls AL, Sanou A, Van Anh NT, et al. Mycobacterium tuberculosis: ecology and evolution of a human bacterium. J Med Microbiol. 2015 Nov;64(11):1261–1269.
- Brejt JA, Golightly LM. Severe malaria: update on pathophysiology and treatment. Curr Opin Infect Dis. 2019 Oct;32(5):413–418.
- Muller DA, Depelsenaire AC, Young PR. Clinical and laboratory diagnosis of dengue virus infection. J Infect Dis. 2017 Mar 1;215(suppl_2):S89–S95.
- Bhattacharyya RP, Thakku SG, Hung DT. Harnessing CRISPR effectors for infectious disease diagnostics. ACS Infect Dis. 2018 Sep 14;4(9):1278–1282.
- Caliendo AM, Gilbert DN, Ginocchio CC, et al. Better tests, better care: improved diagnostics for infectious diseases. Clin Infect Dis. 2013 Dec;57(Suppl 3):S139–70.
- Yang S, Rothman RE. PCR-based diagnostics for infectious diseases: uses, limitations, and future applications in acute-care settings. Lancet Infect Dis. 2004;4(6):337–348.
- Obande GA, Banga Singh KK. Current and future perspectives on isothermal nucleic acid amplification technologies for diagnosing infections. Infect Drug Resist. 2020;13:455–483.
- Matthijs G, Souche E, Alders M, et al. Guidelines for diagnostic next-generation sequencing. Eur J Hum Genet. 2016 Jan;24(1):2–5.
- Kosack CS, Page AL, Klatser PR. A guide to aid the selection of diagnostic tests. Bull World Health Organ. 2017 Sep 1;95(9):639–645.
- Pickar-Oliver A, Gersbach CA. The next generation of CRISPR-Cas technologies and applications. Nat Rev Mol Cell Biol. 2019 Aug;20(8):490–507.
- Ishino Y, Shinagawa H, Makino K, et al. Nucleotide sequence of the iap gene, responsible for alkaline phosphatase isozyme conversion in Escherichia coli, and identification of the gene product. J Bacteriol. 1987 Dec;169(12):5429–5433.
- Jansen R, Embden JD, Gaastra W, et al. Identification of genes that are associated with DNA repeats in prokaryotes. Mol Microbiol. 2002 Mar;43(6):1565–1575.
- Barrangou R. CRISPR-Cas systems and RNA-guided interference. Wiley Interdiscip Rev RNA. 2013 May-Jun;4(3):267–278.
- Horvath P, Barrangou R. CRISPR/Cas, the immune system of bacteria and archaea. Science. 2010 Jan 8;327(5962):167–170.
- Brouns SJ, Jore MM, Lundgren M, et al. Small CRISPR RNAs guide antiviral defense in prokaryotes. Science. 2008 Aug 15;321(5891):960–964.
- Jinek M, East A, Cheng A, et al. RNA-programmed genome editing in human cells. Elife. 2013 Jan;29(2):e00471.
- Cong L, Ran FA, Cox D, et al. Multiplex genome engineering using CRISPR/Cas systems. Science. 2013 Feb 15;339(6121):819–823.
- Gupta D, Bhattacharjee O, Mandal D, et al. CRISPR-Cas9 system: a new-fangled dawn in gene editing. Life Sci. 2019 Sep 1;232:116636.
- Kim HM, Colaiacovo MP. CRISPR-Cas9-guided genome engineering in caenorhabditis elegans. Curr Protoc Mol Biol. 2019 Dec;129(1):e106.
- Song R, Zhai Q, Sun L, et al. CRISPR/Cas9 genome editing technology in filamentous fungi: progress and perspective. Appl Microbiol Biotechnol. 2019 Sep;103(17):6919–6932.
- Jiang W, Bikard D, Cox D, et al. RNA-guided editing of bacterial genomes using CRISPR-Cas systems. Nat Biotechnol. 2013 Mar;31(3):233–239.
