Abstract
Ricin toxin A chain (RTA), from Ricinus communis, is a deadly protein that inactivates ribosomes by degrading an adenine residue at position 4324 in 28S rRNA. Recently, we have demonstrated that pterin-7-carboxamides with peptide pendants were potent RTA inhibitors. Among these, N-(pterin-7-carbonyl)glycyl-L-tyrosine (7PCGY) is the most potent RTA inhibitor as a small organic molecule. However, despite this fascinating inhibitory activity, the mode of interaction of 7PCGY with RTA remains elusive. This study aimed to elucidate the factors responsible for the high RTA inhibitory activity of 7PCGY based on X-ray crystallographic analysis. Herein, we report the successfully resolved X-ray crystal structure of 7PCGY/RTA complexes, revealing that the interaction between the phenolic hydroxy group in 7PCGY and Asn78 of RTA through a hydrogen bonding and the conformational change of Tyr80 and Asn122 are responsible for the high RTA inhibitory activity of 7PCGY.
Introduction
Ricin is a member of ribosome-inactivating proteins (RIPs) and is an N-glycosidase that catalyses the hydrolytic cleavage of an adenine residue at position 4324 (A4324) in 28S ribosomal RNA. Through this degradation, the protein-synthesizing system in the mammalian ribosome is disrupted, leading to cell deathCitation1,Citation2. Ricin is composed of two polypeptide chains, an A-chain and a B-chain, connected via a disulphide bond. The ricin toxin A chain (RTA) is involved in the toxic action of ricin, whereas the ricin toxin B chain (RTB) is a lectin that recognises carbohydrate residues on the cell surface to allow whole ricin to enter a cell. After entry, RTA is detached and subsequently exerts its toxic N-glycosidase activityCitation3. From a public health perspective, the development of antidotes against RTA is a recognised necessity. The active site of RTA consists of two pockets, the primary and secondary pockets with Tyr80 dividing these pockets. In the primary pocket, the A4324 is captured by hydrogen bonds with Val81 and Gly121 and by π-π interactions with Tyr123 and Tyr80, and is cleaved by the catalytic action of Arg180 and Glu177. Whereas, the secondary pocket accommodates a guanine residue adjacent to A4324Citation3. Based on this structural information, extensive efforts have been dedicated to developing RTA inhibitors, but most experiments have yielded unsatisfactory resultsCitation4–9. Based on this background, we recently developed several pterin-7-carboxamides (7PCAs) with peptide pendants that exhibited weak to good inhibition of RTA in vitroCitation10. Among these, N-(pterin-7-carbonyl)glycyl-L-tyrosine (7PCGY, ) was the most potent, with an IC50 value of 6 µM, followed by N-(pterin-7-carbonyl)glycyl-L-phenylalanyl-L-phenylalanine (7PCGFF, ) and N-(pterin-7-carbonyl)glycyl-L-phenylalanine (7PCGF, ), with IC50 values of 15 and 20 µM, respectively. X-ray crystallographic analyses of 7PCGFF/RTA and 7PCGF/RTA cocrystals were performed, and the structural information related to their inhibitory activities was elucidated. However, the crystal structure of RTA incorporating the most potent 7PCGY has not yet been determined; therefore, the details of the interaction between RTA and 7PCGY responsible for such a high inhibitory activity (IC50 = 6 µM) remain elusive. The study aimed to determine the crystal structure of 7PCGY/RTA and the mode of interaction of 7PCGY with RTA.
Materials and methods
Synthesis
The molecule 7PCGY was synthesised by mixing 7-methoxycarbonylpterin (7MCP)Citation11 with glycyl-L-tyrosine in the presence of 1,8-diazabicyclo[5.4.0]undec-7-ene (DBU) in methanol at room temperature, as described previouslyCitation10,Citation12. Spectral data for this material were in good agreement with those reported previouslyCitation10.
