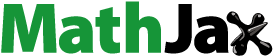
Abstract
The ethyl ether extract of agarwood from an Aquilaria plant afforded six new sesquiterpenoids, Agarozizanol A − F (1−6), together with four known sesquiterpenoids and six known 2-(2-phenylethyl)chromones. Their structures were elucidated via detailed spectroscopic analysis, X-ray diffraction, and comparisons with the published data. All the isolates were evaluated for the α-glucosidase and tyrosinase inhibitory activities in vitro. Compounds 5, 7, 8, and 10 showed significant inhibition of α-glucosidase with IC50 values ranging between 112.3 ± 4.5 and 524.5 ± 2.7 µM (acarbose, 743. 4 ± 3.3 µM). Compounds 13 and 14 exhibited tyrosinase inhibitory effect with IC50 values of 89.0 ± 1.7 and 51.5 ± 0.6 µM, respectively (kojic acid, 46.1 ± 1.3). In the kinetic studies, compounds 5 and 14 were found to be uncompetitive inhibitors for α-glucosidase and mixed type inhibitors for tyrosinase, respectively. Furthermore, molecular docking simulations revealed the binding sites and interactions of the most active compounds with α-glucosidase and tyrosinase.
Introduction
Type 2 diabetes mellitus (T2DM), a chronic metabolic disorder disease, can cause life-threatening long-term complications including heart disease, strokes, blindness, kidney failure, and poor blood flow in the limbsCitation1. In 2015, an estimated 1.6 million deaths directly resulted from diabetes, which is among the top 10 causes of death worldwideCitation2. α-Glucosidase (EC 3.2.1.20) is an exo-acting enzyme, which implicates in the glycoprotein processing and carbohydrate metabolism. Additionally, α-glucosidase catalyzes the cleavage of α-1,4 glycosidic bonds from the nonreducing ends of oligosaccharides and provides high amount of intestine absorbable glucose, which results in the postprandial hyperglycaemia and complications associated with T2DMCitation3,Citation4. Therefore, α-glucosidase inhibition is a very effective therapy to delay glucose absorption and lower blood glucose level after food intake, which can potentially postpone the progression of T2DMCitation5.
As a precious traditional Chinese medicine, agarwood has been used for the treatment of joint pain, inflammatory related dailments, and diarrhoea, as well as a stimulant, sedative, and cardioprotective agentCitation6–8. It is also popular as an incense for cultural and religious ceremonies and its essential oil is further processed into perfume in cosmetics. Previous chemical studies revealed that sesquiterpenoids and 2-(2-phenylethyl)chromone derivatives are the two principal and aroma components in agarwood. To date, over 130 sesquiterpenoids and 120 2-(2-phenylethyl)chromone derivatives have been isolated from agarwoodCitation9 and many of them exhibited various pharmacological properties including antibacterialCitation10, anti-inflammatoryCitation11, α-glucosidase inhibitoryCitation12, acetylcholinesterase inhibitoryCitation13, cytotoxicCitation12, neuroprotectiveCitation14, and antidepressant activitiesCitation15. As part of our long-term project to chemically and biologically characterize chemical constituents from agarwood, the ethyl ether extract of agarwood from an Aquilaria plant was found to potently inhibit α-glucosidase. Then, a phytochemical investigation on the agarwood of an Aquilaria plant was performed and 10 α-glucosidase inhibitory sesquiterpenoids including 6 new sesquiterpenoids, Agarozizanol A − F (1−6), together with 4 known sesquiterpenoids () were isolated and identified. Additionally, 6 known 2-(2-phenylethyl)chromones were also obtained. 2-(2-Phenylethyl)chromones are structurally similar to the flavones except for presence of additional ethyl group and many flavones show intriguing tyrosinase inhibitory activityCitation16–18. Tyrosinase, a rate-limiting enzyme for the melanin biosynthesis, involves in pigmentation of skinCitation19, browning in fresh vegetables and fruitsCitation20, cuticle formation in insectsCitation21,Citation22, and neurodegeneration associated with Parkinson’s diseaseCitation23. Hence, tyrosinase inhibitors have broad application in medicines, food preservatives, bio-insecticides, and cosmetic products. Inspired by this, All the 2-(2-phenylethyl)chromones were assayed for their tyrosinase inhibitory activity. Moreover, the inhibition mechanism were investigated. Herein, we report the isolation, structure elucidation, α-glucosidase and tyrosinase inhibitory activities, as well as kinetic and molecular docking studies of these compounds.
Materials and methods
General experimental procedures
Optical rotations were measured on an Anton Paar MCP 5100 polarimeter. IR spectra were obtained using a Nicolet 380 FT-IR instrument (Thermo, USA) using KBr pellets. HRESIMS were determined by a Waters Autospec Premier (Waters, USA) mass spectrometer. The NMR spectra were recorded on Bruker Avance 500 NMR spectrometers (Bruker, Germany) with TMS as an internal standard. The high performance liquid chromatography (HPLC) was performed with an analytic reversed-phased column (YMC–packed C18, 250 mm × 10 mm, 5 µm) (YMC, Japan) using a G1311C 1260 Quat Pump VL and detected with a G1315D 1260 DAD VL detector (190–500 nm) (Agilent Technologies 1260 infinity, USA). For column chromatography, silica gel (60–80, 200–300 mesh, Qingdao Haiyang Chemical Co., Ltd, China), ODS gel (20–45 µm, Fuji Silysia Chemical Co., Ltd, USA), and Sephadex LH-20 (Merck, Germany) were used. TLC analysis was performed on precoated silica gel GF254 plates (Qingdao Haiyang Chemical Co., Ltd, China), and spots were visualized by spraying with 5% H2SO4 in EtOH followed by heating. Thermo fisher scientific plate reader was used for enzyme inhibition assays.