- Javed MR, Sadaf M, Ahmed T, et al. CRISPR-Cas system: history and prospects as a genome editing tool in microorganisms. Curr Microbiol. 2018 Dec;75(12):1675–1683.
- Oliva R, Ji C, Atienza-Grande G, et al. Broad-spectrum resistance to bacterial blight in rice using genome editing. Nat Biotechnol. 2019 Nov;37(11):1344–1350.
- Wang M, Mao Y, Lu Y, et al. Multiplex gene editing in rice using the CRISPR-Cpf1 system. Mol Plant. 2017 Jul 5;10(7):1011–1013.
- Zhang Y, Qin W, Lu X, et al. Programmable base editing of zebrafish genome using a modified CRISPR-Cas9 system. Nat Commun. 2017 Jul 25;8(1):118.
- Fernandez JP, Vejnar CE, Giraldez AJ, et al. Optimized CRISPR-Cpf1 system for genome editing in zebrafish. Methods. 2018 Nov 1;150:11–18.
- Hall B, Cho A, Limaye A, et al. Genome editing in mice using CRISPR/Cas9 technology. Curr Protoc Cell Biol. 2018 Dec;81(1):e57.
- Roper J, Tammela T, Akkad A, et al. Colonoscopy-based colorectal cancer modeling in mice with CRISPR-Cas9 genome editing and organoid transplantation. Nat Protoc. 2018 Feb;13(2):217–234.
- Chen S, Lee B, Lee AY, et al. Highly efficient mouse genome editing by CRISPR ribonucleoprotein electroporation of zygotes. J Biol Chem. 2016 Jul 8;291(28):14457–14467.
- Bak RO, Dever DP, Porteus MH. CRISPR/Cas9 genome editing in human hematopoietic stem cells. Nat Protoc. 2018 Feb;13(2):358–376.
- Richardson CD, Kazane KR, Feng SJ, et al. CRISPR-Cas9 genome editing in human cells occurs via the Fanconi anemia pathway. Nat Genet. 2018 Aug;50(8):1132–1139.
- Salsman J, Dellaire G. Precision genome editing in the CRISPR era. Biochem Cell Biol. 2017 Apr;95(2):187–201.
- Pardee K, Green AA, Takahashi MK, et al. Rapid, Low-cost detection of zika virus using programmable biomolecular components. Cell. 2016 May 19;165(5):1255–1266.
- Guk K, Keem JO, Hwang SG, et al. A facile, rapid and sensitive detection of MRSA using a CRISPR-mediated DNA FISH method, antibody-like dCas9/sgRNA complex. Biosens Bioelectron. 2017 Sep 15;95:67–71.
- Gilbert LA, Larson MH, Morsut L, et al. CRISPR-mediated modular RNA-guided regulation of transcription in eukaryotes. Cell. 2013 Jul 18;154(2):442–451.
- Chen B, Gilbert LA, Cimini BA, et al. Dynamic imaging of genomic loci in living human cells by an optimized CRISPR/Cas system. Cell. 2013 Dec 19;155(7):1479–1491.
- Ma H, Naseri A, Reyes-Gutierrez P, et al. Multicolor CRISPR labeling of chromosomal loci in human cells. Proc Natl Acad Sci U S A. 2015 Mar 10;112(10):3002–3007.
- Qin P, Parlak M, Kuscu C, et al. Live cell imaging of low- and non-repetitive chromosome loci using CRISPR-Cas9. Nat Commun. 2017 Mar 14;8:14725.
- Zetsche B, Gootenberg JS, Abudayyeh OO, et al. Cpf1 is a single RNA-guided endonuclease of a class 2 CRISPR-Cas system. Cell. 2015 Oct 22;163(3):759–771.
- Shmakov S, Abudayyeh OO, Makarova KS, et al. Discovery and functional characterization of diverse class 2 CRISPR-Cas systems. Mol Cell. 2015 Nov 5;60(3):385–397.
