Abstract
The in vitro effect of Al3+ ions in the concentration range 1.7·10− 6 M–8.7·10− 3 M on pepsin activity at pH 2, via kinetic parameters and its electrophoretic mobility was evaluated. Kinetic study demonstrated the existence of an activation effect of Al3+ at pH 2 on pepsin molecule. Kinetic analysis with respect to concentrations of haemoglobin showed that Al3+ ions increase the maximal velocity (Vmax) and kcat values rather than apparent affinity for substrate (KS) implying the non-competitive nature of activation which indicated that aluminium was a non-essential activator of partial non-competitive type. The values of the equilibrium constants KS and KmA for dissociation of corresponding complexes were evaluated as 0.904 ± 0.083 mM and 8.56 ± 0.51 μM, respectively. Dissociation constant KA, of activator from enzyme-activator complex calculated via kinetic and direct measurement of Al3+ binding data, as well as activation constant A50, the activator concentration that gives a rate equal to half at a saturating concentration of activator, were found to be 8.82 ± 0.90 μM, 8.39 ± 0.76 μM, and 8.05 ± 0.48 μM respectively. Native PAGE electrophoresis shows the decrease in electrophoretic mobility of pepsin and confirms modification of the electric charge and conformational changes of pepsin caused by bound Al3+ on the pepsin molecule. Al3+ induced conformational changes of pepsin were verified by UV-VIS and IR spectra. Moreover, the absence of conformational changes in the haemoglobin molecule in the presence of Al3+ ions confirms that the obtained activation is a consequence of conformational changes caused only in the pepsin molecule.
Introduction
Porcine pepsin A (EC 3.4.23.1), a prominent member of the aspartic protease, is the principal proteolytic enzyme of gastric juice and as the best understood of this family of proteinases it was used as a model to study related enzymes. As all aspartic proteinases pepsin molecule consist of two homologous lobes composed predominantly of β-sheets separated by a hinged substrate-binding cleft. Two active site aspartic residues occupy the cleft to which admittance is restricted by a hinged flexible flap region [Citation1–6]. The pepsin consists of 326 residues with molecular weight of 35000 Da and it derives from its zymogene pepsinogen, by removal of 44 amino acids, from its amino terminus, to give a single chain enzyme with a low pI and three disulfide bridges. Each lobe of pepsin contains a catalytic aspartic acid residue Asp32 and Asp215 located at the centre of the binding cleft between the domains. One of two Asp residues has to be protonated and the other deprotonated, for the protein to be active [Citation1]. Due to the catalytic residues, the active pH ranges from 1.0 to 5.0 [Citation7–11]. Because pepsin has been well structurally characterized, it represents an appropriate model to study the effects of metal ions on structure, function and kinetic behaviour [Citation12,13].
Aluminium does not belong to essential elements, and as a non-regulatory ion can be toxic to many organisms. Even it is a most abundant metal, comprising almost 8% of the earth's crust; its concentration in living system is very low. Aluminium can occur in a number of different forms in water, and his chemistry in water solutions is very complex where many chemical parameters, including pH, determine which aluminium species are present in aqueous solutions. Aluminium enters the human body from foods, particularly those containing aluminium compounds used as food additives and from drinking water. An additional source of aluminium is Al-containing antacids that have been widely used in therapy for dyspepsia. Average daily dietary aluminium intake is approximately 2–6 mg Al/day in children and 6–14 mg Al/day in adults, according World Health Organization Technical Reports [Citation14,15].
Aluminium toxicity in patients with renal failure is well documented [Citation16,17]. It has been proposed (with some controversy) as a cofactor in the pathogenesis of Alzheimer's disease, as well as other neurodegenerative pathologies. Accumulated aluminium accelerates process of aggregation of amiloyd beta peptide and causes activation of present secretases, which belong to aspartic proteases family [Citation18–21]. Some authors perform the studies which included small peptide molecules (13 amino acid residues) which sequence corresponds to those founded in proteins of neuron plaques, shows that bounded aluminium ions cause's significant conformational changes and induce increase in beta structure content (conversion alpha to beta up to 90%) [Citation22]. Al3+ ion altered formation and stabilization of beta structure is probably direct consequence of complex formation between Al3+ ion and carboxilate ion in amino acids residues [Citation23]. However, very little knows about whether Al3+ ions, that enter human organism, can affect activity of gastrointestinal enzymes and influence digestion and utilization of nutrients. Aluminium affects the activity of α-chymotrypsin, in the presence of its specific bovine pancreatic trypsin inhibitor; at the pH 6.5 in the presence of aluminium, the enzyme activity is doubled, and the inhibitor is only 1% as effective as in the absence of the metal ion [Citation24]. Recently it has been shown that Al3+ ions inhibited trypsin activity, but do not pepsin. It was observed highest activation of pepsin activity by 191% in the presence of 25 μg Al3+ / mL of reaction mixture [Citation25].