Plant material
The agarwood was purchased from Bangkok, Thailand in August 2014 and its original plant was identified as a species of the genus Aquilaria by gene sequence analysis of the ITS region. A voucher specimen (201408SLLK) was retained at the Institute of Tropical Bioscience and Biotechnology, Chinese Academy of Tropical Agricultural Sciences.
Extraction and isolation
Air-dried agarwood (384.0 g) was ground and extracted with ethyl ether (1.5 L) for three times. The obtained extract was tested for the α-glucosidase inhibitory activity and showed inhibition rate of 44.13 ± 1.92% at concentration of 50 µg/mL (acarbose, 62.06 ± 4.77%). Then, the ethyl ether extract (20.4 g) was subjected to ODS column chromatography (CC) eluting with MeOH/H2O (v/v, 2:3, 1:1, 3:2, 7:3, 4:1, 9:1, 1:0) to obtain 16 fractions (Fr.1–Fr.16). Fr.4 (0.88 g) was separated by silica gel CC (petroleum ether/EtOAc, 50:1–5:2) to afford 12 subfrctions (Fr.4.1–Fr.4.12), Fr.4.5 was purified by silica gel CC (petroleum ether/EtOAc, 50:4) to yield compound 6 (3.5 mg). Purification of subfraction Fr.4.6 using silica gel CC (petroleum ether/EtOAc, 50:4) furnished compound 10 (3.3 mg). Fr.5 (5.3 g) was applied to silica gel CC eluted by petroleum ether/EtOAc (1:0–5:1), yielding 15 subfrctions (Fr.5.1–Fr.5.15). Separation of Fr.5.2 by silica gel CC (petroleum ether/CHCl3/EtOAc, 50:10:1) afforded compounds 1 (2.9 mg) and 5 (3.2 mg). Compounds 2 (2.9 mg) and 3 (2.8 mg) were obtained by separation of subfraction Fr.5.3 with silica gel CC (petroleum ether/EtOAc/isopropyl alcohol, 100:10:1). Subfraction Fr.5.4 was separated by silica gel CC (petroleum ether/CHCl3/isopropyl alcohol, 100:10:1) and preparative TLC, yielding compounds 9 (2.2 mg) and 4 (1.2 mg). Subfraction Fr.5.7 was chromatographed by silica gel CC (petroleum ether/CHCl3/isopropyl alcohol, 100:15:1) to obtain compounds 7 (1.2 mg) and 8 (2.3 mg). Fr.6 (0.6 g) was fractioned by silica gel CC (petroleum ether/CHCl3/CH3OH, 2:1:1), leading to isolation of 7 subfractions (Fr.6.1–Fr.6.7). Isolation of subfraction Fr.6.6 using silica gel CC and semi-preparative HPLC obtained compounds 15 (18.0 mg) and 16 (5.4 mg). Fr.7 (1.3 g) was divided into 13 subfractions (Fr.7.1–Fr.7.13) by silica gel CC using petroleum ether/EtOAc (500:1–0:1) as eluent. Subfraction Fr.7.11 was isolated via silica gel CC and semi-preparative HPLC to yield compounds 12 (2.2 mg) and 13 (3.0 mg). Fr.8 (0.8 g) was purified by silica gel CC (petroleum ether/EtOAc, 200:1-0:1), affording compounds 11 (0.8 mg) and 14 (3.5 mg).
Agarozizanol A (1)
Colorless crystals; [α]25D -3 (c 0.28, MeOH); IR (KBr) νmax cm−1: 3252, 2956, 2870, 1629, 1462, 1090, and 1044; 1H and 13C NMR data, see and ; HRESIMS m/z 259.1667 [M + Na]+ (calcd for C15H24NaO2, 259.1669); 1 D and 2 D NMR, IR spectra, and HRESIMS, see Figure S2–S9 in Supplementary material.
Table 1. 1H NMR (500 MHz) data for compounds 1−6.
Table 2. 13C NMR (125 MHz) data for compounds 1−6.
Agarozizanol B (2)
Colorless crystals; [α]25D +15 (c 0.28, MeOH); IR (KBr) νmax cm−1: 3413, 2956, 2870, 1450, 1381, 1261, and 1059; 1H and 13C NMR data, see and ; HRESIMS m/z 261.1820 [M + Na]+ (calcd for C15H26NaO2, 261.1825). 1 D and 2 D NMR, IR spectra, and HRESIMS, see Figures S10–S17 in Supplementary material.
Agarozizanol C (3)
White amorphous powder; [α]25D -11 (c 0.25, MeOH); IR (KBr) νmax cm−1: 3444, 2956, 2868, 1456, 1376, 1250, 1091, and 1027; 1H and 13C NMR data, see and ; HRESIMS m/z 261.1817 [M + Na]+ (calcd for C15H26NaO2, 261.1825). 1 D and 2 D NMR, IR spectra, and HRESIMS, see Figure S18-Figure S25 in Supplementary material.
