Abstract
The inhibitory effects of methoxyisobutylisonitrile (MIBI), diethylene triamine pentaacetic acid (DTPA), dimercaptosuccinic acid (DMSA) and metilendifosfonat (MDP) on human erythrocyte glucose 6-phosphate dehydrogenase (hG6PD) activity were investigated. For this purpose, hG6PD was initially purified 557-fold at a yield of 51.43% using 2′,5′-adenosine diphosphate (ADP) sepharose 4B affinity gel chromatography. The in vitro effects of these chelators on hG6PD enzyme were studied. IC50 values of MIBI, DTPA, DMSA and MDP were 0.056, 0.172, 0.274 and 0.175 mM, of hG6PD, respectively. It was detected in in vitro studies that the hG6PD enzyme is inhibited due to these radiopharmaceutical chelators. In addition to in vitro studies, in order to better understand the molecular mechanism of studied compounds, combined in silico approaches, including molecular docking and molecular dynamics (MD), simulations were successfully performed. MD simulations shed light on inhibition mechanisms of the individual inhibitors into the ligand-binding pocket of hG6PD. Essential amino acids for binding are also investigated using per-residue interaction analysis studies.
Introduction
Glucose-6-phosphate dehydrogenase (D-glucose-6-phosphate: NADP+ oxidoreductase, EC 1.1.1.49; G6PD) is a critical enzyme in mammalian erythrocytes that catalyzes the first reaction and rate-limiting enzyme in the pentose phosphate pathway (PPP)Citation1,Citation2. The main physiological function of G6PD is the production of NADPH and ribose 5-phosphate, which are essential for reductive biosynthesis and nucleic acid synthesis ()Citation3. G6PD abnormalities affect as much as 7.5% of the global population, with a wide range of occurrence based on geographic distribution. These abnormalities occur less than 0.1% of certain Japanese populations to more than 35% in some African and European populationsCitation4. NADP+ is reduced to NADPH by glutathione reductase (glutathione: NADP+ oxidoreductase, EC 1.8.1.7; GR) in erythrocytes using glutathione as substrate. Glutathione prevents hemoglobin denaturation, preserves the integrity of red blood cell membrane sulfhydryl groups and detoxifies hydrogen peroxide and oxygen radicals in the erythrocytesCitation5–7. A decrease in G6PD may result in deficiencies of NADPH and reduced glutathione in erythrocytes and a scarcity of reduced glutathione in erythrocytes causes their early hemolysis in the spleenCitation8,Citation9.
Figure 1. Pentose phosphate pathway (PPP) and glutathione coupling: Glucose-6-phosphate dehydrogenase (G6PD), 6-phosphogluconate dehydrogenase (6PGD), glutathione reductase (GR), glutathione (GSH), oxidized glutathione (GSSG), glutathione peroxidase (GPx).
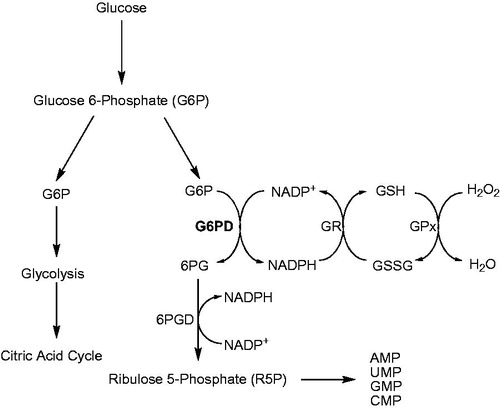
Knowledge of the physiological and biochemical mechanisms that account for the myocardial localization of these agents is important for two reasons. First, an elucidation of the mechanisms of uptake, distribution and retention of these agents is important for the better understanding of the diagnostic information that each can provide. Second, understanding cationic fluxes in the heart can provide an important design strategy for new radiopharmaceuticalsCitation10. There are numerous radiopharmaceutical nuclides used in diagnostic nuclear medicine. However, at present, 99mTc is used in about 90% of all scintigraphic examinationsCitation11. One of the most important diagnostic technique in nuclear medicine for cardiology is the examination of myocardal perfusion for the diagnosis of infarction and ischemia. Methoxyisobutyl isocyanide (MIBI) is the compound used in heart scintigraphy with 99mTcCitation11 and each MIBI acts as monodentally, so that six MIBI molecules are needed to form a hexa MIBI complex with single 99mTc cationsCitation12. The complex will be cationic and be absorbed into the parenchymal cells of the heart myocardiumCitation13. Dynamic kidney scintigraphy, renography and determination of glomerular filtration rate (GFR) can be performed using 99mTc-diethylene triaminepentaacetic acid (DTPA). This means that the physiological events in the kidneys can be studied. 99mTc-DTPA is passed through the kidneys without any binding to kidney tissue. Kidney localization and conditions like infarction can be determined by the use of dimercaptosuccinic acid (DMSA)Citation11. One of the most common examinations in nuclear medicine and oncology is the bone scan for metastases from prostate and breast cancers. The chelator used in bone scintigraphy is methylenediphosphonate (MDP)Citation14. Many radiopharmaceuticals are now being used in therapies.