As the mechanism of Al3+ ions on pepsin activity is not still clear, the objective of this study is to investigate the in vitro influence of different concentrations of Al3+ ions, physiological and toxic ones, on pepsin activity. Moreover, extensive kinetic studies were undertaken to determine the nature of the enzyme modulation (type and mechanism) by investigated metal ion. Besides, we compared the electrophoretic mobility in the absence and presence of investigated Al3+ ions.
Materials and methods
Chemicals
Pepsin, lyophilised powder, was purchased from Sigma–Aldrich, and used without further purification. Haemoglobin from bovine blood was purchased from Sigma–Aldrich and was used as substrate. PAGE-reagents were purchased from Sigma–Aldrich. Other chemicals aluminium chloride (AlCl3·6H2O), hydrochloric (HCl), trichloroacetic acid (TCA) and potassium chloride (KBr) were obtained from MERCK, all of reagent grade were prepared prior to use.
Enzyme assay
The Worthington method based on enzyme-catalyzed measured rate of hydrolysis of denatured haemoglobin (Hb) substrate was used for evaluation of enzyme activity in the absence (control) and presence of Al3+ ions [Citation26]. Pepsin activity was determined in an incubation medium containing 1 mL of pepsin solution (20 μg/mL in 0.01M HCl, pH 2), 5 mL haemoglobin solution (2% solution of haemoglobin in 0.01M HCl). The working solutions were incubated for 10 min at 37°C. The reaction was stopped by addition of 10 mL 5% TCA. The absorbance of clear filtrates recorded at 280 nm, and activities were calculated by the equation:
Estimation of kinetic parameters
Kinetic analysis was carried out according to a slightly modified method of Anson [Citation26], by following the initial velocity of the enzymatic reaction in the absence and presence of Al3+ (1.7·10− 6–8.7·10− 3 M) and increasing concentrations of haemoglobin (0.025–4·10− 3 M). All the assays were performed at pH 2. The data analyzed by the software package Origin 6.1 and the results were recalculated using EZ FIT program [Citation27].
Elemental analysis
For direct measurement of Al3+ binding, sample of 5 mL of pepsin solution (3·10− 5 M of in 0.01 M HCl, pH 2), was extensively dialyzed. Solution was placed in dialysis bags (9 mm flat width) with a molecular cut of 12 to 14 kDa (Sigma- Aldrich) and dialysed against 50 mL AlCl3·6H2O solution (0.01 M pH 2), for 36 h at 4°C with gently stirring. All solutions were prepared in distilled-deionised water. Dialysis bags were prepared by boiling and rinsing in distilled-deionised water. The concentration of aluminium ions inside and outside the dialysis bag was carried out by inductively coupled plasma atomic emission spectroscopy (ICP-AES), (Spectroflame ICP, 2.5 kW, 27 MHz). ICP-AES analysis was performed by measuring the intensity of radiation of the specific wavelength emitted by aluminium at 396.152 nm and with a sample flow rate of 1 mL min− 1. Integration times were 1 per increment (i.e., analysis time of 1 minute per sample).
UV absorbance measurements
UV absorbance measurements of pepsin and haemoglobin samples, both in the absence and in the presence of Al3+ (water solutions acidified with 0.01M HCl, pH2), carried out on Beckman UV 5260 UV-VIS spectrophotometer with an electro-thermal temperature control cell unit. The temperature control performed with DANA – Digital voltmeter model 4800 with chromel-alumel thermocouples. A quartz cell with a 1 cm path length was used for all the absorbance studies. All measurements were carried out at 37°C.
IR studies
Pepsin-Al3+ and haemoglobin-Al3+ samples were lyophilized and used for the further experiments. The removal of any traces of water from the samples was insured by drying samples over silica gel over night and heating during 2 hours at 100°C. For infrared spectroscopy, the samples were prepared in the form of standard potassium bromide (KBr) pallet (the ratio between pepsin and KBr and pepsin – Al3+ and KBr were 1:100); the pallets of haemoglobin samples in KBr were prepared in the same manner. Infrared spectra between 4000 and 400 cm− 1 obtained on a Specord 75 IR, Carl Zeiss in double-beam operation vs. KBr as a reference. IR spectra of both proteins in KBr matrices recorded at room temperature (25°C).