Agarozizanol D (4)
White amorphous powder; [α]25D +14 (c 0.10, MeOH); IR (KBr) νmax cm−1: 3439, 2950, 2867, 1457, 1374, and 1067; 1H and 13C NMR data, see and ; HRESIMS m/z 261.1826 [M + Na]+ (calcd for C15H26NaO2, 261.1825). 1 D and 2 D NMR, IR spectra, and HRESIMS, see Figure S26–S33 in Supplementary material.
Agarozizanol E (5)
White amorphous powder; [α]25D -7 (c 0.33, MeOH); IR (KBr) νmax cm−1: 3447, 2938, 2863, 1627, 1456, 1377, 1260, 1094, and 1034; 1H and 13C NMR data, see and ; HRESIMS m/z 243.1707 [M + Na]+ (calcd for C15H24NaO, 243.1719). 1 D and 2 D NMR, IR spectra, and HRESIMS, see Figure S34–S41 in Supplementary material.
Agarozizanol F (6)
White amorphous powder; [α]25D +2 (c 0.36, MeOH); IR (KBr) νmax cm−1: 3446, 2928, 2867, 1617, 1454, 1382, and 1084; 1H and 13C NMR data, see and ; HRESIMS m/z 259.1662 [M + Na]+ (calcd for C15H24NaO2, 259.1669). 1 D and 2 D NMR, IR spectra, and HRESIMS, see Figure S42–S49 in Supplementary material.
X-ray crystallographic analysis of compound 2
A suitable crystal of 2 obtained from MeOH was selected and determined on a Bruker D8 QUEST diffractometer at 296.15 K. Crystal data for compound 2: C15H26O2 (M = 238.36 g/mol), orthorhombic, space group P212121, a = 6.7545(7) Å, b = 8.8069(8) Å, c = 23.886(2) Å, V = 1420.9 (2) Å3, Z = 4, T = 296(2) K, µ (Cu Kα)=0.556 mm−1, Dcalc = 1.114 g/cm3, 9320 reflections collected (7.402° ≤ 2θ ≤ 136.888°), 2598 unique (Rint = 0.1110, Rsigma = 0.0937). The final R1 was 0.0559 (I > 2σ(I)) and wR2 was 0.1624 (all data), Flack parameter = 0.3(8). The crystallographic data for 2 have been deposited at the Cambridge Crystallographic Data Centre under the reference number CCDC 1873716. This data can be obtained free of charge via http://www.ccdc.cam.ac.uk/conts/retrieving.html or from CCDC, 12 Union Road, Cambridge, CB21EZ, UK; (fax: +44-(0)1223-336033 or e-mail: [email protected]).
α-Glucosidase inhibitory assay
The α-glucosidase (G5003, Sigma-Aldrich) was obtained from Saccharomyces cerevisiae and the enzyme inhibitory assay was performed using formerly described methodCitation24 with some modifications. The samples were dissolved in DMSO and 2-fold diluted to afford a serial concentrations (the highest final concentration was set at 750 µM because of low solubility of 1–10 in buffer). 10 µL sample was incubated with 100 µL α-glucosidase solution (0.2 U/mL in 100 mM phosphate buffer (pH: 6.8)) at 37 °C for 15 min. Then, 40 µL of 2.5 mM p-nitrophenyl-α-d-glucopyranoside (p-NPG) were added and further incubated at 37 °C for 15 min. DMSO instead of compound was used as control and the blank wells contained buffer in place of substrate. The OD values were measured at 405 nm with microplate reader. Acarbose was used as reference compound. The percentage inhibition was calculated using the following equation:
Tyrosinase inhibitory assay
All the isolated compounds (1–16) were tested for the inhibitory activity against tyrosinase (T3824, Sigma-Aldrich) from mushroom according to the previously reported methodCitation16 with slight modifications. Briefly, 130 µL of tyrosinase (50 U/mL) solvated in 50 mM phosphate buffer (pH: 6.8) were mixed with 20 µL of 2-fold serial dilutions (from 1 mM to 0.03125 mM) of compounds in DMSO, and transferred into a 96-well plate. After 5 min pre-incubation of the mixture at 37 °C, 50 µL of 2 mM l-tyrosine in buffer were added and incubated for additional 20 min at 37 °C. The control wells contained DMSO instead of compound, and the blank wells were added with buffer in place of l-tyrosine. Kojic acid was used as a positive control. The absorbance was measured at 495 nm using microplate reader. The percentage inhibition was calculated using the formula as below:
Kinetic analysis
To determine the mechanisms by which the most effective compound 5 inhibited α-glucosidase and compound 14 inhibited tyrosinase, the enzyme kinetic analysis was investigated at various concentrations of substrate (2.5, 1.25, 0.625, and 0.3125 mM p-NPG for α-glucosidase; 4.0, 2.0, 1.0, and 0.5 mM tyrosine for tyrosinase) in the absence or presence of different test inhibitor concentrations (0, 187.5, and 750 µM of 5; 0, 25, and 100 µM of 14) according to the inhibition assay described above. The data were expressed as double-reciprocal Lineweaver − Burk plot and the inhibition constants (KI and KIS) were obtained from secondary plots of slope and y intercept of lines from Lineweaver-Burk plot against inhibitorCitation25.
