Abstract
The urgent demand for effective countermeasures against metallo-β-lactamases (MBLs) necessitates development of novel metallo-β-lactamase inhibitors (MBLIs). This study is dedicated to identifying critical chemical moieties within previously developed MBLIs, and critical MBLs should serve as the target in MBLI evaluations. Using the Preferred Reporting Items for Systematic Reviews and Meta-Analyses (PRISMA), a systematic literature analysis was conducted, and the NCBI RefSeq genome database was exploited to access the abundance profile and taxonomic distribution of MBLs and their variant types. Through the implementation of two distinct systematic approaches, we elucidated critical chemical moieties of MBLIs, providing pivotal information for rational drug design. We also prioritised MBLs and their variant types, highlighting the imperative need for comprehensive testing to ensure the potency and efficacy of the newly developed MBLIs. This approach contributes valuable information to advance the field of antimicrobial drug discovery.
Introduction
Antibiotic resistance has been recognised as a pressing health concern, posing substantial threats characterised by diminishing efficacy and potency of antibiotics against bacterial infectious diseasesCitation1. The challenge to antibiotic efficacy has persisted since their first discovery, exacerbated by the continuous emergence and rapid dissemination of antibiotic resistance genes (ARGs) across One Health sectorsCitation2. Among β-lactam antibiotics, including penicillins, cephalosporins, monobactam, and carbapenems, the latter is crucially employed as one of the last-resort antibiotics against infectious diseases caused by Gram-negative bacteriaCitation3. Carbapenem resistance is primarily caused by the presence of carbapenemases, responsible for enzymatic inactivation of the carbapenemsCitation4. Carbapenemases consist of some of serine β-lactamases (SBLs) of Ambler classes A and D, and all of class B β-lactamases categorised as Zn (II)-dependent metalloenzymesCitation5. Class B β-lactamases represent a class of zinc-binding proteins where the hydroxide ion, attacking nucleophile, is activated and positioned by Zn (II) cations in the active site. This mechanism is clearly distinguished from that of SBLsCitation4. Thus, all metallo-β-lactamases (MBLs) belong to the class B β-lactamases. Zinc ion(s) coordination in the active site is crucial for their activity, though zinc coordination and residues involved in the active sites vary among MBL subclasses (B1, B2, and B3)Citation5. Among these subclasses, the rapid dissemination of subclass B1 MBLs, including New Delhi MBL (NDM), Verona integron-encoded MBL (VIM), and imipenemase (IMP), coupled with the continuous emergence of novel variant types of these MBLs, has intensified the menace posed by MBLsCitation6. Moreover, the absence of clinically approved inhibitors for MBLs is a notable challenge. In contrast, while some serine β-lactamase inhibitors (SBLIs) are used in clinical settings, their unsuitability as MBL inhibitors (MBLIs) is due to distinct mechanisms and active sites between SBLs and MBLsCitation6,Citation7.
In the past few decades, substantial efforts have been dedicated to developing effective MBLIs as a countermeasure for carbapenemase-producing pathogensCitation4,Citation7. Notably, three candidates including taniborbactam (VNRX-5133), are currently undergoing clinical trialsCitation7–10. Recently comprehensive review articles have excellently summarised the characteristics of MBLs, challenges in MBLI development, breakthroughs in drug development, and the classification of MBLIs along with their inhibition spectraCitation4,Citation7,Citation11,Citation12. However, there is a knowledge gap concerning MBLIs at the level of chemical moieties, indicating urgent need for comprehension of the correlation between chemical moieties and their corresponding inhibited MBLs. This insight is crucial for discerning critical chemical moieties, facilitating rational drug design. Furthermore, understandings on target MBLs are essential to mitigate the risk of encountering unexpected variants that may not be effectively inhibitedCitation13.
This study aims to provide essential information for the ongoing development and evaluation of MBLIs. To achieve this objective, we employed two distinct methodologies: a systematic literature analysis using the Preferred Reporting Items for Systematic Reviews and Meta-Analyses (PRISMA) and a genome database analysis using the NCBI RefSeq database. The systematic literature analysis facilitated a comprehensive understanding of critical chemical moieties of developed MBLIs and potent candidates. This analysis also delineated the inhibition spectra of these MBLIs against various MBL families and their variant types. Concurrently, the genome analysis provided abundance profiles and taxonomic distribution of MBLs at the variant type level. The approaches revealed pivotal information guiding the selection of critical chemical moieties in drug development and provided a list of MBLs which should be prioritised for testing in the evaluation of MBLIs.
Materials and methods
Systematic literature analysis
The systematic literature analysis of MBLIs adhered to the PRISMA guidelinesCitation14. Comprehensive searches were conducted across scientific literature databases, including PubMed, MEDLINE, and Embase, using the keywords “metallo-β-lactamase(s)” and “inhibitor(s)”. The searches were confined to titles and abstracts of literatures published since 2010. Initially, a total of 1,059 non-redundant literatures were identified. Subsequently, 613 records including review articles, articles without full-text, articles without β-lactamase inhibitor data and those deficient of useful information, were excluded during the screening procedures. After the screening, 446 articles remained, of which 220 articles that did not report antibacterial therapy results and focused on the application of previously developed SBLIs to MBLs or test of MBLIs against SBLs were eliminated. This resulted in a dataset of 226 scientific literatures for the systematic analysis ().
Figure 1. PRISMA (Preferred Reporting Items for Systematic Review and Meta-Analysis) flow diagram illustrating the study selection process for MBLs and MBLIs.
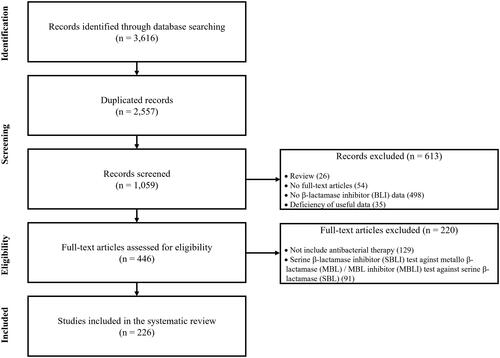
Previous studies have undertaken the classification of MBLIs based on critical chemotypes for the essential moieties involved in MBL binding and inhibition, such as carboxylate, nitrogen-containing cyclic, sulphur-containing, nitrogen/sulphur-containing cyclic, phosphonate, and boronate moietiesCitation4,Citation11,Citation12,Citation15. Referring to these classifications, our investigation focused on identifying essential chemical moieties based on the most potent and effective MBLIs in the selected 226 literatures (Table S1). Other chemical structures conferring distinct inhibition mechanisms, such as chelating, metal replacement, covalent modification, and allosteric inhibition, were also surveyed in the dataset (Table S1). Furthermore, MBLIs were categorised into five types based on the mechanism of inhibitionCitation4,Citation16. MBLIs of 138 studies out of 226 studies were categorised based on the type classification according to the previously report (Table S1)Citation7. MBLs that were tested for evaluation of MBLIs in these studies, were surveyed and among those, only MBLs inhibited by MBLIs were regarded as target MBLs (Table S1). The analyses postulated critical chemical moieties in MBLIs, inhibition types of MBLIs, and inhibited target MBLs. The complete sets of chemical moieties of MBLI and MBL pairs, encompassing all instances of MBL inhibition in the literatures, were counted and used for comparison.