Polyacrylamide gel electrophoresis (PAGE)
Native electrophoresis of pepsin and haemoglobin on 10% polyacrylamide gel carried out at 4°C during 90 min, according to the Laemmli procedure, at pH 8.3 [Citation28]. Water solutions of all samples of enzyme (pepsin dissolved in water to final concentration of 2 mg/mL) were titrated with HCl to pH 2 and incubated at 37°C, with addition of different concentrations of Al3+ ion (1, 5 and 10 mM). The samples of pepsin and haemoglobin were diluted with sample buffer in ratio 1:1 (v/v) and applied on gel in volume of 20 μL. Visualization was performed with Commassie Brilliant Blue G-250 dye. The gels scanned and processed using Corel Draw 11.0 software package. Quantification of electrophoretic mobility of the molecule is carried out via RS value, where it is defined by:
Statistical analysis
Graphs were plotted by using Microcal Origin program (version 6.1). Values including KS, Vmax, KmS, KmA, KA, and A50 and their standard errors are presented as means ± SEM (obtained by the linear regression analysis). The statistical comparisons were performed by Students t-test for paired observations. The means of at least five observations was quoted in the text and p < 0.01 was considered statistically significant.
Results
In vitro effects of Al3+ ions on porcine pepsin activity
The influence of Al3+ ions on porcine pepsin activity was investigated in the concentration range 1.7·10− 6 M–8.7·10− 3 M at pH 2. We tested wide range of Al3+ concentration that included physiological as well as toxic doses of metal ion because of lack of similar information in available literature. The effects of chosen concentrations of Al3+ ions on in vitro pepsin activity are presented in .
Figure 1. Relative activity of pepsin in the presence of Al3 + ions. Proteolytic activity of pepsin expressed as percentage depends on Al3 + ions present. The degree of activation is expressed as % of increased activity considering the activity of pepsin in the absence of Al3 + ions as 100%. All results are expressed as a mean percentage of enzyme activity relative to the corresponding control value, from at least three independent experiments performed in triplicate.
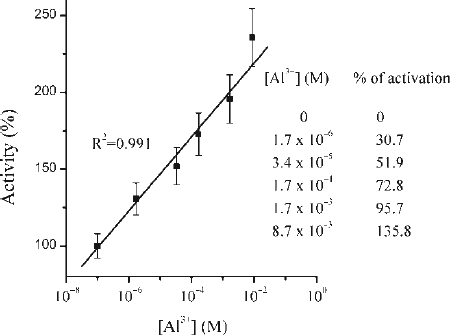
It is obviously from that all investigated concentration of Al3+ ions cause increase of pepsin activity. The increasing concentrations of metal ions induced increase of enzymatic activity. Values are expressed as the percent of increased activity related to the control, which considered as 100%. Aluminium was found to stimulate the enzyme activity in dose-dependent manner and linear curve (the correlation coefficient was 0.991) was obtained and presented on . The presence of 1.7·10− 6 M Al3+ in incubation milieu causes increase in pepsin activity for 30.7%. Increasing the amount of Al3+ led to a more significant increase of proteolytic activity. So, 3.4·10− 5 M of Al3+ increases pepsin activity for 51.9% (p < 0.01), 1.7·10− 4 M for 72.8%, while in the presence of 1.7·10− 3 M Al3+ in incubation milieu the activity of pepsin was doubled (95.7%, p < 0.01). Maximal investigated concentration of Al3+ ions, induce the increase of pepsin activity for 135.8% (p < 0.01), in comparison with corresponding control.
Kinetic analysis
In order to evaluate the nature of porcine pepsin activation induced by Al3+ , kinetic parameters KS and Vmax were determined by varying the concentration of substrate–denatured haemoglobin. The kinetic properties of enzyme were determined in the presence of desired concentration of metal ions (from 1.7·10− 6 to 8.7·10− 3 M, pH 2). The dependence of the initial reaction rate vs. substrate concentration in the presence and the absence of aluminium exhibited typical Michaelis–Menten kinetics that is presented in inset. A double reciprocal plot of the velocity vs. substrate concentrations at increasing concentrations of Al3+ ions resulted in a family of linear plots intersecting at 1/[Hb] axis ().