However, the effects of MIBI, DTPA, DMSA and MDP have not been analyzed on activities of hG6PD. Therefore, the in vitro effects of these radiopharmaceuticals on hG6PD purified from human erythrocytes were investigated in this study. Using the obtained IC50 values, undesirable side effects on G6PD activity and body metabolism can be diminished in used therapies.
Materials and methods
Materials
2′,5′-Adenosine diphosphate (ADP) sepharose 4B was obtained from Pharmacia. NADP+, glucose-6-phosphate, protein assay reagent and chemicals for electrophoresis were purchased from Sigma. All other chemicals used were of analytical grade and were purchased from Merck. MIBI, DTPA, DMSA and MDP were obtained from Eczacıbaşı Monrol (Turkey).
Preparation of hemolysate
Fresh human blood collected in eppendorf tubes with ethylenediamine tetraacetic acid (EDTA) was centrifuged at 2500xg for 15 min and the plasma and leukocyte coat were removed. The packed of red cells were washed with KCl solution (0.16 M) three times and pelleted each time by centrifuged at 2500xg. The supernatants were discarded. The pelleted erythrocytes were hemolysed with 5 vol. of ice-cold water and centrifuged at 4 °C at 10 000×g for 30 min to remove the ghosts and any remaining intact cellsCitation6,Citation8. Ammonium sulfate was slowly added and the fraction between 35% and 65% saturation was collected and centrifuged at 5000xg for 15 min. the pellet was dissolved in 50 mM phosphate buffer (pH 7.0), then dialyzed at 4 °C against 50 mM K-acetate/50 mM K-phosphate buffer (pH 7.0) for 2 h with two changes of buffer.Citation9
Purification of hG6PD by affinity chromatography
Dialyzed sample was loaded onto a 2′,5′-ADP sepharose 4B affinity column and the gel was washed with 25 mL of 0.1 M K-acetate/0.1 M K-phosphate (pH 6.0), followed by 25 mL of 0.1 M K-acetate/0.1 M K-phosphate (pH 7.85) and finally with 0.1 M KCl/0.1 M K-phosphate (pH 7.85). Elution was carried out with an elution buffer (80 mM K-phosphate + 80 mM KCl + 0.5 mM NADP+ + 10 mM EDTA, pH 7.85) at a flow rate of 20 mL/h. Eluate fractions were collected in 2-mL tubes and the enzyme activity in each was calculated separately. All of the purification procedures were performed at 4 °CCitation15. Quantitative protein determination was carried out with Bradford’s method using bovine serum albumin as a standardCitation16. The purified enzyme was run on a SDS polyacryamide gel electrophoresis gel (0.1% SDS) according to Laemmli’s methodCitation17 using a 4% stacking gel and a 10% running gel.
Measurements of hG6PD activity
hG6PD activity assays were run at 25 °C according to Beutler’s methods, which depends on the reduction of NADP+ by hG6PD, in the presence of glucose 6-phosphate. For this spectrophotometric measurement, the reaction medium contained 0.1 mM Tris-HCl (pH 8.5) with 0.2 mM NADP+ and 0.6 mM (G6P) in a total volume of 1 mL. One unit of enzyme (EU) activity was defined as the amount of enzyme that reduced 1 μmol NADP+ per minute at pH 8.5Citation18.