Genome database analysis
Protein sequences of MBLs were extracted from the Comprehensive Antibiotic Resistance Database (CARD, https://card.mcmaster.ca/)Citation17. The information included MBL subclass, family, and variant type number. All these sequences were used to construct the Maximum-Likelihood phylogenetic tree of MBLs, employing JTT substitution model and bootstrapping, implemented in MEGA softwareCitation18. The clustered group of protein sequences in the tree was assigned to a specific MBL clade, with the majority aligning with MBL family classification. To search for MBLs in the NCBI RefSeq genome database (release 221, November 2023), a representative protein sequence for each phylogenetic clade (or family), demonstrating the highest average similarity to all other sequences within the clade (or family), was selected as a query protein sequence for Tblastn (https://blast.ncbi.nlm.nih.gov/Blast.cgi?PROGRAM=tblastn&PAGE_TYPE=BlastSearch&BLAST_SPEC=&LINK_LOC=blasttab&LAST_PAGE=tblastn) search against the database. The sequence identity criterion for MBL family was determined by establishing a cut-off identity which was the lowest identity value in comparison of all of variant types within family. A sequence coverage criterion of 90% was fixed to exclude short fragment MBLs. Signal sequences of NDM, VIM and IMP, were also excluded. Extracted MBLs from the database were identified using variant type sequence information in the CARD. Only identical sequence was assigned to a specific MBL variant type.
Information for genus-level taxonomic origin of assigned MBLs was obtained using the NCBI RefSeq genome database annotation. Variant types were found in the genome database within each family were used to construct a Maximum-Likelihood phylogenetic treeCitation18. To calculate the frequency of the variation in each amino acid residue of MBL, the Shannon index for each residue was calculated and displayedCitation19. Interactive Tree of Life (iTOL) tool was used to visualise multiple panel figures, including MBL phylogenetic tree, taxonomic clustering based on the NCBI taxonomy, taxonomic distribution heatmap (presence or absence), and abundance bar chartCitation20.
Results
Systematic literature analysis
MBLI types and profiles of their chemical moieties
A total of 226 scientific literatures were selected and employed for a comprehensive exploration of the chemical moieties and inhibition mechanisms of MBLIs and their target MBLs. These records encompass the identification of novel MBLI candidates and the validation of the candidates. Various approaches, such as virtual screeningCitation21–25, high-throughput screening of chemical librariesCitation26–28, fragment-based drug discoveryCitation29–32, natural compound screeningCitation33,Citation34, structure-activity relationship analysisCitation35,Citation36, and repurposing approved drugsCitation37–39, have been employed to elucidate novel MBLI scaffolds and their improved derivatives. Microbiological assessment, enzyme kinetic analyses, molecular docking studies, and investigations using animal models were conducted to access the potency of MBLIs. Recent efforts have endeavoured to categorise MBLIs based on their essential chemical structuresCitation4,Citation11,Citation12. In accordance with the categorizations, the chemical moieties in the selected scientific literatures were delineated as follows: those generally implicated in competitive inhibition, such as carboxylate, nitrogen-containing cyclic, sulphur-containing, nitrogen/sulphur-containing cyclic, boronate, and phosphonate moieties, were surveyed. The chemical structures of chemical moieties incorporated in MBLIs are displayed in . Additionally, other chemical structures conferring distinct inhibition mechanisms, including chelating, metal replacement, covalent modification, and allosteric inhibition, were explored.
Moreover, a recent publication classified MBLIs into five types based on their binding modes in the active site and zinc coordinationCitation7. Type I MBLIs involve chemicals in coordination and/or bridging between zinc ion(s) as zinc-binding pharmacophores. Type II, III, and IV MBLIs mimic the binding modes between MBLs and β-lactams as the initial binding, tetrahedral intermediate complex, and product binding in MBL-hydrolysis reactions, respectively. Type V MBLIs stand out, as they degrade or modify the metal ion binding active sites, incorporating features such as zinc chelation, covalent modification of metal-binding site residues, and zinc replacementCitation7.
In our investigation, we delved into the classification of MBLI types within the literatures (). Notably, type V MBLIs emerged as the most frequently reported, attributed to frequent chelator studies, as they displayed broad-spectrum inhibition activity across various MBLsCitation4. A fungal metabolite aspergillomarasmine A (AMA), Ca-EDTA, 1,4,7-triazacyclononane-N,N′,N″-triacetic acid (NOTA), and dipicolinic acid have been developed and validated as chelator MBLIsCitation40–52. Following type V, type I MBLIs was identified as the most prevalent. Within type I MBLIs, characterised by zinc-binding pharmacophores, a diverse array of chemical moieties was encompassed within this category. Type III MBLIs, which include bicyclic boronate inhibitors such as taniborbactam (VNRX-5133), xeruborbactam (QPX7728), and QPX7831, have received frequent attention, because these represent the only three MBLIs currently undergoing clinical trialsCitation8–10,Citation53–60. Type II and IV have received comparatively less investigation in the selected literaturesCitation16,Citation22,Citation36, Citation61–64 ().
Figure 3. Chemical moieties of MBLIs and their target MBLs in the systematic literature analysis. (A) Composition of MBLIs categorised by the classification of inhibitor types and chemical moieties, and composition of MBLs according to subclasses (B1, B2, and B3) and families such as NDM; (B) Pairs of chemical moieties and inhibited MBL variant types in the literatures were counted as cases and their counted amounts were displayed as a heatmap and bar charts, based on MBL variant types used for targets of efficacious MBLIs and chemical moieties of effective MBLIs.
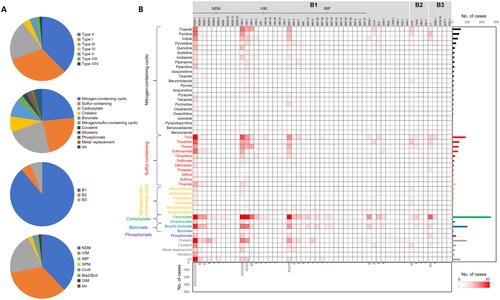
We scrutinised chemical characteristics of competitive MBLIs in the literatures at the chemical moiety-level. Nitrogen-containing cyclic, sulphur-containing, and carboxylate moieties emerged as the most prevalent (). Among nitrogen-containing cyclic moieties, triazole, pyridine, indole, and pyrrolidine were the most frequently found in MBLIs. Triazole was predominantly found in type I MBLIs such as 1,2,4-triazole-3-thione and azolylthioacetamide compoundsCitation65–69, while it was also identified in type II inhibitors such as NH-1,2,3-triazoleCitation60. Pyridine moiety was identified in type I, III, and V MBLIsCitation70–78. Indole moiety serves as an essential component in indole 2-carboxylate (type II)Citation16. Pyrrolidine moiety was commonly found in type I MBLIs together with thiol and carboxylate moietiesCitation79–84. Carboxylate moiety was identified across all types, with predominant presence in type I as zinc coordination moiety and in type III as part of bicyclic boronate drugs (taniborbactam and xeruborbactam). Dicarboxylates were detected in maleic acid derivative (ME1071) and phthalic acid derivativesCitation85–89. Concerning sulphur-containing moieties, the majorities were found in type I MBLIs, suggesting their general involvement as zinc-binding pharmacophores. Thiol emerged the most frequently utilised sulphur-containing moiety for MBLIs exemplified by captopril, thienolate, and mercaptoacetateCitation81,Citation82,Citation84,Citation90. Similar to thiol moiety, thioether were found in type I MBLIs and some of them were also present in type II and III MBLIsCitation62,Citation66,Citation91. Sulphonamide was frequently found as a constituent of type IV inhibitors, exemplified by ANT431 and ANT2681 ()Citation34,Citation35, Citation92,Citation93.