Figure 2. Lineweaver-Burk plot of a series of kinetics measurements in a presence of different Al3 + ions concentrations at pH2; (inset) Hyperbolic plots (sigmoid fit) representing initial pepsin velocity versus haemoglobin concentration in the absence and presence of different Al3 + ions concentrations at pH2.
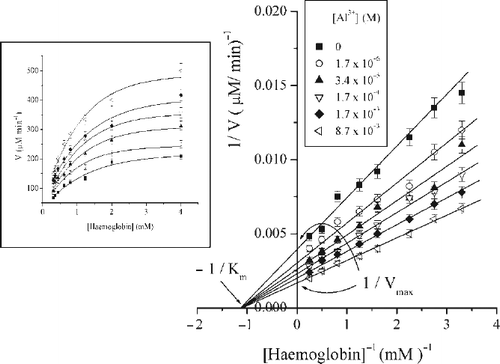
The Lineweaver-Burk plots show that increase of reaction velocity in the presence of Al3+ ions is proportional to increased Al3+ ions concentration. Kinetic constants obtained from Lineaweaver–Burk plot at variety of different activator concentrations, are presented in .
Table I. Influence of Al3 + on Km, Vmax, kcat and catalytic effectiveness of enzyme (kcat Km−1).
As can be seen, increasing of aluminium concentration increased Vmax values, without producing a significant change in the value of apparent enzyme affinity for substrate (KS). The results obtained from Lineweaver-Burk plots, are used for calculation of kinetic constants. The secondary plots of the slopes and intersects vs. activator concentrations are not linear (data not shown), but the reciprocal of the change in slope and intercept (Δslope and Δintercept) that are determined by subtracting the values in the presence of activator from that in its absence, are linear [Citation29,30].
The intercepts of a plot 1/Δslope and 1/Δintercept vs. 1/ [Al3+ ] on 1/Δ axis, and intercepts of both plots on 1/ [Al3+ ] axis are used for calculating equilibrium constants KmS and KmA for dissociation of formed binary enzyme-activator (Al3+ ) and ternary enzyme-activator-substrate complexes (, Scheme ). The calculated values for constants are 0.904 ± 0.083 mM and 8.56 ± 0.51 μM, respectively.
Figure 3. The plots of 1 / (change in slope or intercept), (1/Δ) against 1 / [Al3 + ], where Δ is defined as slope or intercept in the absence of activator (Al3 + ions) minus that in its presence.
![Figure 3. The plots of 1 / (change in slope or intercept), (1/Δ) against 1 / [Al3 + ], where Δ is defined as slope or intercept in the absence of activator (Al3 + ions) minus that in its presence.](/cms/asset/2bb8cbe5-243e-40b3-9f14-ef819fb9ad12/ienz_a_284243_f0003_b.gif)
Scheme 1 Reaction scheme for non-essential activation; Abbreviations are E—enzyme, S—substrate, A—activator, P—product.
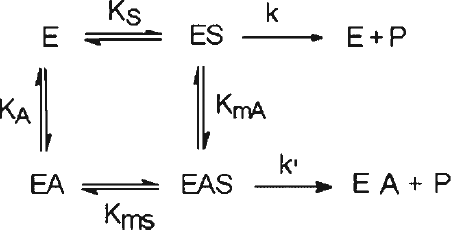
For further comparison of pepsin catalytic behaviour in absence and presence of aluminium the catalytic constant kcat and catalytic effectiveness kcat · KS− 1 were calculated. It could be seen () that increasing concentrations of activator causes the increase of turnover numbers. In the presence of 1.7 μM of Al3+ , the kcat is 3378 ± 81 min− 1, while in the presence of maximal concentration of activator (8.7 mM) kcat is 6112 ± 173 min− 1. In addition, the higher values of catalytic effectiveness were obtained in the presence of Al3+ .
Using UV spectral and aluminium binding assays data (experimental data not shown) the fraction of bound aluminium to pepsin ν and dissociation constant for pepsin-aluminium complex K A, were found to be 0.96 ± 0.09 and 8.39 ± 0.76 μM respectively [Citation31].
