Abstract
Three new n-alkyl dithiocarbamate compounds, as sodium salts, C4H9NHCS2Na (I), C6H13NHCS2Na (II) and C8H17NHCS2Na (III), were synthesized and examined for inhibition of both cresolase and catecholase activities of mushroom tyrosinase (MT) from a commercial source of Agaricus bisporus in 10 mM phosphate buffer pH 6.8, at 293K using UV spectrophotometry. Caffeic acid and p-coumaric acid were used as natural substrates for the enzyme for the catecholase and cresolase reactions, respectively. Lineweaver–Burk plots showed different patterns of mixed and competitive inhibition for catecholase and cresolase reactions, respectively. These new synthetic compounds can be classified as potent inhibitors of MT due to Ki values of 0.8, 1.0 and 1.8 μM for cresolase inhibitory activity, and also 9.4, 14.5 and 28.1 μM for catecholase inhibitory activity for I, II and III, respectively. They showed a greater potency in the inhibitory effect towards the cresolase activity of MT. Both substrate and inhibitor can be bound to the enzyme with negative cooperativity between the binding sites (α>1) and this negative cooperativity increases with increasing length of the aliphatic tail in these compounds. The inhibition mechanism is presumably related to the chelating of the binuclear coppers at the active site and the different Ki values may be related to different interaction of the aliphatic chains of I, II and III with the hydrophobic pocket in the active site of the enzyme.
Introduction
Tyrosinase (EC 1.14.18.1) as a bifunctional enzyme catalyzes the o-hydroxylation of monophenols to the corresponding catechols (cresolase activity), and the oxidation of catechols to the corresponding o-quinones (catecholase activity) [Citation1]. Quinones are highly reactive compounds, which can polymerize spontaneously (non-enzymatically) to form melanin as the most important natural biopolymer responsible of pigmentation and the color and patterns of mammalian skin [Citation2,Citation3]. In some vegetables and fruits, tyrosinase is responsible for browning and is considered to be deleterious to the color quality of plant-derived foods and beverages [Citation4]. This enzyme is widely distributed through the phylogenetic scale from lower to higher life forms.
Tyrosinase has three forms: met, deoxy, and oxy [Citation5]. Structural models for the active site of these three enzyme forms have been proposed [Citation6]. Basically, the enzyme tyrosinase has three domains, of which the central domain contains two Cu binding sites, called CuA and CuB [Citation7]. Common mushroom tyrosinase (MT) from the species Agaricus bisporus is a copper containing enzyme with a molecular mass of 120 kD and is composed of two H subunits (43 kD) and two L subunits (13 kD) and contains two active site [Citation8,Citation9]. The active site consists of a pair of copper ions, which are each bound by three conserved histidine residues [Citation10].
Tyrosinase inhibitors have attracted interest recently due to undesired browning in vegetables and fruits in post-harvest handling [Citation11]. Besides, tyrosinase inhibitors may be clinically used for treatment of some skin disorders associated with melanin hyper-pigmentation and are also important in cosmetics for skin whitening effects Citation12-16.
There is a vast variety of natural and synthetic inhibitors known against catecholase, cresolase or both reactions of tyrosinase. Polyphenols, aldehydes and their derivatives are the most important inhibitors from plant natural sources Citation17-21. Besides higher plants, some compounds from fungal sources have also been identified, e.g. metallothionein from Aspergillus niger has strong avidity to chelate copper at the active site of MT, thereby acting as a strong inhibitor [Citation22]. Kojic acid, an antibiotic, produced by species of Aspergillus and Penicillum in the aerobic process acts as a potent, “slow-binding”, competitive inhibitor of tyrosinase Citation23-27, and is widely used as a cosmetic whitening agent Citation27-30. Synthetic tyrosinase inhibitors may be used as drugs and chemicals. In the case of clinical drugs, captopril, an antihypertensive drug, and methimazole act as tyrosinase inhibitors [Citation31,Citation32]. Simple chemical species capable of binding to copper, such as cyanide, azide, and halide ions, as expected behave as purely competitive inhibitors towards dioxygen binding, even if sharp differences have been seen among polyphenoloxidases from different sources [Citation33]. Other chemicals such as hydrogen peroxide, hydroxylamine, aromatic carboxylic acids, tiron, thiols, and aromatic carboxylic acids have a widespread application as tyrosinase inhibitors [Citation34] and, here, sulfur-containing compounds such as tiron, thiol and sulfites are among the most important tyrosinase inhibitors. The most commonly applied inhibitor of the discoloration process currently is sulfite [Citation11]. Thiol compounds such as cysteine, glutathione, methimazole, diethyldithiocarbamate, 2-mercaptobenzothiazole have been used as inhibitors of tyrosinase from different sources Citation35-39.