Moieties containing both nitrogen and sulphur within cyclic structure are less commonly found in MBLIs. However, thiazole and thiazolidine were identified in certain MBLIs, such as thiazole in ANT431 (type IV) and thiazolidine in bisthiazolidine and 2-mercaptomethyl-thiazolidinesCitation34,Citation35,Citation92,Citation94–96. Boronate and phosphonate moieties play a role in the binding mode mimicking the tetrahedral transition stateCitation4,Citation7. Given the active researches on type III MBLIs, such as taniborbactam and xeruborbactam, bicyclic boronate as their core chemical structure showed a relatively high frequency in the literatures. The presence of the phosphonate moiety was less common and was found in phospholactam and mercaptophosphonate MBLIsCitation97,Citation98. Other chemical structures conferring distinct inhibition mechanisms, such as chelator, metal replacement, covalent, and allosteric inhibition, generally belong to type V MBLIs, and they exhibit diverse chemical structures rather than the presence of conserved chemical moiety ()Citation4,Citation7.
Tested target MBLs for evaluation of MBLIs
Considering the diverse families of MBLs and numerous variant types within each family, an extensive array of MBL variant types exists, each displaying distinct β-lactam resistance spectra and inhibition patterns by MBLIsCitation5. This underscores the critical importance of selecting the appropriate MBLs for MBLI evaluation to ensure drug efficacy. In our analyses, we identified MBLs successfully inhibited by MBLIs (). Subclass B1 MBLs emerged as the predominant inhibition targets. Within this subclass, NDM, VIM, and IMP collectively accounted for over 93% of subclass B1 MBLs tested (). This suggests that these MBLs, known for their horizontal gene transferCitation6, are suitably employed for evaluating MBLIs. In subclass B2, ImiS and CphA MBLs were frequently employed, while L1 MBLs constituted the primary target in subclass B3 (). Generally, these B2 and B3 MBLs were used to elucidate inhibition spectra of developed NDM, VIM, and IMP inhibitors for other subclasses. However, it is noteworthy that among the numerous variant types of NDM, VIM, and IMP, only a limited number of variants have been used for MBLI evaluation. Among subclass B1 MBL variant types, only NDM-1, VIM-2, VIM-1, VIM-4, and IMP-1 were frequently tested, while the others showed very low frequency in the literatures ().
MBLs and MBLI moieties
Pairs of chemical moieties and inhibited MBL variant types systematically tallied to elucidate the correlation between chemical moieties and MBL variant types. The analyses yielded insights into the involvement of each chemical moiety in inhibition spectra, revealing moieties (notably carboxylate and bicyclic boronate) that were involved in the broad spectra (). Most chemical moieties demonstrated efficacy to specific MBL families or types. For instance, thiol and triazole moieties exhibited effective inhibition against VIM variant types but were limitedly effective against NDM-1 among NDM variant types (). Regarding MBL variant types, inhibition was observed across various chemical moieties for NDM-1, VIM-2, VIM-1, and IMP-1, while inhibition of other variants was not consistently verified. As an example, NDM-9 variant exhibiting the reduced inhibition by taniborbactam was shown to be inhibited in only three studies, employing an azetidine moiety and two phytochemicals (magnolol and fisetin) ()Citation99–101.
Genome database analysis
Abundance profiles and taxonomic distribution of MBLs
Based on the literature analysis, it is evident that a limited number of MBLs have been used for MBLI evaluation. Considering the evolving inhibition spectra resulting from mutations in MBLsCitation5, the current approaches, which primarily focused on representative type(s) of each MBL family (typically centred on NDM-1 for NDMs, VIM-2 and VIM-1 for VIMs, and IMP-1 for IMPs), requires reconsideration. While the literature analysis is valuable for understanding the important chemical moieties in MBLIs and their inhibition capacity, it may not provide the actual MBLs that should be urgently studied as targets. To identify critical and urgent MBL targets for MBLI development, elucidation of critical MBL families and their variant types through understanding their abundance profiles and taxonomic distribution in the NCBI RefSeq genome database was conducted.
As of November 2023 (v3.2.8), a total of 440 MBLs were deposited in the CARD, and they were clustered into 91 distinct clades, which predominantly aligned with family classification. A phylogenetic tree exhibited the clear distinctions among subclasses, with the length of collapsed branches indicating the number of types within each family (). Initially, we profiled the total abundance of MBL family proteins deposited in the NCBI RefSeq database. NDM emerged as the most abundant, followed by Bla2/BcII clade, IMP, VIM, CphA, BJP, BlaB, GOB, IND/CGB clade, CfiA, CAR, and L1 (each exceeding 100 proteins in the database). With the exception of CphA (B2), BJP (B3), GOB (B3), CAR (B3), and L1 (B3), the majority belonged to subclass B1. Notably, Bla2/BcII, CphA, BJP, BlaB, GOB, CfiA, CAR, and L1, while abundant, exhibited the presence only in a single genus, Bacillus, Vibrio, Aeromonas, Bradyrhizobium, Elizabethkingia, Elizabethkingia, Bacteroides, Pectobacterium, and Stenotrophomonas, respectively, suggesting intrinsic resistance without dissemination (). In the case of proteins belonging to IND/CGB clade, they were distributed in two genera (Chryseobacterium and Elizabethkingia), but most of the them were found in Chryseobacterium (98%) ().
Figure 4. Abundance profiles of MBL families deposited in the NCBI RefSeq database according to their respective host genera. (A) The taxonomic distribution and abundance of MBLs at the genus and family levels. A total of 81 genera were observed as carriers of MBLs, and their families were displayed above the genus names. The phylogenetic tree of MBLs was constructed based on all MBL protein sequences retrieved from Comprehensive Antibiotic Resistance Database using the Maximum-Likelihood method with 500 bootstrap repetitions. The clustered group of protein sequences in the tree was assigned to a specific MBL clade, with the majority aligning with the classification of MBL families. The horizontal length of an isosceles triangle symbol reflects the proportion of the collapsed variant types’ number. Each subclass of MBLs is differentiated by a specific colour label (red, subclass B1; yellow, subclass B2; blue, subclass B3). Bar charts represent the abundance of each MBL family. Major MBL families (more than 100 proteins) are highlighted with red labels; (B) Taxonomic compositions of the MBL families.