Kinetic data was used also for calculating the activator concentration that gives a rate equal to the half at a saturating concentration of activator (A50), and also dissociation constant KA for enzyme–activator complex () [Citation32]. The calculated values of KA and A50 for pepsin–aluminium complex were 8.82 ± 0.90 μM and 8.05 ± 0.48 μM, respectively, that is consistent with a non-essential partial non-competitive activation system (Scheme ).
Influence of Al3+ ions on pepsin and haemoglobin conformation
UV studies
The pepsin-Al3+ and haemoglobin-Al3+ interactions were followed by UV spectra. The absorption spectra of pepsin and haemoglobin in the absence and presence of 10 mM Al3+ are presented in A and B. It is obvious that the characteristic absorption maximum for pepsin at 280 nm is shifted in a presence of Al3+ to 255 nm by formation pepsin – Al3+ complex for all applied concentrations of aluminium (1, 5, 10 mM) and intensity of characteristic peaks have been decreased in a dose dependent manner ( A). However, the presence of Al3+ ions did not induce any shifts of characteristic peaks positions (273 nm and 369 nm) ( B). In UV spectra of haemoglobin, in the presence of all applied concentrations of aluminium (1, 5, 10 mM), intensity of characteristic peaks decreased in a dose dependent manner ( B). A and B shows only UV spectra in a presence of 10 mM Al3+ .
FIG. 5A –UV absorption spectra of pepsin (solid curve) and pepsin treated with 10 mM Al3 + (dotted curve). B - UV absorption spectra of haemoglobin (solid curve) and haemoglobin treated with 10 mM Al3 + (dotted curve). Other spectral curves obtained in a presence of 1 mM and 5 mM Al3 + are not presented because of clarity of the graphs.
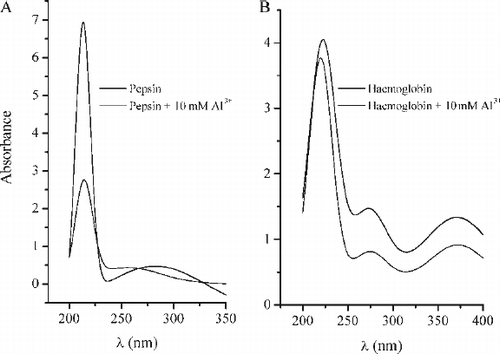
IR studies
IR spectra of pepsin and haemoglobin in KBr matrices in absence (solid curve 1) and in a presence 10 mM Al3+ ions (dotted curve 2) are presented in A and B.
FIG. 6 A–IR spectra of pepsin (solid curve,1) and pepsin in a presence of 10 mM Al3 + ions (dotted curve,2) and B - IR spectra of haemoglobin (solid curve,1) and haemoglobin in a presence of 10 mM Al3 + ions (dotted curve,2). IR spectra were recorded in KBr matrices.
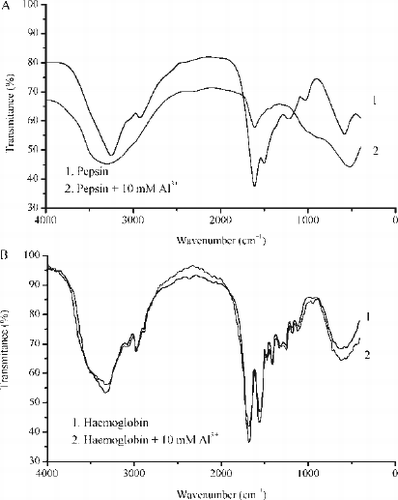
As can be seen, presence of 10 mM Al3+ ion causes noticeable changes in infrared spectra of pepsin. Typical features were: the disappearance of the vibration bands in the 3500–2750 cm− 1 region, especially bands at 3256 and 2920 cm− 1; decrease of intensity of the vibration band at 1600 cm− 1; the disappearance of the vibration bands in the 1500–1200 cm− 1 especially band at 1491 cm− 1 in respect to spectrum of pepsin ( A). However, IR spectra of haemoglobin demonstrate that the treatment with 10 mM Al3+ ions does not cause noticeable changes in its conformation as shown in B. The both spectra of haemoglobin ( B, curve 1) and haemoglobin treated with 10 mM Al3+ ions ( B, curve 2) contain characteristic bands of haemoglobin without changes in its positions and intensity.