After a number of reports on the stability, modification and additional information about the structure and function of MT Citation40-44, two new bi-pyridine synthetic compounds were recently introduced as potent uncompetitive inhibitors for the enzyme [Citation45]. In the present investigation, inhibitory effects of three synthetic n-alkyl dithiocarbamates, as sodium salts, with different aliphatic tails (C4, C6 and C8) are described and the kinetics of their inhibition have been elucidated in both cresolase and catecholase activities.
Materials and methods
Materials
Mushroom tyrosinase (MT; EC1.14.18.1), specific activity 3400 units/mg, was purchased from Sigma. Caffeic acid and p-coumaric acid were available as from authentic samples. n-Butyl dithiocarbamate (I), n-hexyl dithiocarbamate (II) and n-octyl dithiocarbamate (III), sodium salts (), were synthesized (their synthesis and characterization is being published elsewhere). The buffer used in the assay was 10 mM phosphate buffer, pH = 6.8, and its salts were obtained from Merck. All experiments were carried at 293K.
Methods
The kinetic assays of catecholase and cresolase activities for the inhibition experiments were carried out using a Cary spectrophotometer, 100 Bio model, with jacketed cell holders. Freshly prepared enzyme, substrate and inhibitor were used in this work. All the enzymatic reactions were run in phosphate buffer (10 mM) at pH = 6.8 in a conventional quartz cell thermostated to maintain the temperature at 293 ± 0.1K. The selected conditions of solvent, buffer, pH, temperature, and enzyme concentration applied for assaying the oxidase activity of MT followed the method introduced by El-Bayuomi and Frieden [Citation46]. Inhibition of MT by I, II and III compounds was studied using caffeic acid (catecholase activity) and p-coumaric acid (cresolase activity) as substrates. Catecholase activity was followed by depletion of caffeic acid for 2 min at its λmax = 311 nm and enzyme concentration of 11.8 μM, 40 unit/ml. The reactions were carried out using seven different fixed concentrations of substrate (40–160 μM) in different concentrations of the inhibitors (0, 2, 4, 6 and 8 μM for I; 0, 2, 4, 8 and 12 μM for II, and 0, 4, 8, 12 and 16 μM for III). Cresolase reactions were measured by depletion of p-coumaric acid for 10 min at its λmax = 288 nm and enzyme concentration of 17.7 μM, 60 unit/ml. The reactions were followed using eight different fixed concentrations of substrate (20–160 μM) in different concentrations of the inhibitors (0, 0.4, 0.8, 2.5 and 4 μM for I, 0, 0.5, 1.5, 2.5 and 4.5 μM for II, and 0, 0.5, 1, 2.5 and 3 μM for III). All of the assays were repeated at least three times.
Definitions of units were defined by the vender. Accordingly, one unit of cresolase activity is equal to a 0.001 change in the optical density per min of L-tyrosine at 280 nm in 3 ml of the reaction mixture at 25°C and pH = 6.5. Similarly one unit of catecholase activity is equal to a 0.001 change in the optical density of ascorbic acid per min at 265 nm in 3 ml of the reaction mixture at 25 °C and pH = 6.5, when catechol or L-dopa is used as substrate.
Results and discussion
(a, b and c for I, II and III, respectively) shows double reciprocal Lineweaver-Burk plots for the catecholase activity of MT at different fixed concentrations of inhibitors I, II and III. These plots show a set of straight lines which intersect on the left hand side of the vertical axis for each inhibitor, which confirms mixed inhibition. Both values of apparent maximum velocity (V′max) and apparent Michaelis constant (K′m) as well as slopes of these straight lines (K′m/Vmax) can be obtained at different fixed concentrations of each inhibitors. A secondary plot of the slope against the concentration of inhibitor gives a straight line with an abscissa-intercept of − Ki ( a, b and c), and also another secondary plot of the reciprocal apparent maximum velocity against the concentration of inhibitor gives a straight line with an abscissa-intercept of − αKi ( a′, b′ and c′), where Ki is the inhibition constant and α is the interaction factor between the substrate and inhibitor sites. Results for Ki and α values have been summarized in .