Protein production
The pET28a plasmid containing the His-RTA expression sequence was purchased from GenScript (Tokyo, Japan) and transformed into E. coli BL21(DE3) cells following the calcium treatment method. Transformed strains were incubated for 16h at 32 °C on Luria–Bertani (LB) agar culture supplemented with kanamycin. The generated colonies were picked up using a pipet tip and inoculated into 5 L of LB medium, and then incubated at 32 °C with shaking. After approximately 6 h, the bacterial growth was visually confirmed via turbidity. Thereafter, isopropyl-β-D-thiogalactopyranoside (IPTG) was added to the medium to induce expression, and the cultivation continued for 16h at 32 °C. The bacteria were collected by centrifugation and were ultrasonically crushed to obtain a crude extract. His-tagged proteins were collected using a TALON column, dialysed against 10 mM sodium acetate buffer (pH 4.0), and concentrated using an ultrafiltration filter to obtain the target proteins. Crystallisation of RTA was subsequently performed by the hanging drop vapour diffusion method using the obtained RTA protein solution, as described below.
Crystallisation, x-ray data collection and processing, structure determination and refinement
RTA crystals were grown at 23 °C following the hanging drop method using 7.8 mg/mL protein, 11% PEG2000, 0.2 M lithium sulphate, and 0.1 M sodium acetate at pH 4.5. Crystals of the RTA complex with synthetic ligands were obtained by soaking RTA crystals in a crystallisation reservoir containing 3.0 mM of the compound.
Diffraction data of the RTA-ligand complex crystals were collected at 95 K using a PILATUS3 S 6 M detector on the beamline BL-5A at the Photon Factory (PF, Tsukuba, Japan). Diffraction images were captured every 0.50° with a total rotation range of 180°. Data with 1.60 Å resolution were integrated using XDSCitation13 and subsequently processed with SCALACitation14 from the CCP4 suiteCitation15. The crystal belonged to the space group P41212, with unit cell parameters of a = b = 67.80 Å, c = 141.38 Å, α = β = γ = 90°. Processed data were phased by the molecular replacement method using MOLREPCitation16 with the coordinates of RTA with N-(N-(pterin-7-yl)carbonylglycyl)-L-phenylalanine (PDBID: 4HUO)Citation10. Model building and electron density map inspections were performed using COOTCitation17. Following model building, refinement was performed using REFMAC5Citation18. Clear residual electron density was assigned to one 7PCGY, two sulphate anions, and water molecules in the final model. The model was refined to a resolution of 1.60 Å and had an R factor of 21.47% and a Rfree of 23.23%. The final model was analysed using PROCHECKCitation19, which resulted in a Ramachandran plot with 99.2% of the residues in favoured, 0.8% is allowed, and 0% in disallowed regions. The crystallographic statistics are presented in . The protein structures were prepared using CCP4MGCitation20. The atomic coordinates and structural factors were deposited in the Protein Data Bank as PDB entry 8I7P.
Table 1. Data collection, processing, and refinement statisticsTable Footnote†.
Results and discussion
The inhibitor 7PCGY was synthesised using a previously reported procedure: an ester-amide exchange reaction between 7MCP and unprotected glycyl-L-tyrosine (Gly-Tyr) in the presence of DBU in methanol ()Citation10,Citation12. RTA crystals with 7PCGY were prepared under the same conditions as those for preparing the crystals of 7PCGF/RTA complexesCitation10,Citation21, and an X-ray diffraction experiment with the crystals was performed. The resulting three-dimensional structure of the complex is shown in . The active site of RTA consists of two binding pockets: one is the catalytic primary pocket where A4324 is captured and degraded, and the other is a slightly larger secondary pocket which accommodates the guanine residue adjacent to A4324. These two pockets are separated by Tyr80, and the A4324 is captured between Tyr123 and Tyr80 by aromatic interactions in the primary pocket, whereas the guanine residue forms a π-π stacking with Tyr80 to be placed in the secondary pocketCitation3.