Electrophoretic analysis of pepsin and haemoglobin
Native PAGE profiles of untreated and aluminium treated pepsin solutions at pH 2 were studied to verify the conformational changes of pepsin induced by Al3+ ions that resulting in activation effect. Electrophoretic mobility in the presence of Al3+ ions (from 1 to 10 mM) inducing the highest activation (producing around the 100% activation or more upon the enzyme assay data) and in the absence of activator were compared. The electrophoregrams of pepsin and haemoglobin samples in absence or in the presence of different concentrations of Al3+ ion are presented in .
Figure 7. Native PAGE electrophoregram of pepsin (upper) and haemoglobin (lower) without and in a presence of 1, 5 and 10 mM Al3 + at pH 2.
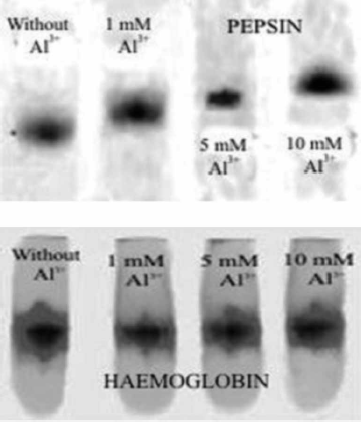
The presence of Al3+ cause the decrease of pepsin electrophoretic mobility at all investigated concentrations. In addition, the degree of decrease is proportional to Al3+ concentrations, which the one has been exposed. In the absence of Al3+ ion, the electrophoretic mobility of pepsin under the physiological conditions the obtained Rs value for pepsin is 0.47 while in the presence of 1, 5 and 10 mM Al3+ ions the obtained Rs values were 0.46, 0.44 and 0.42, respectively. It is obviously that the highest concentration of Al3+ results in the lowest electrophoretic mobility. Electrophoretic mobility of haemoglobin in the absence of Al3+ ion was characterized with Rs value of 0.20, while in the presence of 1, 5 and 10 mM Al3+ ions the obtained Rs values were 0.20, 0.20 and 0.19 respectively. The presence of all investigated concentration of aluminium does not cause changes in electrophoretic mobility of haemoglobin.
Discussion
In the available literature, information about the influence of toxic metal ions on the activity of extra-cellular enzymes is scant and controversial. Variability of data results from the chemical properties of the element and the formation of various chemical species, depending on the pH, ionic strength, presence of competing elements and complexing agents within the gastrointestinal tract. To date, however, there have been no papers about investigations with gastrointestinal enzymes and Al3+ ions and the mechanism of this action.
Aluminium is a toxic ion that is partly absorbed from the mammalian gastrointestinal tract. It was noted that the gastrointestinal fluids have their own intrinsic metal binding properties, especially for copper (2+) and iron (3+). It is known that Al3+ ions interact with Ca2 + and Mg2 + -dependent enzymes. Binding of Al3+ ion with ATP is stronger than with Mg2 + , which is a natural activator of ATP-ase; therefore the Al3+ ion is a strong inhibitor of all ATP-ases. It has been documented that Al3+ inhibits activity of hexokinase, adenylat-cyclase, calmodulin, NAD-kinase and other Mg-dependent enzymes [Citation33]. In addition, there is lack of data referring about mechanism and kinetics of aluminium binding to gastrointestinal fluids, especially to main enzyme of gastric juice–pepsin. In particular, we simulate the effects of Al3+ ions on pepsin activity in vitro conditions.
Results of our study showed that aluminium stimulate pepsin activity. The obtained results are in agreement with previously reported [Citation25] stimulatory effect of Al3+ ions on porcine pepsin activity. The authors reported that applying concentration of 1.1·10− 3 M Al3+ ions induce the activation of 191%, while we obtained 135.8% activation of pepsin activity, in applied concentration of Al3+ ions of 8.7·10− 3 M. The observed disagreements could be explained by differences in experimental conditions (different pH, enzyme/substrate ratio). The obtained activation probably is consequence of changes in conformation of enzyme molecule induced by bonded Al3+ ions. Upon analogy to the other aspartic proteases, it is possible that bounded aluminium ions causes significant conformational changes and induce increase in beta structure content [Citation22,23,34].
As Al3+ ions occur mostly in an ionic form in the acidic milieu (as [Al (H2O)6]3+ ) it probably affect electric charge of the molecules of enzyme and cause the differences in its electrophoretic mobility. The decrease in the electrophoretic mobility of pepsin molecule may be assigned to the change in net electric charge of the pepsin caused by binding of Al3+ ions to corresponding binding sites of pepsin molecule and probably to conformational changes evaluated by UV and IR spectroscopy. The obtained effect is proportional to the increase of Al3+ ions concentration. Overall, it can be concluded that Al3+ ions binding to pepsin molecule induced conformational changes resulting in increased enzyme activity and decrease of electrophoretic mobility.