Figure 2 Double reciprocal Lineweaver-Burk plots of MT kinetic assays for catecholase reactions of caffeic acid in phosphate buffer, pH = 6.8 and 293 K and 11.8 μM enzyme concentration, in the presence of different fixed concentrations of inhibitor compounds: (a) For I: 0 μM (▪), 2 μM (▪), 4 μM (▴), 6 μM ( × ), 8 μM (


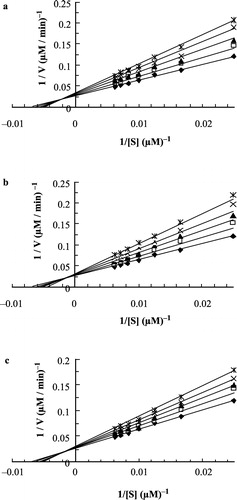
Figure 3 The secondary plots of the slopes in figure 2 (a-c) against the concentration of inhibitor, [I], gives a straight line with an abscissa-intercept of -Ki, inhibition constant for: (a) I; (b) II; and (c) III compounds. Another secondary plot of the reciprocal apparent maximum velocity against the [I] gives a straight line with an abscissa-intercept of − α Ki. α is the interaction factor for: (á) I; (b′) II; and (ć) III compounds.
![Figure 3 The secondary plots of the slopes in figure 2 (a-c) against the concentration of inhibitor, [I], gives a straight line with an abscissa-intercept of -Ki, inhibition constant for: (a) I; (b) II; and (c) III compounds. Another secondary plot of the reciprocal apparent maximum velocity against the [I] gives a straight line with an abscissa-intercept of − α Ki. α is the interaction factor for: (á) I; (b′) II; and (ć) III compounds.](/cms/asset/055adbba-fbb9-4d1b-b208-7f2c23d714b4/ienz_a_117973_f0003_b.jpg)
Table I. Inhibitory constants for compounds I, II and III in catecholase and cresolase reactions.
Double reciprocal Lineweaver-Burk plots for the cresolase activity of MT in the presence of each inhibitor (I, II and III) are shown in (a, b and c for I, II and III, respectively). A series of straight lines intersecting exactly on the vertical axis, the value of maximum velocity is unchanged by the inhibitor but the K′m value is increased, which confirms competitive inhibition of MT. The slope values at each concentration of inhibitor were obtained from and plotted versus concentration of inhibitor to give a secondary plot (see inset in ), from which the inhibition constants (Ki) were obtained from the abscissa-intercept. Results for the Ki values have been summarized in .
Figure 4 Double reciprocal Lineweaver-Burk plots of MT kinetic assays for cresolase reactions with p-coumaric acid in phosphate buffer, pH = 6.8, 293K and 17.7 μM enzyme concentration, in the presence of different fixed concentrations of inhibitor compounds: (a) For I: 0 μM (▪), 0.4 μM (▪), 0.8 μM (▴), 2.5 μM ( × ), 4 μM (


![Figure 4 Double reciprocal Lineweaver-Burk plots of MT kinetic assays for cresolase reactions with p-coumaric acid in phosphate buffer, pH = 6.8, 293K and 17.7 μM enzyme concentration, in the presence of different fixed concentrations of inhibitor compounds: (a) For I: 0 μM (▪), 0.4 μM (▪), 0.8 μM (▴), 2.5 μM ( × ), 4 μM (Display full size); (b) For II: 0 μM (▪), 0.5 μM (▪), 1.5 μM (▴), 2.5 μM ( × ), 4.5 μM(Display full size) and (c) For III: 0 μM (▪), 0.5 μM (▪), 1 μM (▴), 2.5 μM ( × ), 3 μM (Display full size). Insets: The secondary plots of straight line slopes against [I] is shown; [I] is inhibitor concentration, and abscissa-intercept is -Ki.](/cms/asset/aa65068d-a877-4b84-8d61-cbb3f9c984b5/ienz_a_117973_f0004_b.jpg)
First, comparison of these Ki values () with other common inhibitors of MT reveals that they are among the most potent inhibitors for both cresolase and catecholase activities of the enzyme. However, Ki values for cresolase activity of these compounds are less than those for catecholase activity which means more potent the inhibitory effect of these synthetic compounds on the cresolase activity of MT. Moreover, Ki values increase in magnitude as the length of the aliphatic tail increases for these compounds which means that a shorter tail gives a more potent inhibitor. Also, binding of these compounds has occurred at the active site of the enzyme, which prevents binding of the substrate for cresolase activity of the enzyme; i.e. competitive inhibition. However, mixed inhibition occurred for the catecholase activity of the enzyme in the presence of these compounds; i.e. both substrate and inhibitor can be bound to the enzyme with negative cooperativity for binding in their sites (α>1). The negative cooperativity for the binding of substrate and inhibitor in their sites increases as the length of aliphatic tail in these compounds increases.