Inhibitor study
MIBI, DTPA, DMSA and MDP were used as inhibitors. In the media with or without inhibitor, the substrate (G6P) concentrations were 0.012, 0.024, 0.036, 0.048 and 0.06 mM. Inhibitor (radiopharmaceutical chelator) solutions were added to the reaction medium, resulting in three different fixed concentrations of inhibitors in 1 mL of total reaction volume. In order to determine IC50 values, activities were calculated with a 0.60 mM constant substrate (G6P) and different inhibitor concentrations. Cuvette activity in the absence of inhibitor was acknowledged to be 100%. Regression analysis graphs were drawn using inhibition% values by a statistical package on a computer. The inhibitor concentrations causing up to 50% inhibition (IC50) were determined from the graphsCitation2,Citation19.
Atomistic in silico approaches
System setup
X-ray structure of human glucose-6-phosphate dehydrogenase complexed with glucose-6-phosphate was retrieved from the Protein Data Bank (PDB ID: 2BHL)Citation20. The structure was repaired and prepared via following of consequent steps; building of missing side chains, assignment of bond orders, adding hydrogen atoms and keeping of the crystallized water molecules within 5 Å of the active site, assigned by the cocrystallized ligand in PBD file. The constructed system was assigned at the physiological pH (pH = 7.4) and then minimized using molecular mechanic (MM) methods with the force field of OPLS 2005Citation21. The obtained system from process above, were implemented in atomistic simulations, carried out in current calculations.
Ligand data set
Two-dimensional (2D) structures of the used compounds are sketched in Maestro molecular modeling package of the Schrodinger.Citation22 Protonation states of studied compounds are determined and these molecules are optimized in the physiological pH using LigPrep moduleCitation23. Conformational search is also performed for each compound to find the lowest energy conformer.
Molecular docking simulations
Docking protocol of GlideCitation24 and also Prime moduleCitation25 implemented in Schrodinger Suit were used to perform a flexible-receptor docking study. Induced fit docking (IFD) module of Schrodinger SuitCitation26 employs Glide and Prime protocols to carry out this calculation. This approach help to predict interaction energy (docking score) of studied compounds and also conformations of small molecules at the binding pocket of the target structure. IFD consists of following consequent steps; (i) docking of ligand at the binding pocket of the protein, (ii) refining of atoms of amino acids (backbone and side-chain atoms) within 5 Å of docked ligand, (iii) redocking of ligand into the active site.
Molecular dynamics (MD) simulations
Classical molecular dynamics (MD) simulations were implemented to investigate the time-dependent conformational changes of complex structures and per-residue interaction energy analyses. HG6PD in complexes with DTPA, DMSA and MDP were considered, and the three complexes (initial conformers were retrieved from the outputs of IFD poses and a control system (ligand-free protein; apo state) were submitted in MD simulations. Four atomistic MD simulations were independently carried out using Desmond codeCitation27. All systems were solvated by SPC water model and then naturalized. The thickness of water layer was set to 10 Å. Before the production MD run, the systems were minimized with a maximum iteration of 2000 steps. Then, each of the systems were submitted in a 10 ns MD simulations. Temperature and pressure were assigned on 300 K and 1.01325 bar, respectively, using isothermal–isobaric (NPT) ensemble. Nose-Hoover chain and Martyna–Tobias–Kleinwas were implemented thermostat and barostat methods, respectively. Cutoff radios of 9 Å and long-range method of smooth Particle Mesh Ewald (PME) were used for Coulomb interactions.
Results and discussion
Worldwide, G6PD deficiency is frequently seen in African, Mediterranean, Middle East and far East nations and their lineages, with a frequency ranging from 5% to 40%Citation28. The use of pamaquine in malaria therapy resulted in same severe side effects, including dark color urine and anemia in some patients. Along with these outward symptoms, G6PD deficiency was also determined in these individuals. Use of this and a number of other drugs cause the hemolysis that is connected with complications. These data showed that enzymes and related reactions were important for the maintenance of lifeCitation29.