- Chen JS, Ma E, Harrington LB, et al. CRISPR-Cas12a target binding unleashes indiscriminate single-stranded DNase activity. Science. 2018 Apr 27;360(6387):436–439.
- Gootenberg JS, Abudayyeh OO, Lee JW, et al. Nucleic acid detection with CRISPR-Cas13a/C2c2. Science. 2017 Apr 28;356(6336):438–442.
- Li SY, Cheng QX, Wang JM, et al. CRISPR-Cas12a-assisted nucleic acid detection. Cell Discov. 2018;4:20.
- Zhang YM, Zhang Y, Xie K. Evaluation of CRISPR/Cas12a-based DNA detection for fast pathogen diagnosis and GMO test in rice. Mol Breed. 2020;40(1). DOI:10.1007/s11032-019-1092-2
- Qin P, Park M, Alfson KJ, et al. Rapid and fully microfluidic ebola virus detection with CRISPR-Cas13a. ACS Sens. 2019 Apr 26;4(4):1048–1054.
- Ackerman CM, Myhrvold C, Thakku SG, et al. Massively multiplexed nucleic acid detection with Cas13. Nature. 2020 Jun;582(7811):277–282.
- Harrington LB, Burstein D, Chen JS, et al. Programmed DNA destruction by miniature CRISPR-Cas14 enzymes. Science. 2018 Nov 16;362(6416):839–842.
- Cohen J. A cut above: pair that developed CRISPR earns historic award. Science. 2020 Oct 16;370(6514):271–272.
- Garneau JE, Dupuis ME, Villion M, et al. The CRISPR/Cas bacterial immune system cleaves bacteriophage and plasmid DNA. Nature. 2010 Nov 4;468(7320):67–71.
- Bhaya D, Davison M, Barrangou R. CRISPR-Cas systems in bacteria and archaea: versatile small RNAs for adaptive defense and regulation. Annu Rev Genet. 2011;45:273–297.
- Hochstrasser ML, Doudna JA. Cutting it close: CRISPR-associated endoribonuclease structure and function. Trends Biochem Sci. 2015 Jan;40(1):58–66.
- Charpentier E, Richter H, Van Der Oost J, et al. Biogenesis pathways of RNA guides in archaeal and bacterial CRISPR-Cas adaptive immunity. FEMS Microbiol Rev. 2015 May;39(3):428–441.
- Sternberg SH, Redding S, Jinek M, et al. DNA interrogation by the CRISPR RNA-guided endonuclease Cas9. Nature. 2014 Mar 6;507(7490):62–67.
- Sternberg SH, Richter H, Charpentier E, et al. Adaptation in CRISPR-Cas Systems. Mol Cell. 2016 Mar 17;61(6):797–808.
- Koonin EV, Makarova KS, Zhang F. Diversity, classification and evolution of CRISPR-Cas systems. Curr Opin Microbiol. 2017 Jun;37:67–78.
- Koonin EV, Makarova KS. Origins and evolution of CRISPR-Cas systems. Philos Trans R Soc Lond B Biol Sci. 2019 May 13;374(1772):20180087.
- Makarova KS, Wolf YI, Iranzo J, et al. Evolutionary classification of CRISPR-Cas systems: a burst of class 2 and derived variants. Nat Rev Microbiol. 2020 Feb;18(2):67–83.
- Makarova KS, Zhang F, Koonin EV. SnapShot: class 1 CRISPR-Cas Systems. Cell. 2017 Feb 23;168(5):946–946 e1.
- Liu TY, Doudna JA. Chemistry of Class 1 CRISPR-Cas effectors: binding, editing, and regulation. J Biol Chem. 2020 Oct 16;295(42):14473–14487.
- Gong B, Shin M, Sun J, et al. Molecular insights into DNA interference by CRISPR-associated nuclease-helicase Cas3. Proc Natl Acad Sci U S A. 2014 Nov 18;111(46):16359–16364.
- Van Der Oost J, Westra ER, Jackson RN, et al. Unravelling the structural and mechanistic basis of CRISPR-Cas systems. Nat Rev Microbiol. 2014 Jul;12(7):479–492.