Figure 3. X-ray crystal structures of ricin toxin A chain (RTA) with or without the pterin-based inhibitors. (A) Omit map for bound N-(pterin-7-carbonyl)glycyl-L-tyrosine (7PCGY). Fo–Fc electron density (shown with black baskets) contoured to 2σ showing evidence for 7PCGY bound to this region. The omit map was generated by removing the ligand from the structure. This image was created using PyMOLCitation27. (B) Surface representation of the 7PCGY/RTA complex. The RTA surface is shown as an atomic charge map. (C) The interactions of 7PCGY in the catalytic primary pocket of RTA observed in the crystal structure of the 7PCGY/RTA complex. Conventional hydrogen bonds are represented as cyan dashed lines, hydrogen bonds between CH and carbonyl oxygen are represented as yellow dashed lines and aromatic (π-π and T-shaped) interactions are represented as magenta dashed lines. (D) Superimposition of protein crystal structures of 7PCGY/RTA (grey) and a ligand-free RTA (PDBID: 1RTC, orange). (E) X-ray crystal structure of the 7PCGF/RTA complex (PDBID: 4HUO). Conventional hydrogen bonds are represented as cyan dashed lines, hydrogen bonds between CH and carbonyl oxygen are represented as yellow dashed lines and aromatic (π-π and T-shaped) interactions are represented as magenta dashed lines. (F) A comparison of crystal structures of 7PCGY/RTA and 7PCGF/RTA. The protein portion of 7PCGY/RTA is shown in grey and that of 7PCGF/RTA in medium blue. All image creations, except (A), and interaction detections were performed using the Discovery Studio VisualiserCitation28.
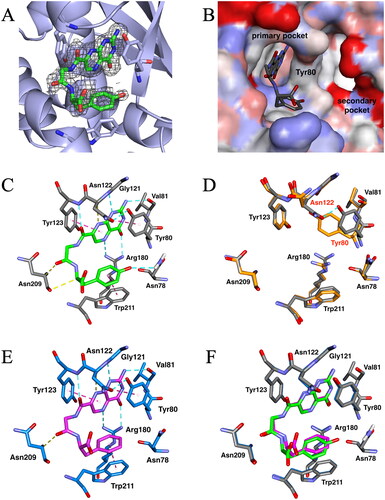
For 7PCGY, the pterin ring was bound to the catalytic primary pocket () and interacted with Val81, Gly121, Tyr123, and Arg180 via hydrogen bonds, and with Tyr123 via an aromatic (π-π) interaction ( and ). These interactions were similarly observed in the X-ray crystallography of the cocrystals of all pterin-based RTA inhibitors with RTA, regardless of inhibitory activityCitation9,Citation10,Citation21–25. The phenyl ring of the tyrosine moiety in 7PCGY interacted with Trp211 in a T-shaped manner (), thus, the conformation of Tyr80 was changed from that observed for the inhibitor-free crystal structure ()Citation26. Together with this movement, the position of Asn122 changed approaching Tyr80 to form a hydrogen bond, as if opening the gate to the secondary pocket. The same conformational changes in Tyr80 and Asn122 were observed with 7PCGF () and 7PCGFF, which exhibited high RTA inhibitory activitiesCitation10. Furthermore, the 7PCAs that were capable of interacting with the secondary pocket also induced the same rearrangements of Tyr80 and Asn122 upon binding to RTACitation21,Citation25. Based on these facts, it is strongly suggested that the conformational changes of Tyr80 and Asn122 are crucial for achieving high RTA inhibitory activity.
Table 2. Interactions between pterin-based inhibitors and RTA.