Analysis of obtained kinetic data [Citation29,35] implies the activation as a non-essential partial non-competitive type. It suggests that aluminium do not influence on substrate binding at specific binding sites on pepsin, but causes conformational changes ( and ) that increase the rate of substrate converting to the reaction products. The results are consistent with a partial activation system (Scheme ), in which the kinetic constants KmS and KmA for dissociation of corresponding binary and ternary complexes can be determined [Citation29].
At equilibrium conditions partially non-competitive activation exists if the following conditions are satisfied, i.e. KS = KmS, KA = KmA and k < k′ (Scheme ) [Citation29,35]. Constant KA was calculated from kinetic and equilibrium binding assay data; the obtained values are in good agreement to each other, and are 8.82 ± 0.90 μM and 8.39 ± 0.76 μM respectively. Other constants, KmA = 8.56 ± 0.51 μM for binding of aluminium to pepsin-haemoglobin complex and KmS = 0.904 0.76 ± 0.083 mM for binding of haemoglobin to pepsin-aluminium complex are in agreement with proposed conditions for partially non-competitive activation mechanism.
At the same time bound Al3+ could induce conformational changes of enzyme responsible for enzyme ability to convert substrate to product (increase in Vmax) rather than apparent affinity for substrate (KS) obtained by kinetic analyses.
In summary, the present kinetic analyses, which classify this phenomenon as a case of non-essential activation, indicated that the activation by Al3+ ions was of partial non-competitive type.
Acknowledgements
Especial thanks are due to N. Miljevic, Ph. D. and M. Stoiljkovic, Ph. D. from Vinca- Institute of Nuclear Sciences, Belgrade, Serbia for valuable discussions during the development of this paper. This study is a part of Project No 142025, Ministry of Science and Environmental Protection of the Republic of Serbia.
References
- J Tang, and RNS Wang. (1987). Evolution in the structure and function of aspartic proteases. J Cell Biochem 33:53–63.
- NS Andreeva, AS Zdanov, AA Fedorov, AE Gushchina, and NE Shutskever. (1984). X-ray analysis of pepsin. VI. Atomic structure of the enzyme at 2-angstrom resolution. Mol Biol 18:313–322.
- ET Baldwin, TN Bhat, S Gulnik, MV Hosur, RC Sowder, RE Cachau, J Collins, AM Silva, and JW Erickson. (1993). Crystal structures of native and inhibited forms of human cathepsin D: Implications for lysosomal targeting and drug design. Proc Nat Acad Sci USA 90:6796–6800.
- TL Blundell, J Jenkin, BT Sewell, LH Pearl, JB Cooper, IJ Tickle, B Veerapandian, and SP Wood. (1990). X-ray analyses of aspartic proteinases. The three-dimensional structure at 2.1 A resolution of endothiapepsin. J Mol Biol 211:919–941.
- VB Pedersen, and B Foltmann. (1973). The amino acid sequence of a hitherto unobserved segment from porcine pepsinogen preceding the N-terminus of pepsin. FEBS Lett 35:250–258.
- P Sepulveda, J Marciniszyn, D Lui, and J Tang. (1975). Primary structure of porcine pepsin. III. Amino acid sequence of a cyanogens bromide fragment, CB2A, and the complete structure of porcine pepsin. J Biol Chem 250:5082–5088.
- AR Sielecki, AA Fedorov, A Boodho, A Andreeva, and MNG James. (1990). Molecular and crystal structures of monoclinic porcine pepsin refined at 1.8 Å resolutions. J Mol Biol 214:43–170.
- JB Cooper, G Khan, G Taylor, IJ Tickle, and TL Blundell. (1990). X-ray analyses of aspartic proteinases. II. Three-dimensional structure of the hexagonal crystal form of porcine pepsin at 2.3 Å resolution. J Mol Biol 214:199–222.
- A Wlodawer, and JW Erickson. (1993). Structure-based inhibitors of HIV-1 protease. Ann Rev Biochem 62:543–585.
- BM Dunn, M Jimenez, BF Parten, MJ Valler, CE Rolph, and J Kay. (1988). A systematic series of synthetic chromogenic substrates for aspartic proteinases. Biochemistry 27:4827–4834.