Some thiol compounds act as inhibitors of tyrosinase due to their ability to chelate Cu2+[Citation37]. Comparison of compounds I, II and III with other sulfur containing tyrosinase inhibitors and the manner of their inhibition, let us to considering them as copper chelators. Their polar head as electron donor may be acts as a Cu2+ chelator by coordination of one sulfur atom. Monophenol and diphenol substrates have been proposed to bind in different fashions to the binuclear copper site of tyrosinase. Monophenols bind to one copper ion via their phenolate oxygen while diphenols coordinate to the binuclear site through both oxygens, thereby forming a bridged structure [Citation47]. It is proposed that, there is a physical difference in the docking of mono- and o-diphenols to the tyrosinase active site and that this difference would be an essential determinant for the course of the catalytic cycle. Thus monophenols would dock to CuA and o-diphenols to CuB due the orientation of one of their hydroxyl groups [Citation48]. It is probable that compounds I, II and III coordinate to CuA in the binuclear active site, thus, show a competitive manner of inhibition of cresolase activity. In the case of catecholase activity mixed inhibition shows that inhibitors can bind both with the free enzyme (E), and enzyme-substrate (ES) complex. In this case diphenol substrates, by the above stated manner of docking may bind to CuB, while the inhibitor coordinates to the CuA site. Besides, monophenols combine only with oxytyrosinase and diphenols with oxy and met forms [Citation47]. Moreover, if inhibitors show a prefered binding pattern to the oxy form, the mixed inhibition mode in cathecholase activity may result from the reaction stoichiometry. From another point of view, the n-alkyl tails of compounds I, II and III may be responsible for different inhibition constants. Several instances show different effects of substrate/inhibitors specificity of tyrosinase through various substitutions of polyphenols and aromatic carboxylic acids [Citation49,Citation50]. Aliphatic dicarboxylic acids ranging between C8 and C13 have been studied as inhibitors of polyphenoloxidase [Citation51]. In gallic acid, increasing the length of long chain esters or hydrophobicity provides greater resistant to oxidation due to disruption of the tertiary structure of the enzyme [Citation52]. The availability of crystallographic data of hemocyanins and recently of sweet potato catechol oxidase shows that in the model of the three-dimensional structure of the tyrosinase family, the catalytic copper center is accommodated in a central four-helix-bundle located in a hydrophobic pocket close to the surface [Citation53]. Ki is a dissociation constant of the enzyme-inhibitor complex and increasing its value by increasing the length of the aliphatic tail of the inhibitor needs more structural investigation by considering the hydrophobic pocket.
Our work described here may assist future aims in to the design of inhibitors to prevent undesirable fruit browning in vegetables or as color skin modulators in mammals.
Acknowledgements
The financial support provided by the Research Council of the University of Tehran is gratefully acknowledged.