Although 7PCGY and 7PCGF differ only in the presence or absence of a hydroxy group on the phenyl group, the RTA inhibitory activity of 7PCGY is approximately three times higher than that of 7PCGF. When the RTA structure of 7PCGY/RTA was superimposed on that of 7PCGF/RTA, the two structures were perfectly comparable (). The positions of the pterin ring in 7PCGY and 7PCGF were completely overlapped, whereas the remaining dipeptide moieties in 7PCGY and 7PCGF were slightly offset from each other. A closer examination of the crystal structure of the 7PCGY/RTA complex revealed that the phenolic hydroxy group in 7PCGY interacted with Asn78 of RTA ( and ). This interaction anchored the tyrosine moiety of 7PCGY, which consequently anchored the arrangement of the other portions of 7PCGY, thereby forming an additional interaction with Asn209 ( and ). These extra interactions were not observed in 7PCGF ( and ) and probably led to the higher RTA inhibitory activity of 7PCGY than that of 7PCGF. These results indicated the conformational changes of Tyr80 and Asn122, and that further interactions between an inhibitor and RTA are required to obtain a high inhibitory activity against RTA. Thus, the factors responsible for the high RTA inhibitory activity of 7PCGY have been elucidated, achieving the objective of this study. These findings will facilitate the development of compounds with even higher RTA inhibitory activity.
Conclusion
In the present study, the previously unknown structure of 7PCGY-RTA complex was unambiguously determined using X-ray crystallography. Comparison of the crystal structure of the previously reported 7PCGF-RTA complex with that of the present 7PCGY-RTA complex reveals that the hydrogen bonding between the phenolic hydroxy group in 7PCGY and Asn78 of RTA and the conformational change of Tyr80 and Asn122 are responsible for the high RTA inhibitory activity of 7PCGY. The findings of this study will guide future optimisation of pterin-based RTA inhibitors, with the aim of increasing the inhibitory activity.
Ethical statement
This study was performed with the approval of the Photon Factory Program Advisory Committee (proposal No. 2018G520 and 2022G603).
Acknowledgement
We would like to thank the staff of the Photon Factory for their assistance with the data collection. The authors would also like to thank Editage (www.editage.jp) for the English language editing.
Disclosure statement
The authors report no conflicts of interest.
Additional information
Funding
References
- Endo Y, Tsurugi K. RNA N-glycosidase activity of ricin A-chain. Mechanism of action of the toxic lectin ricin on eukaryotic ribosomes. J Biol Chem. 1987;262(17):8128–8130.
- Endo Y, Tsurugi K. The RNA N-glycosidase activity of ricin A-chain. The characteristics of the enzymatic activity of ricin A-chain with ribosomes and with rRNA. J Biol Chem. 1988;263(18):8735–8739.
- Ribosome-inactivating Proteins: Ricin and Related Proteins. Hoboken, NJ: Wiley-Blackwell; 2014.
- Bai Y, Monzingo AF, Robertus JD. The X-ray structure of ricin A chain with a novel inhibitor. Arch Biochem Biophys. 2009;483(1):23–28.
- Endo Y, Gluck A, Wool IG. Ribosomal RNA identity elements for ricin A-chain recognition and catalysis. J Mol Biol. 1991;221(1):193–207.
- Miller DJ, Ravikumar K, Shen H, Suh J-K, Kerwin SM, Robertus JD. Structure-based design and characterization of novel platforms for ricin and shiga toxin inhibition. J Med Chem. 2002;45(1):90–98.
- Monzingo AF, Robertus JD. X-ray analysis of substrate analogs in the ricin A-chain active site. J Mol Biol. 1992;227(4):1136–1145.
- Yan X, Hollis T, Svinth M, Day P, Monzingo AF, Milne GW, Robertus JD. Structure-based identification of a ricin inhibitor. J Mol Biol. 1997;266(5):1043–1049.
- Jasheway K, Pruet J, Anslyn EV, Robertus JD. Structure-based design of ricin inhibitors. Toxins (Basel)). 2011;3(10):1233–1248.
- Saito R, Pruet JM, Manzano LA, Jasheway K, Monzingo AF, Wiget PA, Kamat I, Anslyn EV, Robertus JD. Peptide-conjugated pterins as inhibitors of ricin toxin A. J Med Chem. 2013;56(1):320–329.
- Pruet JM, Robertus JD, Anslyn EV. Acyl radical insertion for the direct formation of new seven-substituted pterin analogs. Tetrahedron Lett. 2010;51(18):2539–2540.