Molecular docking study
The X-ray structures of mushroom tyrosinase (PDB: 2Y9X) at a resolution of 2.78 Å and α-glucosidase (PDB: 3A4A) co-crystalized with glucose at a resolution of 1.6 Å were achieved from RCSB Protein Data Bank (www.rcsb.org). All hydrogen atoms and gasteiger charges in the protein structure were added by AutoDockTools and molecular docking simulations were performed with Autodock 4.2 software using the Lamarckian Genetic AlgorithmCitation26,Citation27. Compounds 5 and 10 were docked into the catalytic site of α-glucosidase with a grid box of 50 Å × 50 Å × 50 Å at 0.375 Å space, centered at coordinates of x = 21.275, y = −0.741, and z = 18.635. Genetic Algorithm parameters were set at Runs 50 and the maximum number of evals was set as medium (25000000). For the compounds 13 and 14, mixed type tyrosinase inhibitors, a blind molecular docking simulation was performed (Runs 20 and the maximum number of evals was set as short (250000)). The size of grid box was set at 100 Å × 100 Å × 100 Å in the x, y, and z dimensions at 0.375 Å space. This computational docking identified the site that bound tightly to the ligand. Then, a refined docking simulation was conducted with smaller grid box of 30 Å × 44 Å × 30 Å at 0.375 Å space, centered at the above identified binding site (x = −16.345, y = −36.541, z = −22.916). The Genetic Algorithm parameters were set at Runs 50 and the maximum number of evals was set as medium (25000000). The predicted geometries were ranked by the binding energies and clustered at RMSD value of 2 Å, and the best pose was selected for further analysis.
Results and discussion
Structure elucidation
Compound 1 was obtained as colorless crystals and its molecular formula was assigned to be C15H24O2 based on the HRESIMS ion peak [M + Na]+ at m/z 259.1667 (calcd for C15H24NaO2, 259.1669), requiring 4 degrees of unsaturation. The 1H NMR data () of 1 showed resonances for two methyl groups at δH 1.10 (3H, s) and 1.13 (3H, s), two exocyclic olefinic protons at δH 4.81 (1H, d, J = 1.5 Hz) and 4.79 (1H, d, J = 1.5 Hz), and one oxygenated methylene at δH 3.53 (1H, t, J = 10.7 Hz) and 3.42 (1H, dd, J = 10.7, 4.5 Hz). The 13C NMR data () of 1 showed fifteen carbon signals which were categorized by HSQC spectrum as two methyl groups, six methylenes (one oxygenated), four methines, and three quaternary carbons. Apart from the double bond (δC 162.6, 108.2), the remaining three degrees of unsaturation combined with above mentioned spectroscopic data implied that 1 was a tricyclic sesquiterpenoid. In the 1H-1H COSY spectrum (), two spin systems were observed: H2-12–H-2–H2-3–H2-4 and H-11–H-8–H2-9–H2-10. The two spin systems in association with key HMBC correlations from H2-12 to C-1, from H2-4 to C-1, C-5, and C-6, from H-5 to C-6, C-7, C-1, C-10, and C-11, from H2-13 to C-6, C-7, and C-8, and from H3-14 and H3-15 to C-5, C-6, and C-7 constructed the planar structure of 1, possessing a prezizaane skeleton, as shown in .
Figure 2. Key 1H-1H COSY (bold lines), HMBC (arrows), and ROESY (dashed double arrows) correlations of compounds 1−6.
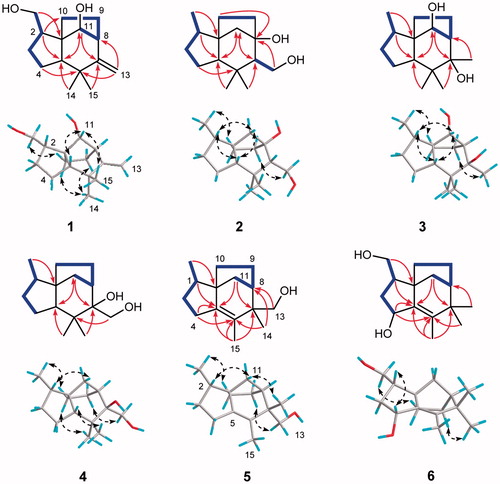
The relative configuration of 1 was clarified unambiguously from analysis of the ROESY spectrum. The ROE cross peak of H2-12 with H-10a revealed that H2-12 and H-10a located at the same side and assumed that they were α oriented. H-11 showed ROESY correlations with H-5 and H3-14, indicating they were cofacial and assigned to be β orientation. Thus, the structure of 1 was determined to be as shown in , named Agarozizanol A.
Compound 2 was isolated as colorless crystals and had a molecular formula C15H26O2 on the basis of its HRESIMS and 13C NMR spectroscopic data. The 1H NMR data () of 2 revealed the presence of three methyl group at δH 0.87 (3H, d, J = 6.7 Hz), 1.06 (3H, s), and 0.79 (3H, s) and one oxygenated methylene at δH 3.89 (1H, dd, J = 11.1, 9.4 Hz) and 3.77 (1H, d, J = 11.1, 2.7 Hz). The 13C NMR () and HSQC spectra of 1 showed the resonances for three methyl groups, six methylenes (one oxygenated), three methines, and three quaternary carbons (one oxygenated). The aforementioned spectroscopic data were similar to those of 8, a co-occurring known prezizaane sesquiterpenoids Jinkohol IICitation28. The only difference was the presence of additional oxidized quaternary carbon signal (δC 83.6) and the absence of a methine signal in the NMR spectra of 2. The HMBC correlations () of H2-13, H2-11, and H2-10 with this carbon suggested that the oxygenated carbon was C-8. H3-12 showed ROESY correlations with H-10a, suggesting they were cofacial. Other ROESY interactions of H-2 with H-5, H-11b and H3-14 with H-5 and H-7 were found, revealing that these protons were resided on the same face. Therefore, the structure of 2 was deduced as Agarozizanol B shown in .