GSH is used in antioxidant defence mechanisms and its production requires that NADPH be synthesized in the pentose phosphate metabolic pathway in which G6PD and 6PGD participateCitation19–30. Inhibitory effects of many drugs and other chemicals on G6PD enzyme activities in different animal species and human beings have been reported in many investigations. For example, thallium-201, thiamphenicol, amikacin, gentamycin, netilmycin, chloramine-T and CuSO4 can inhibit erythrocyte G6PD activityCitation2,Citation9,Citation15. In other studies, anesthetics inhibited hGR and carbonic anhydrase (CA) activity in the human erythrocyteCitation31–34. Ciftci et al. investigated effects of some antibiotics on human erythrocyte G6PD and these antibiotic IC50 values for 2.9 to 125 mMCitation15.
As shown in , the specific activity of the purified hG6PD was 5.68 EU/mg proteins, which represented a yield of 51.43% and a purification of 557-fold. The IC50 value for MIBI, DTPA, DMSA and MDP were 0.056, 0.172, 0.274 and 0.175 mM, of hG6PD, respectively (). The IC50 graphics showed in .
Figure 2. Activity% versus [MIBI] regression analysis graphs for hG6PD in the presence of seven different MIBI concentrations.
![Figure 2. Activity% versus [MIBI] regression analysis graphs for hG6PD in the presence of seven different MIBI concentrations.](/cms/asset/1caf0453-39a8-43b4-b414-97018f0efb07/ienz_a_1178735_f0002_c.jpg)
Figure 3. Activity% versus [DTPA] regression analysis graphs for hG6PD in the presence of seven different DTPA concentrations.
![Figure 3. Activity% versus [DTPA] regression analysis graphs for hG6PD in the presence of seven different DTPA concentrations.](/cms/asset/e21d49aa-9d57-491a-a0a4-5456ca234ecc/ienz_a_1178735_f0003_c.jpg)
Figure 4. Activity% versus [DMSA] regression analysis graphs for hG6PD in the presence of six different DMSA concentrations.
![Figure 4. Activity% versus [DMSA] regression analysis graphs for hG6PD in the presence of six different DMSA concentrations.](/cms/asset/dea3cfe0-48c0-412a-bc2d-4ee0a75bc283/ienz_a_1178735_f0004_c.jpg)
Figure 5. Activity% versus [MDP] regression analysis graphs for hG6PD in the presence of six different MDP concentrations.
![Figure 5. Activity% versus [MDP] regression analysis graphs for hG6PD in the presence of six different MDP concentrations.](/cms/asset/d58b5764-2499-467a-bdf2-39cdbcc42f12/ienz_a_1178735_f0005_c.jpg)
Table 1. Purification scheme of hG6PD by 2′,5′-ADP sepharose 4B affinity gel chromatography.
Table 2. IC50 and predicated binding energy values of hG6PD inhibitors.
Molecular modeling and simulations
Docking and atomistic MD simulations approved as informative approaches, profoundly provided to track and pursue the treatments of protein and small moleculesCitation35–45. Conformational stabilities and transitions, protein–ligand interactions (hydrogen bonding, hydrophobic interactions, salt bridges, etc.) and prediction of interaction energies of ligands, can be investigated using molecular-modeling applications. shows predicted interaction energies of studied compounds DTPA, DMSA and MDP using IFD method (docking pose of MIBI compound could not obtained using IFD methods). Docking scores of DTPA, DMSA and MDP molecules are founds as −8.821, −9.061 and −8.214 kcal/mole, respectively.
In order to better monitor and understand the inhibition mechanism of each compound and how the inhibitors influence the structure of protein upon binding at the active site, the complexes, derived from IFD study were submitted in 10 ns MD simulations.
As seen in (upper panel), the flexibility degree of hG6PD (Cα atoms were included) upon binding to DTPA, DMSA and MDP were evaluated throughout the simulations. Ligand-free system revealed higher mobility compared to holo conformers. HG6PD in complexes with MDP and DTPA were fluctuating in stable conditions and converging after 4 ns. In addition, fluctuations of ligands at the active site based on their heavy atoms are investigated using root mean square deviation (RMSD) analysis in (lower panel). DMSA shows different RMSD profile throughout the simulations compared to other two studied compounds DTPA and MDP due to its unique inhibition mechanism.