- T Kageyama, and K Takahashi. (1983). Occurrence of two different pathways in the activation of porcine pepsinogen to pepsin. J Biochem 93:743–754.
- J Kay. (1985). Aspartic proteinases and their inhibitors. Biochem Soc Trans 13:1027–1029.
- P Mc Phie. (1975). The origin of the alkaline inactivation of pepsinogen. Biochemistry 14:5253–5256.
- Evaluation of Certain Food Additives and Contaminants. WHO Technical Reports 1982; Series No 683.
- Evaluation of Certain Food Additives and Contaminants. Who Technical Reports 1989; Series No 776.
- M Gomez, JL Domingo, D Del Castillo, JM Llobet, and J Corbella. (1994). Comparative aluminium mobilizing actions of several chelators in aluminium-loaded ureamic rats. Hum Exp Toxicol 13:135–139.
- DNS Kerr, and MK Ward. (1988). Aluminium intoxication: History of its clinical recognition and management. In: H Sigel, and A Sigel. Metal ions in biological systems: Aluminium and its role in biology. New York: Marcel Dekker, 217–258.
- VB Gupta, S Anitha, ML Hegde, L Zecca, RM Garruto, R Ravid, SK Shankar, R Stein, P Shanmugavrlu, and KS Jagannatha Rao. (2005). Aluminium in Alzheimer's disease: Are we still at a crossroad?. Cell Mol Life Sci 62:143–158.
- P Zatta, P Zambenedetti, and M Milanese. (1999). Activation of monoamine oxidase type-B by aluminium in rat brain homogenate. Neuroreport 10:1–4.
- P Zatta, P Zambenedetti, V Bruna, and B Filippi. (1994). Activation of acetylcholinesterase by Al(III): Relevance of the metal speciation. Neuroreport 5:1777–1780.
- J. Yanolatas. The Beta amyloid peptide, the gamma secretase complex, and their implications in familial Alzheimer's disease 2004. Available from http://www.serendip.brynmawr.edu.
- TP Flaten, and RM Garruto. (1992). Polynuclear ions in aluminium toxicity. J Theoret Biol 156:129–132.
- EE Bittar, Z Xiang, and YP Huang. (1992). Citrate as an aluminium chelator and positive effector of the sodium efflux in single barnacle muscle fibers. Biochim Biophys Acta 1108:210–214.
- M Clauberg, and JG Joshi. (1993). Regulation of serine protease activity by aluminium: Implications for Alzheimer disease. Proc Nat Acad Sci 90:1009–1012.
- Z Krejpcio, and RW Wojciak. (2002). The influence of Al3+ ions on pepsin and trypsin activity in vitro. Pol J Environ stud 11:251–254.
- M Anson. (1938). The estimation of pepsin, trypsin, papain and cathepsin with hemoglobin. J Gen Physiol 22:79–89.
- WF Perrela. (1988). A Practical Curve Fitting microcomputer program for the analysis of kinetic data on IBM-PC compatible computers. Anal Biochem 174:437–447.
- Laemmli. (1970). Cleavage of structural proteins during the assembly of the head of bacteriophage. Nature 227:680–685.
- M Dixon, and EC Webb. (1979). Enzymes. 3rd ed. London: Longmans, 339–398.
- IH Segel. (1975). Enzyme kinetics. New York: John Wiley & Sons, 227–272.
- DL Nelson, and MM Cox. (2005). Lehninger principles of biochemistry. 4th ed. New York: WH Freeman and Co., 160–161.
- A Cortes, M Cascante, ML Cardenas, and A Cornish-Bowden. (2001). Relationships between inhibition constants, inhibitor concentrations for 50% inhibition and types of inhibition: New ways of analysing data. Biochem J 357:263–268.
- JJ Powell, R Jugdaohsingh, A Piotrowicz, KN White, CR Mc Crohan, and RPH Thompson. (2004). Application of critical precipitation assay to complex samples: Aluminium binding capacity of human gastrointestinal fluids. Chem Spec Bioavailability 16:7–104.
- JT Cottrell, LJ Harris, T Tanaka, and RY Yada. (1997). The sole lysine residue in porcine pepsin works as a key residue for catalysis and conformational flexibility. J Biol Chem 270:19974–19978.
- R Fontes, JM Ribeir, and A Sillero. (2000). Inhibition and activation of enyzmes. The effects on modifier on reaction rate and on kinetic parameters. Acta Biochim Pol 47:233–257.