References
- Mason HS. Mechanisms of oxygen metabolism. Advan Enzymol. FF Nord. Academic Press, NewYork 1957; 79–234
- Yasunobu KT. Pigment cell biology, M Gordon. Academic Press, NewYork 1959; 583
- Raper HS. The anaerobic oxidases. Physiol Rev 1928; 8: 245–282
- Martinez MV, Whitaker JR. The biochmistry and control of enzymatic browning. Trends Food Sci Technol 1995; 6: 195–200
- Espin JC, Jolivet S, Wichers HJ. Kinetic study of the oxidation of (L-glutaminyl-4-hydroxybenzene catalyzed by Agaricus Bisporus tyrosinase. 1999; 47: 3495–3502
- Fenoll LG, Rodriguez-Lopez JN, Garcia-Sevilla F, Garcia-Ruis PA, Varon A, Garcia-Canovas F, Tudela J. Analysis and interpretation of the action mechanism of mushroom tyrosinase on monophenols and diphenols generating highly unstable o-quinone. Biochim Biophys Acta 2001; 1545: 1–22
- Van Gelder CWG, Flurkey WH, Wichers HJ. Sequence and structural features of plant and fungal tyrosinase. Phytochemistry 1997; 45: 1309–1323
- Strothkemp KJ, Jolley RL, Mason HS. Quaternary structure of mushroom tyrosinase. Biochem Biophys Res Commun 1976; 70: 519–524
- Yong G, Leone C, Strothkemp KJ. Agaricus bisporus metapotyrosinase: Preparation, characterization, and conversion to mixed-metal derivatives of the binuclear site. Biochemistry 1990; 29: 9684–9690
- Jackman MP, Hajnal A, Lerch K. Albino mutants of Streptomyces glaucescens tyrosinase. Biochem J 1991; 274: 707–713
- Taylor SL, Bush RK. Sulfites as food ingredients. Food Technol 1986; 40: 47–52
- Ohyama Y, Mishima Y. Melanogenesis inhibitory effects of kojic acid and its action mechanism. Fragrance J 1990; 6: 53
- Palumbo A, d'Ischia M, Misuraca G, Parota G. Mechanism of inhibition of Melanogenesis by hydroquinone. Biochim Biophys Acta 1991; 1073: 85–90
- Maeda K, Fukuda M. In vitro effectiveness of several whitening cosmetic components in human melanocytes. J Soc Cosmet Chem 1991; 42: 361–368
- Friedman M. Food browning and its prevention: An overview. J Agric Food Chem 1996; 44: 631–653
- Update: Dermatology in general medicine, DB Mosher, MA Pathak, TB Fitzpatric. McGraw Hill, New York 1983; 205–225
- Kubo I, Kinst-Hori I. Flovonols from saffron flower: Tyrosinase inhibitory activity and inhibition mechanism. J Agric Food Chem 1999; 47: 4121–4125
- Kubo I, Kinst-Hori I, Ishiguro K, Chaudhuri SK, Sanchez Y, Ogura T. Tyrosinase inhibitory flovonoids from Heterotheca inuloides and their structural functions. Bioorg Med Chem Lett 1994; 4: 1443–1446
- Chen QX, Kubo I. Kinetics of mushroom tyrosinase inhibition by quercetin. J Agric Food Chem 2002; 50: 4108–4112
- Kubo I, Kinst-Hori I, Chaudhuri SK, Kubo Y, Sanchez Y, Ogura T. Flovonols from Heterotheca inuloides: Tyrosinase inhibitory activity and structural criteria. Bioorg Med Chem 2000; 8: 1749–1755
- Kubo I, Kinst-Hori I. Tyrosinase inhibitors from cumin. J Agric Food Chem 1988; 46: 5338–5341
- Goetghebeur M, Kermasha S. Inhibition of polyphenol oxidase by copper-metallothionein from Aspergillus niger. Phytochemistry 1996; 42: 935–940
- Kim YM, Yun J, Lee CK, Lee H, Min KR, Kim Y. Oxyresveratrol and Hydroxystilbene Compounds: Inhibitory effect on tyrosinase and mechanism of action. J Biol Chem 2002; 227: 16340–16344
- Chen JS, Wei C, Rolle RS, Otwell WS, Balban MO, Marshall MR. Inhibitory effect of kojic acid on some plant and crustacean polyphenol oxidases. J Agric Food Chem 1991; 39: 1396–1401
- Chen JS, Wei C, Marshall MR. Inhibition mechanism of kojic acid on polyphenol oxidase. J Agric Food Chem 1991; 39: 1897–1901
- Kahn V. Enzymatic browning and its prevention, CY Lee, JR Whitaker. American chemical Society, Washington DC 1995; 277–294
- Kahn V, Ben-Shalom N, Zakin V. Effect of kojic acid on the oxidation of N-acetyldopamine by mushroom tyrosinase. J Agric Food Chem 1997; 45: 4460–4465
- Cabanes J, Chazarra S, Garcia-Carmona F. Kojic acid, a cosmetic skin agent, is a slow-binding inhibitor of catecholase activity of tyrosinase. J Pharm Pharmacol 1994; 46: 982–985