Figure 6. RMSD evolution of Cα of hG6PD in complexes with MDP, DTPA and DMSA compounds, and control system (upper panel) and also heavy atoms of three ligands into the active site (lower panel) during 10 ns MD simulation.
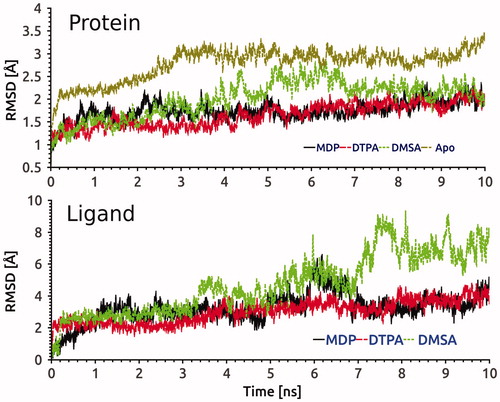
Protein–ligand interaction analysis of MDP, DTPA and DMSA into the ligand-binding pocket of hG6PD throughout the MD simulations is shown in . Hydrogen bonding, hydrophobic interactions and water bridges formed between ligands and target were considered and their interaction occupancies were calculated using derived trajectory frames from MD simulations. Three complexes were submitted in postprocessing analysis and the interaction fractions for each residue at the binding pocket are shown in . In the case of DMSA, the profile suggests that His201 is the most popular amino acid, contributed in the binding interactions. This residue simultaneously establishes hydrogen bonding, ionic bond and water bridges with DMSA atoms. Lys171 is the other key amino acid, participating in forming of hydrogen bonding, water bridges and ionic bond interactions. A water molecule in the active site mediates in establishing of polar interactions by Asp258, as describing the important role of waters in stabilization of ligand. DTPA compound is large in size, and it is expected to have interactions with various amino acids. Lys360, Asp258, Arg246, Glu239 and His201 are the most important amino acids forming the active site of the complex. The interaction profile of MDP-bound complex reveals that Lys47, Glu239, Lys205, His201, Tyr202, Gln305 and Arg365 amino acids can significantly play into the ligand-protein interactions. In light of our interaction analysis, performed throughout MD simulation, we found that inhibitor-binding pocket of hG6PD is increasingly tending to accommodate the ligands via polar and charged connections, and the role of the hydrophobic interactions has not been pronounced. shows 3D ligand interaction diagrams of studied compounds.
Conclusions
The used of 99mTc chelators (MIBI, DTPA, DMSA and MDP) may worsen the health of patients who already have a deficiency of G6PD enzyme. According to these data, these radiopharmaceutical chelators were found to be more efficient inhibitors to G6PD enzyme activity than were other drugs. Owing to the wide use of these compounds in both humans and animals, knowing the effect of these materials on G6PD activity is important. For this reason, if these materials are given to the patients with G6PD deficiency, their dosages should be very tightly monitored to prevent their side-effects on G6PG in red blood cells. Molecular simulations results revealed that polar interactions, not the hydrophobic interactions play important role at the binding of the studied compounds in this study.
Declaration of interest
The authors report no conflicts of interest. The authors alone are responsible for the content and writing of this article.