- Saito R, Suzuki S, Sasaki K. Pterin-7-carboxamides as a new class of aldose reductase inhibitors. Bioorg Med Chem Lett. 2016;26(20):4870–4874.
- Kabsch W. Xds. Acta Crystallogr D Biol Crystallogr. 2010;66(Pt 2):125–132.
- Evans P. Scaling and assessment of data quality. Acta Crystallogr D Biol Crystallogr. 2006;62(Pt 1):72–82.
- Winn MD, Ballard CC, Cowtan KD, Dodson EJ, Emsley P, Evans PR, Keegan RM, Krissinel EB, Leslie AG, McCoy A, et al. Overview of the CCP4 suite and current developments. Acta Crystallogr D Biol Crystallogr. 2011;67(Pt 4):235–242.
- Vagin A, Teplyakov A. Molecular replacement with MOLREP. Acta Crystallogr D Biol Crystallogr. 2010;66(Pt 1):22–25.
- Emsley P, Lohkamp B, Scott WG, Cowtan K. Features and development of coot. Acta Crystallogr D Biol Crystallogr. 2010;66(Pt 4):486–501.
- Murshudov GN, Skubak P, Lebedev AA, Pannu NS, Steiner RA, Nicholls RA, Winn MD, Long F, Vagin AA. REFMAC5 for the refinement of macromolecular crystal structures. Acta Crystallogr D Biol Crystallogr. 2011;67(Pt 4):355–367.
- Laskowski RA, Macarthur MW, Moss DS, Thornton JM. Procheck – a program to check the stereochemical quality of protein structures. J Appl Crystallogr. 1993;26(2):283–291.
- McNicholas S, Potterton E, Wilson KS, Noble MEM. Presenting your structures: the CCP4MG molecular-graphics software. Acta Crystallogr D Biol Crystallogr. 2011;67(Pt 4):386–394.
- Goto M, Higashi S, Ohba T, Kawata R, Nagatsu K, Suzuki S, Anslyn EV, Saito R. Conformational change in ricin toxin A-chain: a critical factor for inhibitor binding to the secondary pocket. Biochem Biophys Res Commun. 2022;627:1–4.
- Pruet JM, Jasheway KR, Manzano LA, Bai Y, Anslyn EV, Robertus JD. 7-Substituted pterins provide a new direction for ricin A chain inhibitors. Eur J Med Chem. 2011;46(9):3608–3615.
- Pruet JM, Saito R, Manzano LA, Jasheway KR, Wiget PA, Kamat I, Anslyn EV, Robertus JD. Optimized 5-membered heterocycle-linked pterins for the inhibition of ricin toxin A. ACS Med Chem Lett. 2012;3(7):588–591.
- Wiget PA, Manzano LA, Pruet JM, Gao G, Saito R, Monzingo AF, Jasheway KR, Robertus JD, Anslyn EV. Sulfur incorporation generally improves Ricin inhibition in pterin-appended glycine-phenylalanine dipeptide mimics. Bioorg Med Chem Lett. 2013;23(24):6799–6804.
- Saito R, Goto M, Katakura S, Ohba T, Kawata R, Nagatsu K, Higashi S, Kurisu K, Matsumoto K, Ohtsuka K. Pterin-based small molecule inhibitor capable of binding to the secondary pocket in the active site of ricin-toxin A chain. PLoS One. 2022;17(12):e0277770.
- Mlsna D, Monzingo AF, Katzin BJ, Ernst S, Robertus JD. Structure of recombinant ricin a-chain at 2.3 Angstrom. Protein Sci. 1993;2(3):429–435.
- The PyMOL Molecular Graphics System [software]. 2.5.4. Schrödinger, LLC.; 2021 [accessed 2023 Jan 20]. Available from: https://pymol.org.
- Discovery Studio Visualizer [software]. 21.1.0.20298. San Diego, U.S.A.: Dassalt Systems BIOVIA; 2020 [accessed 2022 May 23]. Available from: https://www.3ds.com/products-services/biovia/products/molecular-modeling-simulation/biovia-discovery-studio/visualization/.