Compound 3, a white amorphous powder, was found to have a molecular formula of C15H26O2 from the ion peak [M + Na]+ at m/z 261.1817 (calcd for C15H26NaO2, 261.1825) in the HRESIMS. The 1H and 13C NMR data ( and ) of 3 exhibited the characteristic signals for prezizane sesquiterpenoid including four methyls, four methylenes, four methines, and three quaternary carbons. The NMR spectroscopic data of 3 closely resemble that of 7, Jinkohol ICitation29, with exception of deshieled C-11 at δC 83.5. This chemical shift indicated the attachment of a hydroxyl to C-11, which was confirmed by HMBC correlation from H-11 to C-9/C-10/C-5/C-7 and 1H-1H COSY correlations of H-11–H-8–H-9–H2-10 (). The ROESY correlations of H-2/H-11/H-5 suggested that these protons were cofacial and β oriented. Additional ROESY cross peaks of H3-12/H-10a and H3-13/H-9b permitted assignment of α orientation to H3-12 and H3-13. Summarizing these data, the structure of 3 was determined as shown in and named Agarozizanol C.
Obtained as white amorphous powder, compound 4 showed an ion peak [M + Na]+ at m/z 261.1826 (calcd for C15H26NaO2, 261.1825) in the HRESIMS, consistent with a molecular formula of C15H26O2. The structure of 4 was similar to that of known compound 7, except for the presence of additional hydroxyl at C-13 in 4, which was verified by the deshielded chemical shift of C-13 at δC 62.7 and the molecular formula of 4 with one more oxygen atom than that of 7. The ROESY interactions of H3-12 with H2-10, H2-9 with H2-13 and H3-15 (), indicated these protons located at the same side of the molecule and were assigned to be α oriented. While H-5, H3-14, H-2, and H-11a were oriented on the other side by their ROESY correlations. Hence, the structure of 4 was established as a new epimer of synthetic (+)-prezizaene diolCitation30, named Agarozizanol D.
Compound 5 was obtained as white amorphous powder and was shown to have a molecular formula C15H24O based on its ion peak [M + Na]+ at m/z 243.1707 (calcd for C15H24NaO, 243.1719) in HRESIMS. Resonances of three methyls at δH 0.95 (3H, d, J = 6.5 Hz), 1.07 (3H, s), and 1.41 (3H, s), and one oxygenated methylene at δH 3.60 (1H, d, J = 10.9 Hz) and 3.54 (1H, d, J = 10.9 Hz) were observed in the 1H NMR spectrum of compound 5. The 13C NMR () and HSQC spectra of 5 showed the presence of three methyl groups, six methylenes (one oxygenated), two methines, and four quaternary carbons including two olefinic carbons. These spectroscopic data of 5 were similar to those of compound 10Citation31, a known zizaene sesquiterpenoid. The major difference was that the hydroxyl, which located at C-12 in the 10, was attached to the C-13 in the compound 5. This assignment was confirmed by the deshielded chemical shifts of C-13 at δC 66.7 and the HMBC correlations between H2-13 and C-6/C-14/C-7/C-8 (). The ROESY correlations of H3-12/H-10a, H2-13/H-9a, and H3-14/H-11b were observed, allowing the assignment of α orientation for H3-12 and H-9a, and β orientation for H3-14 and H-11b. Based on all the above evidence, the structure of 5 was identified as shown in and named as Agarozizanol E.
Compound 6 was obtained as white amorphous powder and displayed an ion peak [M + Na]+ at m/z 259.1662 (calcd for C15H24NaO2, 259.1669) in its HRESIMS, corresponding to a molecular formula C15H24O2 with one more oxygen atom than that of 10. The 1H and 13C NMR data of 6 were closely similar to those of 10Citation31 with only difference being the deshieled chemical shift of CH-4 (δH 4.29, δC 81.3), which indicated the presence of hydroxyl at C-4. This deduction was further verified by HMBC correlations of H-4 with C-1/C-5/C-6 (). The α orientation of CH2OH-12, OH-4, and CH2-10 was assigned from the ROESY correlations of H2-12/H-10b and H-2/H-4. Accordingly, the structure of 6 was characterized as shown in , named Agarozizanol F.
On the basis of their structural similarity, compounds 1−6 should be generated from a common precursor in their biosynthetic pathway (Supplementary material, Figure S1), and possessed the same stereochemistry at C-1 and C-2. Fortunately, single-crystals of 2 were obtained and subjected to X-ray diffraction experiment with Cu Kα radiation (). However, the imperfect Flack parameter [0.3(8)] only allowed confirmation of the above deduced planar structure and relative configuration of 2. Therefore, further effort was needed to determine the absolute configuration of 1−6.