- Lim JT. Treatment of melasma using kojic acid in a gel containing hydroquinone and glycolic acid. Dermatol Surg 1999; 25: 282–284
- Battaini G, Monzani E, Casella L, Santagostini L, Pagliarin R. Inhibition of the catecholase activity of biomimetic dinuclear copper complexes by kojic acid. J Biol Inorg Chem 2000; 5: 262–268
- Espin JC, Wichers HG. Effect of captopril on mushroom tyrosinase activity in vitro. Biochem Biophys Acta 2001; 1554: 289–300
- Andrawis A, Khan V. Effect of methimazole on the activity of mushroom tyrosinase. Biochem J 1996; 235: 91–96
- Ferrar PH, Walker JRI. Inhibition of diphenoloxidases: A comparative study. J Food Biochem 1996; 20: 15–30
- Seo SY, Sharma VK, Sharma N. Mushroom tyrosinase: Recent Prospects. J Agric Food Chem 2003; 51: 2837–2853
- Pierpoint WS. The enzymic oxidation of chlorogenic acid and some reactions of the quinone produced. Biochem J 1966; 98: 567–580
- Seiji M, Yoshida T, Itakura H, Irimajiri T. Inhibition of melanin formation by sulfhydryl compounds. J Investig Dermatol 1969; 52: 280–286
- Hanlon DP, Shuman S. Copper ion binding and enzyme inhibitory properties of the antithyroid drug methimazole. Experientia 1975; 31: 1005–1006
- Anderson JW. Extraction of enzymes and subcellular organelles from plant tissues. Phytochemistry 1968; 7: 1973–1988
- Palmer JK, Roberts JB. Inhibition of banana polyphenol oxidase by 2-mercaptobensothiazole. Science 1967; 157: 200–201
- Karbassi F, Haghbeen K, Saboury AA, Ranjbar B, Moosavi-Movahedi AA. Activity, structural and stability changes of mushroom tyrosinase by sodium dodecyl sulfate. Colloids and surfaces B: Biointerfaces 2003; 32: 137–143
- Karbassi F, Saboury AA, Haghbeen K, Rezaei-Tavirani M, Ranjbar B. Concomitant calorimetric, spectrophotometric and circular dichroism studies on the impact of sodium dodecyl sulfate on the mushroom tyrosinase structure. Biologia 2004; 59: 319–326
- Saboury AA, Karbassi F, Haghbeen K, Ranjbar B, Moosavi-Movahedi AA, Farzami B. Stability, structural and suicide inactivation changes of Mushroom tyrosinase after acetylation by N-acetylimidazole. Int J Biol Macromol 2004; 34: 257–262
- Shareefi-Borojerdi S, Haghbeen K, Karkhane AA, Fazli M, Saboury AA. Successful resonance raman study of cresolase activity of mushroom tyrosinase. Biochem Biophys Res Commun 2004; 314: 925–930
- Haghbeen K, Saboury AA, Karbassi F. Substrate share in the suicide inactivation of mushroom tyrosinase. Biochim Biophys Acta 2004; 1675: 139–146
- Karbassi F, Saboury AA, Hassan-Khan MT, Iqbal-Choudhary M, Saifi ZS. Mushroom tyrosinase inhibition by two potent uncompetitive inhibitors. J Enz Inhib Med Chem 2004; 19: 349–353
- El- Bayoumi MA, Frieden EA. Spectrophotometric method for the determination of the catecholase activity of tyrosinase and some of its applications. J Am Chem Soc 1957; 79: 4854–4858
- Wilcox DE, Porras AG, Hawang YT, Lerch K, Winker ME, Solomon EI. Substrate analogue binding to coupled binuclear copper active site in tyrosinase. J Am Chem Soc 1985; 107: 4015–4027
- Olivares C, Garcia-Boron JC, Solano F. Identification of active site residues involved in metal cofactor binding and stereospecific substrate recognition in mammalian tyrosinase: Implication to the catalytic cycle. Biochemistry 2002; 41: 679–686
- Menon S, Fleck RW, Yong G, Strothkamp KG. Benzoic acid inhibition of the α, β and γ isosymes of Agaricus bisporus tyrosinase. Arch Biochem Biophys 1990; 280: 27–32
- Kermasha S, Goetghebeur M, Monfette A, Metchet M, Rovelt M. Inhibitory effects of cysteine and aromatic acid on tyrosinase activity. Phytochemistry 1993; 34: 349–353
- Prota G. Melanins and melanogenesis. Academic Press, San Diego 1992
- Kubo I, Kinst-Hori I, Kubo Y, Yamagiwa Y, Kamikawa T, Haraguchi H. Molecular design of antibrowning agents. J Agric Food Chem 2000; 48: 1395–1399
- Klabunde T, Eicken C, Sacchettini JC, Krebs B. Crystal structure of a plant catechol oxidase containing a dicopper center. Nat Struct Biol 1998; 5: 1084–1090