References
- Cappellini MD, Fiorelli G. Glucose-6-phosphate dehydrogenase deficiency. Lancet 2008;371:64–74
- Sahin A, Senturk M, Ciftci M, et al. Effects of thallium-201 and radioactivity on human erythrocyte glucose 6-phosphate dehydrogenase activity. Nucl Med Biol 2010;37:389–94
- Kuo W, Lin J, Tang TK. Human glucose-6-phosphate dehydrogenase (G6PD) gene transforms nih 3t3 cells and induces tumors in nude mice. Int J Cancer 2000;85:857–64
- Beutler E. G6PD deficiency. Blood 1994;84:3613–36
- Bergmeyer HU, Grassl M, Walter HE. In: Bergmeyer HU, ed. Methods of enzymatic analysis. 3rd ed. Vol. II. Deerfield Beach, FL: Verlag Chemie; 1983: pp. 222–3
- Senturk M, Kufrevioglu OI, Ciftci M. Effects of some antibiotics on human erythrocyte glutathione reductase: an in vitro study. J Enzyme Inhib Med Chem 2008;23:144–8
- Kanji MI, Toews ML, Carpar WR. A kinetic study of glucose 6-phosphate dehydrogenase. J Biol Chem 1976;25:2258–62
- Jacquin C, Nasica X, Guillard D, et al. Intravitreal treatment of endophthalmitis. Méd Maladies Infect 2001;3:1493–7
- Senturk M, Ceyhun SB, Erdogan O, Kufrevioglu OI. In vitro and in vivo effects of some pesticides on glucose-6-phosphate dehydrogenase enzyme activity from rainbow trout (Oncorhynchus mykiss) erythrocytes. Pestic Biochem Phys 2009;95:95–9
- Mousa SA, Williams SJ, Sands H. Characterization of in vivo chemistry of cations in the heart. J Nucl Med 1987;28:1351–7
- Hjelstuen OK. Technetium-99m chelators in nuclear medicine. A review Analyst 1995;120:863–6
- Lever SZ, Wagner Jr HN. Technetium and rhenium in chemistry and nuclear medicine 3, Cortina International, Verona; 1990, pp. 649–59
- Meerdink DJ, Leppo JA. Experimental studies of the physiologic properties of technetium-99m agents: myocardial transport of perfusion imaging agents. Am J Cardiol 1990;66:9–15
- Hanson RN. Radiopharmaceuticals structure-activity relationships. New York: Grune and Stratton; 1981:314–16
- Ciftci M, Kufrevioglu OI, Gundogdu M, Ozmen I. Effects of some antibiotics on enzyme activity of glucose-6-phosphate dehydrogenase from human erythrocytes. Pharmacol Res 2000;41:109–13
- Bradford MM. A rapid and sensitive method for the quantitation of microgram quantities of protein utilizing the principle of protein-dye binding. Anal Biochem 1976;72:248–51
- Laemmli DK. Cleavage of structural proteins during the assembly of the head of bacteriophage T4. Nature 1970;227:680–3
- Beutler E. Red cell metabolism manual of biochemical methods. London: Academic Press; 1971
- Coban TA, Senturk M, Ciftci M, Kufrevioglu OI. Effects of some metal ions on human erythrocyte glutathione reductase: an in vitro study. Protein Pept Lett 2007;14:1027–30
- Kotaka M, Gover S, Vandeputte-Rutten L, et al. Structural studies of glucose-6-phosphate and NADP+ binding to human glucose-6-phosphate dehydrogenase. Acta Crystallogr Sect D: Biol Crystallogr 2005;495–504
- Siu SWI, Pluhackova K, Böckmann RA. Optimization of the OPLS-AA force field for long hydrocarbons. J Chem Theory Comput 2012;8:1459–70
- Schrödinger Release 2015-3: Maestro, version 10.3, Schrödinger, LLC, New York, NY; 2015
- Schrödinger Release 2014-4: LigPrep, version 3.2, Schrödinger, LLC, New York, NY; 2014
- Friesner R. a, Banks JL, Murphy RB, et al. Glide: a new approach for rapid, accurate docking and scoring. 1. method and assessment of docking accuracy. J Med Chem 2004;47:1739–49
- Jacobson MP, Pincus DL, Rapp CS, et al. A hierarchical approach to all-atom protein loop prediction. Proteins Struct Funct Genet 2004;55:351–67
- Sherman W, Day T, Jacobson MP, et al. Novel procedure for modeling ligand/receptor induced fit effects. J Med Chem 2006;49:534–53
- D. E. Shaw Research. Desmond Molecular Dynamics System, version 2.2, Maestro-Desmond Interoperability Tools, version 2.2, Schrödinger. New York, NY, USA; 2009.