By comparing their experimental spectroscopic data with reported data in the literature, the known compounds were identified as Jinkohol I (7)Citation29, Jinkohol II (8)Citation28, Jinkoholic acid (9)Citation32, isokhusenol (10)Citation31, 6-hydroxy-2-[2-(3-methoxy-4-hydroxyphenyl)ethyl]chromone (11)Citation33, 6-methoxy-7-hydroxy-2-[2-(3-hydroxy-4-methoxyphenyl)ethyl]chromone (12)Citation34, 6-hydroxy-7-methoxy-2-[2-(3-hydroxy-4-methoxyphenyl)ethyl]chromone (13)Citation14, 6,8-dihydroxy-2-[2-(4-methoxyphenyl)ethyl]chromone (14)Citation12, 8-chloro-2–(2-phenylethyl)-5,6,7-trihydroxy-5,6,7,8-tetrahydrochromone (15)Citation35, 8-chloro-2–(2-(4-methoxyphenyl)ethyl)-5,6,7-trihydroxy-5,6,7,8-tetrahydrochromone (16)Citation36.
Bioactivity assays, kinetic analysis, and molecular docking simulations
α-Glucosidase inhibitory activity assay, kinetic analysis, and molecular docking study
All the isolated compounds were assessed for the inhibition against α-glucosidase. The results were listed in the . Compounds 5, 7, 8, and 10 showed inhibitory effect on α-glucosidase (IC50 values ranging from 112.3 ± 4.5 to 524.5 ± 2.7 µM) superior to acarbose (IC50, 743. 4 ± 3.3 µM). Compounds 2, 4, and 9 also possessed inhibitory activity comparable to the positive control.
Table 3. α-Glucosidase inhibitory activities of compounds 1−16Table Footnotea.
To elucidate the type of inhibition of isolated sesquiterpenoids with α-glucosidase, compound 5 with most potency was selected for the kinetic analysis. The Lineweaver-Burk plot () of 5 was established and revealed that Km and Vmax decreased in a parallel fashion, indicating that 5 acted as an uncompetitive α-glucosidase inhibitor by binding only with the enzyme-substrate complexCitation37. The inhibition constant was calculated using secondary plot of y intercept versus concentration of inhibitor, yielding KI value of 168.0 µM ().
Figure 4. Kinetics of α-glucosidase inhibition by 5. Lineweaver-Burk plot of 5 against α-glucosidase (A). Secondary plot of y intercept vs. inhibitor for determination of inhibition constant (B).
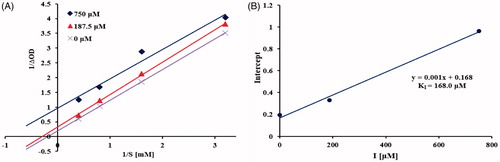
In order to rationalize the uncompetitive inhibition mechanism indicated by kinetic study, molecular modeling studies were performed. The most active compounds 5 and 10 were well docked into the catalytic site of the α-glucosidase () with binding energies of −7.2 and −7.1 kcal/mol, respectively. The docking results revealed that compounds 5 and 10 bound to the entrance part of the active site cavity of α-glucosidase, therefore blocking the release of product (glucose) after the enzyme catalyzed reaction was completedCitation37. This may explain why compound 5 behaved as an uncompetitive inhibitor of α-glucosidase. Specifically, compound 5 bound to the active site through hydrogen bonds with residues Asp 352 and Arg 442, and hydrophobic interactions with Tyr 158, Phe 303, and Arg 315 (). Compound 10 took hydrogen bonding with Asn 415 and hydrophobic interactions with Lys 156, Phe 314, and Arg 315 (). These interactions may provide hints for designing of a new class of α-glucosidase inhibitor.
Figure 5. Docking analysis of 5 (yellow sticks), 10 (green sticks), and acarbose (red sticks) with α-glucosidase co-crystalized with glucose (cyan sticks). Binding mode of 5, 10, acarbose, and glucose in the active site of α-glucosidase (A). 3D cartoon diagram of the interactions of 5 (B), 10 (C), and acarbose (D) with α-glucosidase.
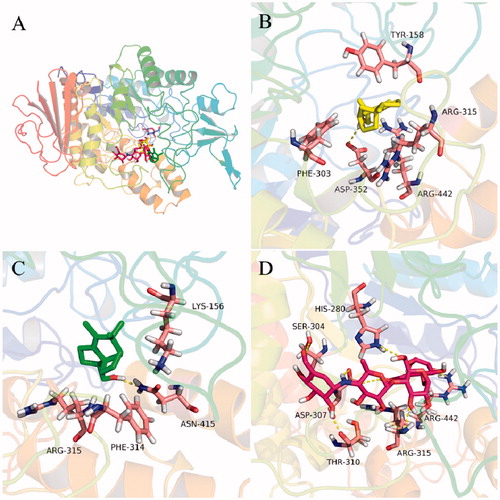
Tyrosinase inhibitory actvity assay, kinetic analysis, and molecular docking study
2-(2-Phenylethyl)chromones 11−16 as well as sesquiterpenoids 1−10 were assayed for the inhibition toward the tyrosinase. As illustrated in , Compounds 14 and 13 displayed potent inhibitory activity against tyrosinase with IC50 values of 51.5 ± 0.6 and 89.0 ± 1.7 µM, respectively (kojic acid, 46.1 ± 1.3 µM). Compound 15 also exhibited weak inhibition effect with inhibition rate of 34.8 ± 1.4% at 100 µM. Whereas, compounds 1−12 and 16 were inactive to the tyrosinase up to concentration of 100 µM (inhibition rate <30%).
Table 4. Tyrosinase inhibitory activities of compounds 1−16Table Footnotea.