- Xiao Y, Luo M, Hayes RP, et al. Structure basis for directional R-loop formation and substrate handover mechanisms in type I CRISPR-Cas system. Cell. 2017 Jun 29;170(1):48–60 e11.
- Samai P, Pyenson N, Jiang W, et al. Co-transcriptional DNA and RNA cleavage during type III CRISPR-Cas immunity. Cell. 2015 May 21;161(5):1164–1174.
- Liu T, Pan S, Li Y, et al. Type III CRISPR-Cas system: introduction and its application for genetic manipulations. Curr Issues Mol Biol. 2018;26:1–14.
- Ozcan A, Pausch P, Linden A, et al. Type IV CRISPR RNA processing and effector complex formation in Aromatoleum aromaticum. Nat Microbiol. 2019 Jan;4(1):89–96.
- Chylinski K, Makarova KS, Charpentier E, et al. Classification and evolution of type II CRISPR-Cas systems. Nucleic Acids Res. 2014 Jun;42(10):6091–6105.
- Jinek M, Chylinski K, Fonfara I, et al. A programmable dual-RNA-guided DNA endonuclease in adaptive bacterial immunity. Science. 2012 Aug 17;337(6096):816–821.
- Yan WX, Hunnewell P, Alfonse LE, et al. Functionally diverse type V CRISPR-Cas systems. Science. 2019 Jan 4;363(6422):88–91.
- Anantharaman V, Makarova KS, Burroughs AM, et al. Comprehensive analysis of the HEPN superfamily: identification of novel roles in intra-genomic conflicts, defense, pathogenesis and RNA processing. Biol Direct. 2013 Jun;15(8):15.
- Abudayyeh OO, Gootenberg JS, Essletzbichler P, et al. RNA targeting with CRISPR-Cas13. Nature. 2017 Oct 12;550(7675):280–284.
- Cox DBT, Gootenberg JS, Abudayyeh OO, et al. RNA editing with CRISPR-Cas13. Science. 2017 Nov 24;358(6366):1019–1027.
- O’Connell MR. Molecular mechanisms of RNA targeting by Cas13-containing type VI CRISPR-Cas systems. J Mol Biol. 2019 Jan 4;431(1):66–87.
- Wang Q, Zhang B, Xu X, et al. CRISPR-typing PCR (ctPCR), a new Cas9-based DNA detection method. Sci Rep. 2018 Sep 20;8(1):14126.
- Huang M, Zhou X, Wang H, et al. Clustered regularly interspaced short palindromic repeats/Cas9 triggered isothermal amplification for site-specific nucleic acid detection. Anal Chem. 2018 Feb 6;90(3):2193–2200.
- Zhou W, Hu L, Ying L, et al. A CRISPR-Cas9-triggered strand displacement amplification method for ultrasensitive DNA detection. Nat Commun. 2018 Nov 27;9(1):5012.
- Quan J, Langelier C, Kuchta A, et al. FLASH: a next-generation CRISPR diagnostic for multiplexed detection of antimicrobial resistance sequences. Nucleic Acids Res. 2019 Aug 22;47(14):e83.
- Wang X, Xiong E, Tian T, et al. Clustered regularly interspaced short palindromic repeats/Cas9-mediated lateral flow nucleic acid assay. ACS Nano. 2020 Feb 25;14(2):2497–2508.
- Zhang Y, Qian L, Wei W, et al. Paired design of dCas9 as a systematic platform for the detection of featured nucleic acid sequences in pathogenic strains. ACS Synth Biol. 2017 Feb 17;6(2):211–216.
- Qiu XY, Zhu LY, Zhu CS, et al. Highly effective and low-cost microRNA detection with CRISPR-Cas9. ACS Synth Biol. 2018 Mar 16;7(3):807–813.
- Hajian R, Balderston S, Tran T, et al. Detection of unamplified target genes via CRISPR-Cas9 immobilized on a graphene field-effect transistor. Nat Biomed Eng. 2019 Jun;3(6):427–437.