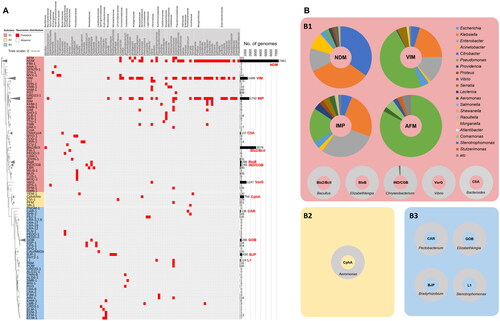
NDM, VIM, and IMP belonging to subclass B1, displayed high abundance and a broad taxonomic distribution across 29, 27, and 24 genera belonging to diverse families, respectively. NDM was dominantly present in Escherichia (35%) and Klebsiella (33%), Enterobacter (12%), and Acinetobacter (9%). In the case of VIM, Pseudomonas occupied 47% of total VIM proteins, and followed by Klebsiella (20%) and Enterobacter (14%). IMP are distributed mainly in Enterobacter (30%), Klebsiella (25%), and Pseudomonas (18%). These distributions indicate critical importance of NDM, VIM, and IPM as target MBLs for MBLI development. These findings align with the literature analyses ( and ).
Apart from these three MBLs, others were found in only 1–3 genera which are taxonomically close, indicating that these MBLs are presumed as not mobile ARGs in a current status. Remarkably, AFM demonstrated a broader distribution across 7 genera, despite having only 41 proteins in the database. Among these proteins, 34 proteins were found in Pseudomonas (family Pseudomonadaceae), while others were found in Commamonas (family Comamonadaceae, 2 protein), Stutzerimonas (family Pseudomonadaceae, 1 protein), Achromobacter (family Alcaligenaceae, 1 protein), Bordetella (family Alcaligenaceae, 1 protein), Aeromonas (family Aeromonadaceae, 1 protein), and Stenotrophomonas (family Xanthomonadaceae, 1 protein), revealing the presence of the ARG across broad taxa. It indicates that AFM should be considered a crucial target MBL for MBLI development, alongside NDM, VIM, and IMP (). As of November 2023, only four variant types have been reported for AFMs in BLDB (http://bldb.eu/)Citation102. Among these variants, only AFM-1 exhibited the distribution across the seven genera whereas the others were exclusively found in Pseudomonas, indicating that AFM-1 represents a disseminated variant type within this family.
Abundance profiles and taxonomic distribution of variant types of NDM, VIM, and IMP
NDM, VIM, and IMP were shown to be the most abundant and prevalent MBLs in the database (), thus their variant types were scrutinised to postulate critical variant types of them. In the case NDM, NDM-1 emerged as the most frequently detected variant, and NDM-5 also exhibited high abundance in the database. Mutations in NDM-5 (V88L, M154L, and A233V) compared to NDM-1, were conserved in many other variants, highlighting them as major polymorphic residues (). NDM-1 and NDM-5 were found in 29 and 12 genera, emphasising their significance as mobile ARGs. The total number of NDM-1 and NDM-5 proteins accounted for more than 93% of detected NDMs in the database. These findings suggest that NDM-5 should be recognised as a critical NDM variant together with NDM-1. Among the less abundant types, NDM-7 (2.1%), NDM-9 (1.6%), NDM-4 (0.9%), and NDM-14 (0.5%) were found frequently, albeit at lower levels compared to two major types. Unique alterations such as D130N, E152K, and D130G were identified in NDM-7, NDM-9, and NDM-14, respectively (). Notably, NDM-14 was exclusively found in Klebsiella, whereas NDM-7, NDM-9, and NDM-4 were distributed across 5, 4, and 3 genera, respectively. Their proportions in genera were depicted as a composition of taxonomic origin, revealing that NDM-1, NDM-5, NDM-7, NDM-9, and NDM-4 are mobile ARGs of priority (). Considering their abundance, unique variations, and taxonomic distribution, these five NDM variants are suggested as critical MBLs for use in MBLI development. Notably, among critical NDM variant types, only NDM-1 and NDM-9 were found in Acinetobacter, indicating that NDM-9 is a critical NDM variant that should be concerned although with relatively low prevalence ().
Figure 5. Abundance profiles, phylogeny, polymorphisms, and taxonomical compositions of NDM variant types deposited in the NCBI RefSeq database. (A) Abundance profiles, phylogeny, and polymorphisms of NDM variant types. The binary chart displays distribution of polymorphic residues across all NDM variant types. Heat map exhibits the taxonomic distribution of NDM proteins at the genus levels. Abundance of each variant type was displayed using a bar chart. The phylogenetic tree of NDM proteins was constructed based on all of NDM protein sequences retrieved from Comprehensive Antibiotic Resistance Database using the Maximum-Likelihood method with 500 bootstrap repetitions. NDM-1, the most abundant and most prevalent NDM variant type, was selected as the reference protein for comparison of polymorphic residues. Conserved and mutated polymorphic residues of other NDM proteins except for signal sequence were displayed as empty and filled circle, respectively. The actual amino acid residue of mutated site in each NDM family protein was represented in the circles with white letters. A total of 29 genera were observed to contain NDM variant types, and were utilised for assess their distribution across the genera in heat map. Bar charts represent the abundance of each NDM variant types. Major NDM families (more than 40 proteins) are highlighted with red labels; (B) Shannon diversity indices of each amino acid residue of all NDM variant types deposited in the NCBI RefSeq database. (C) Taxonomic compositions of the major NDM variant types.
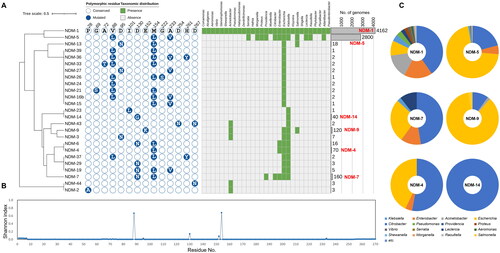
The phylogenetic tree of VIM variant types clearly demonstrates two distinct clades, each represented by VIM-1 and VIM-2 (). They accounted for more than 78% of total VIMs, and the majority of polymorphisms of VIM was attributed to their sequence difference (). VIM-1 and VIM-2 were found in 18 and 11 genera across various families. Beside these two major types, VIM-4 and VIM-5 were also frequently detected, distributed across 15 and 6 taxa, respectively. In contrast, the other minor types were found in 1 or 2 genera. VIM-4 and VIM-5 are variants of VIM-1, and VIM-5 exhibited unique sequence variations, such as A128K, E202A, and K257T (). Analyses of the composition of taxonomic origin revelled that VIM-2, VIM-1, VIM-4, and VIM-5 are mobile ARGs (). Regarding their abundance, the presence of unique variations, and broad taxonomic distribution, these four VIM variants are suggested as critical MBLs that should be used as targets in the MBLI development. For instance, VIM-5 was frequently found in Acinetobacter (55%), compared to other critical VIM variant types, revealing the importance of this variant type ().