As shown in the Lineweaver-Burk plot of the most active compound 14 (), increasing inhibitor concentration resulted in a decrease in Vmax and an increase in Km, which indicated a mixed type inhibition for 14 against tyrosinaseCitation25. Thus, compound 14 inhibited the tyrosinase not only by binding with the free enzyme but also with the enzyme − substrate complex. The inhibition constants of 14 binding with the free enzyme (KI) and with enzyme-substrate complex (KIS) were determined by the slope and the y intercept versus the inhibitor concentration, respectively. From , the inhibition constants KI and KIS of 14 were calculated to be 39.6 µM and 72.8 µM, respectively, by secondary re-plots, indicating that compound 14 effectively bound to free enzyme as compared to enzyme-substrate complex.
Figure 6. Kinetics of tyrosinase inhibition by 14. Lineweaver-Burk plot of 14 against tyrosinase (A). Secondary re-plot of slope vs. inhibitor for determination of inhibition constant KI (B). Secondary re-plot of y intercept vs. inhibitor for determination of inhibition constant KIS (C).
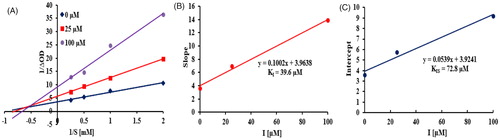
To further gain insight into the binding mechanism of 2-(2-phenylethyl)chromones with tyrosinase, molecular docking simulations were performed. As presented in , compounds 13 and 14 were able to anchor the same binding pocket of tyrosinase with the binding energy of −7.49 and −7.75 kcal/mol, respectively. Compound 14 formed hydrogen bonds with residues Gln 41, Lys 180, and hydrophobic interactions with residues His 178 and Gln 44. However, compound 13 bound to the binding site with a different mode and interacted via hydrogen bonds with residues Gln 41, Lys 180, Asn 174, and Glu173, and hydrophobic interactions with residues Gln 44 and Ala 45. The differences may stem from the substitution of ring A and B of 2-(2-phenylethyl)chromones. These results unveiled a possible allosteric site of tyrosinase by 2-(2-phenylethyl)chromones and will be helpful for understanding the binding interactions between 2-(2-phenylethyl)chromones and tyrosinase.
Conclusions
The ethyl ether extract of agarwood from an Aquilaria plant afforded 6 previously undescribed sesquiterpenoids, 4 known sesquiterpenoids, as well as 6 known 2-(2-phenylethyl)chromones. Among them, compounds 2, 4, 5, and 7–10 showed intriguing inhibitory activity against α-glucosidase. The combined enzyme kinetic and molecular docking studies were performed and revealed that the most effective compound 5, a new zizaane-type sesquiterpenoid, behaved as an uncompetitive α-glucosidase inhibitor and blocked catalytic reaction by interacting with the entrance of active site of α-glucosidase. Uncompetitive inhibitors are considered to be superior to competitive and noncompetitive inhibitors in drug development and are expected to display better in vivo efficacyCitation37,Citation38. Therefore, compound 5 could serve as a new promising lead compound for synthesis of more potent derivatives as α-glucosidase inhibitors. Additionally, compounds 13 and 14 manifested remarkable tyrosinase inhibitory effect. Compound 14 with the most potency was revealed to have affinity for the allosteric site of tyrosinase as a mixed type inhibitor. Agarwood formation is due to a self-defense mechanism initiated by the tree to fight off pests and pathogens invading through holes and wounds on the tree surfaceCitation6. 2-(2-Phenylethyl)chromone is one of the two main components of agarwood and our study revealed the 2-(2-phenylethyl)chromones exert inhibitory activity on tyrosinase, which may provide the chemical evidence for the self-defense mechanism of the agar-producing tree against pests.
Supplemental Material
Download PDF (3.2 MB)Disclosure statement
The authors declare no conflict of interest.
Additional information
Funding
References
- Nathan DM. Long-term complications of diabetes mellitus. N Engl J Med 1993;328:1676–85.
- Santos-Longhurst A, Krucik G. Type 2 diabetes statistics and facts. Healthline 2014. Available from: www.healthline.com/health/type-2-diabetes/statistics.
- Song YH, Uddin Z, Jin YM, et al. Inhibition of protein tyrosine phosphatase (PTP1B) and α-glucosidase by geranylated flavonoids from Paulownia tomentosa. J Enzyme Inhib Med Chem 2017;32:1195–202.
- Bojarova P, Kren V. Glycosidases: a key to tailored carbohydrates. Trends Biotechnol 2009;27:199–209.
- Israili ZH. Advances in the treatment of type 2 diabetes mellitus. Am J Ther 2011;18:117–52.
- Lee SY, Mohamed R, The origin and domestication of Aquilaria, an important agarwood-producing genus, Agarwood. Singapore: Springer, 2016;pp.1–20.
- Yang D-L, Wang H, Guo Z-K, et al. A new 2-(2-phenylethyl) chromone derivative in Chinese agarwood ‘Qi-Nan’ from Aquilaria sinensis. J Asian Nat Prod Res 2014;16:770–6.
- Hashim YZH-Y, Kerr PG, Abbas P, et al. Aquilaria spp. (agarwood) as source of health beneficial compounds: a review of traditional use, phytochemistry and pharmacology. J Ethnopharmacol 2016;189:331–60.
- Dai H-F, Research progress of agarwood. Beijing: Science press, 2017.
- Li W, Cai C-H, Guo Z-K, et al. Five new eudesmane-type sesquiterpenoids from Chinese agarwood induced by artificial holing. Fitoterapia 2015;100:44–9.