- Laurence DR, Bennett PN, Brown MJ. Clinical Pharmacology. 8th ed. Singapore: Churchill Livingstone; 1997
- Morelli A, Benatti U, Gaetani GF, De Flora A. Biochemical mechanisms of glucose-6-phosphate dehydrogenase deficiency. Proc Natl Acad Sci USA 1978;75:1979–83
- Altikat S, Ciftci M, Büyükokuroglu ME. In vitro effects of some anesthetic drugs on enzymatic activity of human red blood cell glucose 6-phosphate dehydrogenase. Pol J Pharmacol 2002;54:67–71
- Senturk M, Kufrevioglu OI, Ciftci M. Effects of some analgesic anaesthetic drugs on human erythrocyte glutathione reductase: an in vitro study. J Enzyme Inhib Med Chem 2009;24:420–4
- Akkemik E, Senturk M, Ozgeris FB, et al. In vitro effects of some drugs on human erythrocyte glutathione reductase. Turk J Med Sci 2011;41:235–41
- Sahin A, Senturk M, Akkemik E, Ciftci M. The effects of chemical and radioactive properties of Tl-201 on human erythrocyte glutathione reductase activity. Nucl Med Biol 2012;39:161–5
- Senturk M, Alici HA, Beydemir S, Kufrevioglu OI. In vitro and in vivo effects of some benzodiazepine drugs on human and rabbit erythrocyte carbonic anhydrase enzymes. J Enzyme Inhib Med Chem 2012;27:680–4
- Fidan I, Salmas RE, Arslan M, et al. Carbonic anhydrase inhibitors: design, synthesis, kinetic, docking and molecular dynamics analysis of novel glycine and phenylalanine sulphonamide derivatives. Bioorg Med Chem 2015;23:7353–8
- Cakmak R, Durdagi S, Ekinci D, et al. Design, synthesis and biological evaluation of novel nitroaromatic compounds as potent glutathione reductase inhibitors. Bioorg Med Chem Lett 2011;21:5398–402
- Durdagi S, Papadopoulos MG, Papahatjis DP, Mavromoustakos T. Combined 3D QSAR and molecular docking studies to reveal novel cannabinoid ligands with optimum binding activity. Bioorg Med Chem Lett 2007;17:6754–63
- Fotakis C, Gega S, Siapi E, et al. Interactions at the bilayer interface and receptor site induced by the novel synthetic pyrrolidinone analog MMK3. Biochim Biophys Acta 2010;1798:422–32
- Durdagi S, Senturk M, Ekinci D, et al. Kinetic and docking study of phenol-based inhibitors of carbonic anhydrase isoform I, II, IX and XII evidence a new binding mode within the enzyme active site. Bioorg Med Chem 2011;19:1381–9
- Isık S, Vullo D, Durdagi S, et al. Interaction of carbonic anhydrase isozymes I, II, and IX with some pyridine and phenol hydrazinecarbothioamide derivatives. Bioorg Med Chem Lett 2015;25:5636–41
- Balaydin HT, Durdagi S, Ekinci D, et al. Inhibition of human carbonic anhydrase isozymes I, II and VI with A series of bisphenol, methoxy and bromophenol compounds. J Enzyme Inhib Med Chem 2012;27:467–75
- Durdagi S, Guo J, Lees-Miller J, et al. Structure-guided topographic mapping and mutagenesis to elucidate binding sites for the Herg1 potassium channel (KCNH2) activator-NS1643. J Pharmacol Exp Ther 2012;342:441–52
- Ekinci D, Cavdar H, Durdagi S, et al. Structure-activity relationships for the interaction of 5,10-dihydroindeno[1,2-b]indole derivatives with human and bovine carbonic anhydrase isoforms I, II, III, IV, and VI. Eur J Med Chem 2012;49:68–73
- Balaydin HT, Senturk M, Goksu S, Menzek A. Synthesis and carbonic anhydrase inhibitory properties of novel bromophenols and their derivatives including natural products: vidalol B. Eur J Med Chem 2012;54:423–8
- Ekinci D, Şentürk M, Şentürk E. Purification and characterization of carbonic anhydrase enzyme from bovine heart tissue and investigation of inhibition effects of some sulphonamide derivative drugs. Acta Physiologica 2015;215:99