Figure 6. Abundance profiles, phylogeny, polymorphisms, and taxonomical compositions of VIM variant types deposited in the NCBI RefSeq database. (A) Abundance profiles, phylogeny, and polymorphisms of VIM variant types. The binary chart displays distribution of polymorphic residues across all VIM variant types. Heat map exhibits the taxonomic distribution of VIM proteins at the genus levels. Abundance of each variant type was displayed using a bar chart. The phylogenetic tree of VIM proteins was constructed based on all of VIM protein sequences retrieved from Comprehensive Antibiotic Resistance Database using the Maximum-Likelihood method with 500 bootstrap repetitions. VIM-2, the most abundant and most prevalent VIM variant type, was selected as the reference protein for comparison of polymorphic residues. Conserved and mutated polymorphic residues of other VIM proteins except for signal sequence were displayed as empty and filled circle, respectively. The actual amino acid residue of mutated site in each VIM family protein was represented in the circles with white letters. A total of 27 genera were observed to contain VIM variant types, and were utilised for assess their distribution across the genera in heat map. Bar charts represent the abundance of each VIM variant types. Major VIM families (more than 38 proteins) are highlighted with red labels; (B) Shannon diversity indices of each amino acid residue of all VIM variant types deposited in the NCBI RefSeq database. (C) Taxonomic compositions of the major VIM variant types.
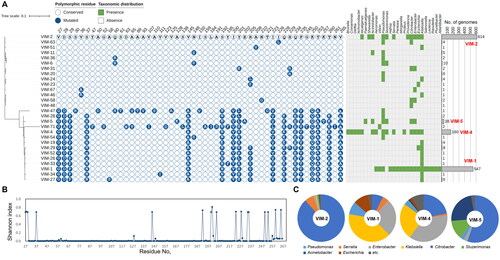
Compared to NDM and VIM, IMP displayed less conserveness throughout protein sequences (). Numerous polymorphic residues were present, and many of them conferred high values of Shannon index (). IMP-4 and IMP-1 were the most abundant types, collectively occupying more than 68% of total IMP proteins, and present in 12 and 18 genera, respectively (). In addition to two major types, there were four types (IMP-27, IMP-6, IMP-8, and IMP-26) demonstrating relatively high abundance and a broad taxonomic distribution, each represented by more than 30 proteins across more than 4 genera (). Along with IMP-4 and IMP-I, these IMP variant types also should be considered target MBLs in MBLI development.
Figure 7. Abundance profiles, phylogeny, polymorphisms, and taxonomical compositions of IMP variant types deposited in the NCBI RefSeq database. (A) Abundance profiles, phylogeny, and polymorphisms of IMP variant types. The binary chart displays distribution of polymorphic residues across all IMP variant types. Heat map exhibits the taxonomic distribution of IMP proteins at the genus levels. Abundance of each variant type was displayed using a bar chart. The phylogenetic tree of IMP proteins was constructed based on all of IMP protein sequences retrieved from Comprehensive Antibiotic Resistance Database using the Maximum-Likelihood method with 500 bootstrap repetitions. IMP-1, the most abundant and most prevalent IMP variant type, was selected as the reference protein for comparison of polymorphic residues. Conserved and mutated polymorphic residues of other IMP proteins except for signal sequence were displayed as empty and filled circle, respectively. The actual amino acid residue of mutated site in each IMP family protein was represented in the circles with white letters. A total of 24 genera were observed to contain IMP variant types, and were utilised for assess their distribution across the genera in heat map. Bar charts represent the abundance of each IMP variant types. Major IMP families (more than 50 proteins) are highlighted with red labels; (B) Shannon diversity indices of each amino acid residue of all IMP variant types deposited in the NCBI RefSeq database. (C) Taxonomic compositions of five major IMP variant types and IMP-26.
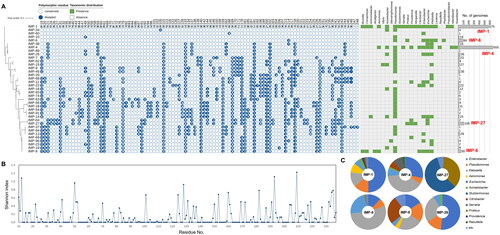
Discussion
In this study, we employed two distinct approaches to decipher critical chemical moieties required for the development of effective MBLIs and to identify MBLs which should be prioritised as target MBLs for the MBLI evaluation. Assessments of the prevalence of chemical moieties in the literatures were based on the previous classification of chemotypes of MBLIs, usually concerned with the essential moieties involved in MBL binding and inhibitionCitation4,Citation11,Citation12,Citation15. The analyses unveiled both narrow and broad-spectrum moieties, providing pivotal information crucial for rational drug design ().
In the case of MBLs used in the literature, the survey revealed that only a limited number of MBLs have been used in evaluating MBLIs. Out of tremendous variant types of major subclass B1 MBLs (NDM, VIM, and IMP), only five variant types (NDM-1, VIM-2, VIM-1, VIM-4, and IMP-1) have been mainly used for evaluation of the MBLIs. Although some studies focused on mutations or variant types and their inhibition by MBLIsCitation42,Citation53,Citation103,Citation104, most of studies employed only major variants to evaluate MBLIs. This observation may account for instances where certain MBL variant types, such as NDM-9, were not tested in the development process of taniborbactam. A recent study corroborated this, demonstrating that the pre-existing NDM variant, NDM-9, is not inhibited by taniborbactam due to unique sequence alteration (E152K)Citation13. Furthermore, a follow-up study revealed that NDM-9 was successfully inhibited by a different bicyclic boronate MBLI, xeruborbactamCitation105. These results indicate that critical variant types should be elucidated and applied to the MBLI tests, indicating that proper criteria and approach are demanded for selection. Based on the insights gained from literature analyses, we hypothesise that proposing critical MBLs and their variants for MBLI evaluation could enhance the efficacy of novel MBLIs and ensure their effectiveness. Leveraging the genome database analyses regarding prevalence and taxonomic distribution of MBLs, we have compiled a comprehensive list of critical MBLs and their variant types, including some that were previously underestimated in the literatures (). These MBL variant types are designated as targets for the extended-spectrum MBLI development.
Considering overall landscape of MBLs and their variant types, variants exhibiting the high prevalence and taxonomic distribution must be classified as potentially significant variants which should be used for MBLI evaluations (). This underscores the importance of considering variant types of NDM, VIM and IMP suggested in this study as target for MBLI evaluations.
Furthermore, AFM family was initially reported in Alcaligenes faecalisCitation106, and now they are distributed across various genera, suggesting that it should be under strict surveillance. AFM-1 was shown to be a major disseminated variant type within this family. However, polymorphic residues in other variant types were located in the signal sequence regionCitation107, indicating that there may not be the distinct resistance spectra against AFM variant types and the different inhibition patterns by MBLIs among the variant types. As a study in the future, it would be better (i) to conduct analyses that explore the relationship between chemical moieties and MBL families (or variant types) through enzyme-ligand docking analyses, (ii) to investigate the relative abundance of the different MBL variant types in the clinical isolates, and (iii) to reveal the impact of the chemical moieties of developed MBLIs when targeting Enterobacterales, or non-fermentors such as Pseudomonas aeruginosa or Acinetobacter baumannii.