- Huo H-X, Zhu Z-X, Song Y-L, et al. Anti-inflammatory dimeric 2-(2-phenylethyl) chromones from the resinous wood of Aquilaria sinensis. J Nat Prod 2018;81:543–53.
- Liao G, Mei W-L, Dong W-H, et al. 2-(2-Phenylethyl) chromone derivatives in artificial agarwood from Aquilaria sinensis. Fitoterapia 2016;110:38–43.
- Yang D-L, Mei W-L, Zeng Y-B, et al. 2-(2-phenylethyl)chromone derivatives in Chinese agarwood “Qi-Nan” from Aquilaria sinensis. Planta Med 2013;79:141329–34.
- Yang L, Qiao L, Xie D, et al. 2-(2-phenylethyl)chromones from Chinese eaglewood. Phytochemistry 2012;76:92–7.
- Yang L, Qiao L, Ji C, et al. Antidepressant abietane diterpenoids from Chinese eaglewood. J Nat Prod 2013;76:216–22.
- Kim JH, Cho IS, So YK, et al. Kushenol A and 8-prenylkaempferol, tyrosinase inhibitors, derived from Sophora flavescens. J Enzyme Inhib Med Chem 2018;33:1048–54.
- Chang TS. An updated review of tyrosinase inhibitors. Int J Mol Sci 2009; 10:2440–75.
- Şöhretoğlu D, Sari S, Barut B, et al. Tyrosinase inhibition by some flavonoids: inhibitory activity, mechanism by in vitro and in silico studies. Bioorg Chem 2018;81:168–74.
- Olivares C, Solano F. New insights into the active site structure and catalytic mechanism of tyrosinase and its related proteins. Pigment Cell Melanoma Res 2009;22:750–60.
- Mcevily JA, Iyengar R, Otwell WS. Inhibition of enzymatic browning in foods and beverages. Crit Rev Food Sci Nutr 1992;32:253–73.
- Guerrero A, Rosell G. Biorational approaches for insect control by enzymatic inhibition. Curr Med Chem 2005;12:461–9.
- Gorman MJ, An C, Kanost MR. Characterization of tyrosine hydroxylase from Manduca sexta. Insect Biochem Mol Biol 2007;37:1327–37.
- Xu Y, Stokes AH, Freeman WM, et al. Tyrosinase mRNA is expressed in human substantia nigra. Mol Brain Res 1997;1:4559–162.
- Liu Q-B, Cheng Z-Y, Yan Z-Y, et al. Prunus tomentosa seed waste as a source of aromatic glycosides: valuable phytochemicals with α-glucosidase inhibitory and hepatoprotective properties. Ind Crops Prod 2018;111:590–6.
- Si Y-X, Ji S, Fang N-Y, et al. Effects of piperonylic acid on tyrosinase: mixed-type inhibition kinetics and computational simulations. Process Biochem 2013;48:111706–14.
- Morris GM, Goodsell DS, Halliday RS, et al. Automated docking using a Lamarckian genetic algorithm and an empirical binding free energy function. J Comput Chem 1998;19:1639–62.
- Huey R, Morris GM, Olson AJ, et al. A semiempirical free energy force field with charge-based desolvation. J Comput Chem 2007;28:1145–52.
- Nakanishi T, Yamagata E, Yoneda K, et al. Jinkoh-eremol and jinkohol II, two new sesquiterpene alcohols from agarwood. J Chem Soc Perkin Trans 1983;1:601–4.
- Nakanishi T, Yamagata E, Yoneda K, et al. Jinkohol, a prezizane sesquiterpene alcohol from agarwood. Phytochemistry 1981;20:1597–9.
- Sharma MK, Banwell MG, Willis AC. Generation of (+)‐prezizanol, (+)‐prezizaene, and the ent‐β‐isopipitzol framework via cationic rearrangement of khusiol and related compounds. Asian J Org Chem 2014;3:5632–7.
- Komae H, Nigam IC. Essential oils and their constituents. XXXIX. Structures of khusenic acid and isokhusenic acid, two sesquiterpenic constituents of oil of vetiver. J Org Chem 1968;33:1771–3.
- Raharivelomanana P, Bianchini JP, Faure RA, et al. Jinkoholic acid, a prezizane sesquiterpene from Neocallitropsis pancheri. Phytochemistry 1994;35:1059–60.
- Shao H, Mei W-L, Li W, et al. Chemical constituents of agarwood originating from Gyrinops salicifolia. Nat Prod Res Dev 2015;27:2046–9.
- Shao H, Mei W-L, Dong W-H, et al. 2-(2-Phenylethyl) chromone derivatives of agarwood originating from Gyrinops salicifolia. Molecules 2016;21:1313.
- Yagura T, Ito M, Kiuchi F, et al. Four new 2-(2-phenylethyl)chromone derivatives from withered wood of Aquilaria sinensis . Chem Pharm Bull 2003;51:560–4.
- Huo H-X, Gu Y-F, Sun H, et al. Anti-inflammatory 2-(2-phenylethyl) chromone derivatives from Chinese agarwood. Fitoterapia 2017;118:49–55.
- Song YH, Kim DW, Curtis-Long MJ, et al. Cinnamic acid amides from Tribulus terrestris displaying uncompetitive α-glucosidase inhibition. Eur J Med Chem 2016;114:201–8.
- Cornish-Bowden A. Why is uncompetitive inhibition so rare? A possible explanation, with implications for the design of drugs and pesticides. FEBS Lett 1986;203:3–6.