- Li L, Li S, Wu N, et al. HOLMESv2: a CRISPR-Cas12b-assisted platform for nucleic acid detection and DNA methylation quantitation. ACS Synth Biol. 2019 Oct 18;8(10):2228–2237.
- Teng F, Guo L, Cui T, et al. CDetection: CRISPR-Cas12b-based DNA detection with sub-attomolar sensitivity and single-base specificity. Genome Biol. 2019 Jul 1;20(1):132.
- Dai Y, Somoza RA, Wang L, et al. Exploring the trans-cleavage activity of CRISPR-Cas12a (cpf1) for the development of a universal electrochemical biosensor. Angew Chem Int Ed Engl. 2019 Nov 25;58(48):17399–17405.
- Khan MZ, Haider S, Mansoor S, et al. Targeting plant ssDNA viruses with engineered miniature CRISPR-Cas14a. Trends Biotechnol. 2019 Aug;37(8):800–804.
- Gootenberg JS, Abudayyeh OO, Kellner MJ, et al. Multiplexed and portable nucleic acid detection platform with Cas13, Cas12a, and Csm6. Science. 2018 Apr 27;360(6387):439–444.
- Myhrvold C, Freije CA, Gootenberg JS, et al. Field-deployable viral diagnostics using CRISPR-Cas13. Science. 2018 Apr 27;360(6387):444–448.
- Fehr AR, Perlman S. Coronaviruses: an overview of their replication and pathogenesis. Methods Mol Biol. 2015;1282:1–23.
- Su S, Wong G, Shi W, et al. Epidemiology, genetic recombination, and pathogenesis of coronaviruses. Trends Microbiol. 2016 Jun;24(6):490–502
- Malik YS, Kumar N, Sircar S, et al. Coronavirus disease pandemic (COVID-19): challenges and a global perspective. Pathogens. 2020 Jun 28;9:7.
- Latinne A, Hu B, Olival KJ, et al. Origin and cross-species transmission of bat coronaviruses in China. Nat Commun. 2020 Aug 25;11(1):4235.
- Arumugam A, Faron ML, Yu P, et al. A rapid SARS-CoV-2 RT-PCR assay for low resource settings. Diagnostics (Basel). 2020;10(10). doi:10.3390/diagnostics10100739.
- Pillay S, Giandhari J, Tegally H, et al. Whole genome sequencing of SARS-CoV-2: adapting illumina protocols for quick and accurate outbreak investigation during a pandemic. Genes (Basel). 2020 17;11(8):949.
- Zhang F, Abudayyeh OO, Gootenberg JS. A protocol for detection of COVID-19 using CRISPR diagnostics. 2020.
- Ding X, Yin K, Li Z, et al. All-in-one dual CRISPR-Cas12a (AIOD-CRISPR) assay: a case for rapid, ultrasensitive and visual detection of novel coronavirus SARS-CoV-2 and HIV virus. bioRxiv. 2020.
- Broughton JP, Deng X, Yu G, et al. CRISPR–Cas12-based detection of SARS-CoV-2. Nat Biotechnol. 2020;38(7):870–874.
- Krammer F, Hou T, Zeng W, et al. Development and evaluation of a rapid CRISPR-based diagnostic for COVID-19. PLoS Pathog. 2020;16(8).
- Ali Z, Aman R, Mahas A, et al. iSCAN: an RT-LAMP-coupled CRISPR-Cas12 module for rapid, sensitive detection of SARS-CoV-2. Virus Res. 2020 Oct 15;288:198129.
- Lotfi M, Rezaei N. CRISPR/Cas13: a potential therapeutic option of COVID-19. Biomed Pharmacother. 2020 Nov;131:110738.
- Liang M, Li Z, Wang W, et al. A CRISPR-Cas12a-derived biosensing platform for the highly sensitive detection of diverse small molecules. Nat Commun. 2019 Aug 14;10(1):3672.