Conclusion
Based on two systematic approaches, we propose critical chemical moieties essential for MBLIs, providing pivotal information for further rational drug design. Additionally, we identified critical MBLs and their variant types, which must undergo testing to ensure the efficacy and potent of novel MBLIs. Together with previously examined NDM, VIM, and IMP families, AFM family is also suggested as a critical MBL family to be used in the extended-spectrum MBLI evaluation. In the case of variant types for the evaluation of extended-spectrum MBLIs, NDM-5, NDM-7, NDM-9, NDM-4, VIM-4, VIM-5, IMP-4, IMP-27, IMP-6, IMP-8, IMP-26, and AFM-1 were newly selected as target MBL variant types, along with previously used variants NDM-1, VIM-2, VIM-1, and IMP-1.
Author contributions statement
JHL, S-GK and D-WK contributed to the genome database survey and analysis. JHL, S-GK, K-MJ, KS, HJ, D-WK, BCJ and SHL performed the literature search and article writing (original draft preparation). SHL contributed to the funding acquisition and supervision. All authors have read and agreed to the published version of the manuscript.
Supplemental Material
Download MS Excel (42.3 KB)Acknowledgements
We thank our colleagues, both past and present, at the National Leading Research Laboratory of Drug Resistance Proteomics, Myongji University, for sharing their insights into the concepts presented here.
Disclosure statement
No potential conflict of interest was reported by the author(s).
Data availability
None.
Additional information
Funding
References
- Ventola CL. The antibiotic resistance crisis: part 2: management strategies and new agents. P T. 2015;40(5):1–13.
- Kim DW, Cha CJ. Antibiotic resistome from the one-health perspective: understanding and controlling antimicrobial resistance transmission. Exp Mol Med. 2021;53(3):301–309.
- Papp-Wallace KM, Endimiani A, Taracila MA, Bonomo RA. Carbapenems: past, present, and future. Antimicrob Agents Chemother. 2011;55(11):4943–4960.
- Bahr G, González LJ, Vila AJ. Metallo-β-lactamases in the age of multidrug resistance: From structure and mechanism to evolution, dissemination, and inhibitor design. Chem Rev. 2021;121(13):7957–8094.
- Tooke CL, Hinchliffe P, Bragginton EC, Colenso CK, Hirvonen VHA, Takebayashi Y, Spencer J. βLactamases and β-lactamase inhibitors in the 21st century. J Mol Biol. 2019;431(18):3472–3500.
- Mojica MF, Rossi MA, Vila AJ, Bonomo RA. The urgent need for metallo-β-lactamase inhibitors: an unattended global threat. Lancet Infect Dis. 2022;22(1):e28–e34.
- Yang Y, Yan YH, Schofield CJ, McNally A, Zong Z, Li GB. Metallo-β-lactamase-mediated antimicrobial resistance and progress in inhibitor discovery. Trends Microbiol. 2023;31(7):735–748.
- Liu B, Trout REL, Chu GH, McGarry D, Jackson RW, Hamrick JC, Daigle DM, Cusick SM, Pozzi C, De Luca F, et al. Discovery of taniborbactam (VNRX-5133): a broad-spectrum serine- and metallo-β-lactamase inhibitor for carbapenem-resistant bacterial infections. J Med Chem. 2020;63(6):2789–2801. ‘
- Hecker SJ, Reddy KR, Lomovskaya O, Griffith DC, Rubio-Aparicio D, Nelson K, Tsivkovski R, Sun D, Sabet M, Tarazi Z, et al. Discovery of cyclic boronic acid QPX7728, an ultrabroad-spectrum inhibitor of serine and metallo-β-lactamases. J Med Chem. 2020;63(14):7491–7507.
- Reddy KR, Parkinson J, Sabet M, Tarazi Z, Boyer SH, Lomovskaya O, Griffith DC, Hecker SJ, Dudley MN. Selection of QPX7831, an orally bioavailable prodrug of boronic acid β-lactamase inhibitor QPX7728. J Med Chem. 2021;64(23):17523–17529.
- Wu W, Feng Y, Tang G, Qiao F, McNally A, Zong Z. NDM metallo-β-lactamases and their bacterial producers in health care settings. Clin Microbiol Rev. 2019;32(2):e00115-18.
- Li R, Chen X, Zhou C, Dai QQ, Yang L. Recent advances in β-lactamase inhibitor chemotypes and inhibition modes. Eur J Med Chem. 2022;242:114677.
- Le Terrier C, Gruenig V, Fournier C, Nordmann P, Poirel L. NDM-9 resistance to taniborbactam. Lancet Infect Dis. 2023;23(4):401–402.
- Page MJ, Moher D, Bossuyt PM, Boutron I, Hoffmann TC, Mulrow CD, Shamseer L, Tetzlaff JM, Akl EA, Brennan SE, et al. Prisma 2020 explanation and elaboration: updated guidance and exemplars for reporting systematic reviews. BMJ. 2021;372:n160.
- Chen C, Oelschlaeger P, Wang D, Xu H, Wang Q, Wang C, Zhao A, Yang KW. Structure and mechanism-guided design of dual serine/metallo-carbapenemase inhibitors. J Med Chem. 2022;65(8):5954–5974.
- Brem J, Panduwawala T, Hansen JU, Hewitt J, Liepins E, Donets P, Espina L, Farley AJM, Shubin K, Campillos GG, et al. Imitation of β-lactam binding enables broad-spectrum metallo-β-lactamase inhibitors. Nat Chem. 2022;14(1):15–24.
- Alcock BP, Huynh W, Chalil R, Smith KW, Raphenya AR, Wlodarski MA, Edalatmand A, Petkau A, Syed SA, Tsang KK, et al. CARD 2023: expanded curation, support for machine learning, and resistome prediction at the comprehensive antibiotic resistance database. Nucleic Acids Res. 2023;51(D1):D690–D699.
- Hall BG. Building phylogenetic trees from molecular data with MEGA. Mol Biol Evol. 2013;30(5):1229–1235.
- Iwai S, Chai B, Sul WJ, Cole JR, Hashsham SA, Tiedje JM. Gene-targeted-metagenomics reveals extensive diversity of aromatic dioxygenase genes in the environment. Isme J. 2010;4(2):279–285.
- Letunic I, Bork P. Interactive Tree of Life (iTOL) v5: an online tool for phylogenetic tree display and annotation. Nucleic Acids Res. 2021;49(W1):W293–W296.
- Wang X, Lu M, Shi Y, Ou Y, Cheng X. Discovery of novel New Delhi metallo-β-lactamases-1 inhibitors by multistep virtual screening. PLoS One. 2015;10(3):e0118290.
- Li GB, Abboud MI, Brem J, Someya H, Lohans CT, Yang SY, Spencer J, Wareham DW, McDonough MA, Schofield CJ. NMR-filtered virtual screening leads to non-metal chelating metallo-β-lactamase inhibitors. Chem Sci. 2017;8(2):928–937.
- Spyrakis F, Celenza G, Marcoccia F, Santucci M, Cross S, Bellio P, Cendron L, Perilli M, Tondi D. Structure-based virtual screening for the discovery of novel inhibitors of New Delhi metallo-β-lactamase-1. ACS Med Chem Lett. 2018;9(1):45–50.
- Shi C, Bao J, Sun Y, Kang X, Lao X, Zheng H. Discovery of baicalin as NDM-1 inhibitor: virtual screening, biological evaluation and molecular simulation. Bioorg Chem. 2019;88:102953.
- Wang X, Yang Y, Gao Y, Niu X. Discovery of the novel inhibitor against New Delhi metallo-β-lactamase based on virtual screening and molecular modelling. Int J Mol Sci. 2020;21(10):3567.
- Brindisi M, Brogi S, Giovani S, Gemma S, Lamponi S, De Luca F, Novellino E, Campiani G, Docquier JD, Butini S. Targeting clinically-relevant metallo-β-lactamases: from high-throughput docking to broad-spectrum inhibitors. J Enzyme Inhib Med Chem. 2016;31(sup1):98–109.
- Thomas PW, Spicer T, Cammarata M, Brodbelt JS, Hodder P, Fast W. An altered zinc-binding site confers resistance to a covalent inactivator of New Delhi metallo-β-lactamase-1 (NDM-1) discovered by high-throughput screening. Bioorg Med Chem. 2013;21(11):3138–3146.
- Betts JW, Phee LM, Abdul Momin MHF, Umland K-D, Brem J, Schofield CJ, Wareham DW. In vitro and in vivo activity of ML302F: a thioenolate inhibitor of VIM-subfamily metallo β-lactamases. Med Chem Commun. 2016;7(1):190–193.
- Cain R, Brem J, Zollman D, McDonough MA, Johnson RM, Spencer J, Makena A, Abboud MI, Cahill S, Lee SY, et al. In silico fragment-based design identifies subfamily B1 metallo-β-lactamase inhibitors. J Med Chem. 2018;61(3):1255–1260.
- Caburet J, Boucherle B, Bourdillon S, Simoncelli G, Verdirosa F, Docquier JD, Moreau Y, Krimm I, Crouzy S, Peuchmaur M. A fragment-based drug discovery strategy applied to the identification of NDM-1 β-lactamase inhibitors. Eur J Med Chem. 2022;240:114599.
- Vella P, Hussein WM, Leung EWW, Clayton D, Ollis DL, Mitić N, Schenk G, McGeary RP. The identification of new metallo-β-lactamase inhibitor leads from fragment-based screening. Bioorg Med Chem Lett. 2011;21(11):3282–3285.
- Christopeit T, Leiros HK. Fragment-based discovery of inhibitor scaffolds targeting the metallo-β-lactamases NDM-1 and VIM-2. Bioorg Med Chem Lett. 2016;26(8):1973–1977.
- Yang Y, Guo Y, Zhou Y, Gao Y, Wang X, Wang J, Niu X. Discovery of a novel natural allosteric inhibitor that targets NDM-1 against Escherichia coli. Front Pharmacol. 2020;11:581001.
- Somboro AM, Osei Sekyere J, Amoako DG, Kumalo HM, Khan R, Bester LA, Essack SY. In vitro potentiation of carbapenems with tannic acid against carbapenemase-producing enterobacteriaceae: exploring natural products as potential carbapenemase inhibitors. J Appl Microbiol. 2019;126(2):452–467.
- Davies DT, Leiris S, Sprynski N, Castandet J, Lozano C, Bousquet J, Zalacain M, Vasa S, Dasari PK, Pattipati R, et al. ANT2681: SAR studies leading to the identification of a metallo-β-lactamase inhibitor with potential for clinical use in combination with meropenem for the treatment of infections caused by NDM-producing Enterobacteriaceae. ACS Infect Dis. 2020;6(9):2419–2430.
- Leiris S, Coelho A, Castandet J, Bayet M, Lozano C, Bougnon J, Bousquet J, Everett M, Lemonnier M, Sprynski N, et al. SAR studies leading to the identification of a novel series of metallo-β-lactamase inhibitors for the treatment of carbapenem-resistant enterobacteriaceae infections that display efficacy in an animal infection model. ACS Infect Dis. 2019;5(1):131–140.
- Grigorenko VG, Khrenova MG, Andreeva IP, Rubtsova MY, Lev AI, Novikova TS, Detusheva EV, Fursova NK, Dyatlov IA, Egorov AM. Drug repurposing of the unithiol: inhibition of metallo-β-lactamases for the treatment of carbapenem-resistant Gram-negative bacterial infections. Int J Mol Sci. 2022;23(3):1834..
- Liu Y, Yang K, Jia Y, Wang Z. Repurposing peptidomimetic as potential inhibitor of New Delhi metallo-β-lactamases in Gram-negative bacteria. ACS Infect Dis. 2019;5(12):2061–2066.
- Muteeb G, Alsultan A, Farhan M, Aatif M. Risedronate and methotrexate are high-affinity inhibitors of New Delhi metallo-β-lactamase-1 (NDM-1): a drug repurposing approach. Molecules. 2022;27(4):1283.
- Sychantha D, Rotondo CM, Tehrani K, Martin NI, Wright GD. Aspergillomarasmine a inhibits metallo-β-lactamases by selectively sequestering Zn2. J Biol Chem. 2021;297(2):100918.
- King AM, Reid-Yu SA, Wang W, King DT, De Pascale G, Strynadka NC, Walsh TR, Coombes BK, Wright GD. Aspergillomarasmine A overcomes metallo-β-lactamase antibiotic resistance. Nature. 2014;510(7506):503–506.
- Rotondo CM, Sychantha D, Koteva K, Wright GD. Suppression of β-lactam resistance by aspergillomarasmine A is influenced by both the metallo-β-lactamase target and the antibiotic partner. Antimicrob Agents Chemother. 2020;64(4):e01386–19.
- Zhang J, Wang S, Wei Q, Guo Q, Bai Y, Yang S, Song F, Zhang L, Lei X. Synthesis and biological evaluation of aspergillomarasmine A derivatives as novel NDM-1 inhibitor to overcome antibiotics resistance. Bioorg Med Chem. 2017;25(19):5133–5141.
- Koteva K, King AM, Capretta A, Wright GD. Total synthesis and activity of the metallo-β-lactamase inhibitor aspergillomarasmine. Angew Chem Int Ed Engl. 2016;55(6):2210–2212.
- Bergstrom A, Katko A, Adkins Z, Hill J, Cheng Z, Burnett M, Yang H, Aitha M, Mehaffey MR, Brodbelt JS, et al. Probing the interaction of aspergillomarasmine A with metallo-β-lactamases NDM-1, VIM-2, and IMP-7. ACS Infect Dis. 2018;4(2):135–145.
- Albu SA, Koteva K, King AM, Al-Karmi S, Wright GD, Capretta A. Total synthesis of aspergillomarasmine A and related compounds: a sulfamidate approach enables exploration of structure-activity relationships. Angew Chem Int Ed Engl. 2016;55(42):13259–13262.
- Yoshizumi A, Ishii Y, Livermore DM, Woodford N, Kimura S, Saga T, Harada S, Yamaguchi K, Tateda K. Efficacies of calcium-EDTA in combination with imipenem in a murine model of sepsis caused by Escherichia coli with NDM-1 β-lactamase. J Infect Chemother. 2013;19(5):992–995.
- Aoki N, Ishii Y, Tateda K, Saga T, Kimura S, Kikuchi Y, Kobayashi T, Tanabe Y, Tsukada H, Gejyo F, et al. Efficacy of calcium-EDTA as an inhibitor for metallo-β-lactamase in a mouse model of Pseudomonas aeruginosa pneumonia. Antimicrob Agents Chemother. 2010;54(11):4582–4588.
- Zhang E, Wang MM, Huang SC, Xu SM, Cui DY, Bo YL, Bai PY, Hua YG, Xiao CL, Qin S. Nota analogue: A first dithiocarbamate inhibitor of metallo-β-lactamases. Bioorg Med Chem Lett. 2018;28(2):214–221.
- Somboro AM, Tiwari D, Bester LA, Parboosing R, Chonco L, Kruger HG, Arvidsson PI, Govender T, Naicker T, Essack SY. NOTA: a potent metallo-β-lactamase inhibitor. J Antimicrob Chemother. 2015;70(5):1594–1596.
- Chen AY, Thomas PW, Stewart AC, Bergstrom A, Cheng Z, Miller C, Bethel CR, Marshall SH, Credille CV, Riley CL, et al. Dipicolinic acid derivatives as inhibitors of New Delhi metallo-β-lactamase-1. J Med Chem. 2017;60(17):7267–7283.
- Chen AY, Thomas PW, Cheng Z, Xu NY, Tierney DL, Crowder MW, Fast W, Cohen SM. Investigation of dipicolinic acid isosteres for the inhibition of metallo-β-lactamases. ChemMedChem. 2019;14(13):1271–1282.
- Lomovskaya O, Tsivkovski R, Nelson K, Rubio-Aparicio D, Sun D, Totrov M, Dudley MN. Spectrum of β-lactamase inhibition by the cyclic boronate QPX7728, an ultrabroad-spectrum β-lactamase inhibitor of serine and metallo-β-lactamases: enhancement of activity of multiple antibiotics against isogenic strains expressing single β-lactamases. Antimicrob Agents Chemother. 2020;64(6):e00212-20.
- Nelson K, Rubio-Aparicio D, Sun D, Dudley M, Lomovskaya O. In vitro activity of the ultrabroad-spectrum-β-lactamase inhibitor QPX7728 against carbapenem-resistant enterobacterales with varying intrinsic and acquired resistance mechanisms. Antimicrob Agents Chemother. 2020;64(8):e00757-20.
- Nelson K, Rubio-Aparicio D, Tsivkovski R, Sun D, Totrov M, Dudley M, Lomovskaya O. In vitro activity of the ultra-broad-spectrum β-lactamase inhibitor QPX7728 in combination with meropenem against clinical isolates of carbapenem-resistant Acinetobacter baumannii. Antimicrob Agents Chemother. 2020;64(11):e01406-20.
- Tsivkovski R, Totrov M, Lomovskaya O. Biochemical characterization of QPX7728, a new ultrabroad-spectrum β-lactamase inhibitor of serine and metallo-β-lactamases. Antimicrob Agents Chemother. 2020;64(6):e00130-20.
- Lomovskaya O, Rubio-Aparicio D, Nelson K, Sun D, Tsivkovski R, Castanheira M, Lindley J, Loutit J, Dudley M. In vitro activity of the ultrabroad-spectrum β-lactamase inhibitor QPX7728 in combination with multiple β-lactam antibiotics against Pseudomonas aeruginosa. Antimicrob Agents Chemother. 2021;65(6):e00210-21.
- Lomovskaya O, Rubio-Aparicio D, Tsivkovski R, Loutit J, Dudley M. The ultrabroad-spectrum β-lactamase inhibitor QPX7728 restores the potency of multiple oral β-lactam antibiotics against β-lactamase-producing strains of resistant enterobacterales. Antimicrob Agents Chemother. 2022;66(2):e0216821.
- Krajnc A, Brem J, Hinchliffe P, Calvopiña K, Panduwawala TD, Lang PA, Kamps J, Tyrrell JM, Widlake E, Saward BG, et al. Bicyclic boronate VNRX-5133 inhibits metallo- and serine-β-lactamases. J Med Chem. 2019;62(18):8544–8556.
- Hamrick JC, Docquier JD, Uehara T, Myers CL, Six DA, Chatwin CL, John KJ, Vernacchio SF, Cusick SM, Trout REL, et al. VNRX-5133 (taniborbactam), a broad-spectrum inhibitor of serine- and metallo-β-lactamases, restores activity of cefepime in enterobacterales and Pseudomonas aeruginosa. Antimicrob Agents Chemother. 2020;64(3):e01963-19.
- Weide T, Saldanha SA, Minond D, Spicer TP, Fotsing JR, Spaargaren M, Frère JM, Bebrone C, Sharpless KB, Hodder PS, et al. NH-1,2,3-triazole-based inhibitors of the VIM-2 metallo-β-lactamase: synthesis and structure-activity studies. ACS Med Chem Lett. 2010;1(4):150–154.
- Wade N, Tehrani K, Brüchle NC, van Haren MJ, Mashayekhi V, Martin NI. Mechanistic investigations of metallo-β-lactamase inhibitors: strong zinc binding is not required for potent enzyme inhibition. ChemMedChem. 2021;16(10):1651–1659.
- Torelli NJ, Akhtar A, DeFrees K, Jaishankar P, Pemberton OA, Zhang X, Johnson C, Renslo AR, Chen Y. Active-site druggability of carbapenemases and broad-spectrum inhibitor discovery. ACS Infect Dis. 2019;5(6):1013–1021.
- Dai Q, Yan Y, Ning X, Li G, Yu J, Deng J, Yang L, Li GB. Ancphore: a versatile tool for anchor pharmacophore steered drug discovery with applications in discovery of new inhibitors targeting metallo-β-lactamases and indoleamine/tryptophan 2,3-dioxygenases. Acta Pharm Sin B. 2021;11(7):1931–1946.
- Legru A, Verdirosa F, Hernandez JF, Tassone G, Sannio F, Benvenuti M, Conde PA, Bossis G, Thomas CA, Crowder MW, et al. 1,2,4-Triazole-3-thione compounds with a 4-ethyl alkyl/aryl sulfide substituent are broad-spectrum metallo-β-lactamase inhibitors with re-sensitization activity. Eur J Med Chem. 2021;226:113873.
- Gavara L, Sevaille L, De Luca F, Mercuri P, Bebrone C, Feller G, Legru A, Cerboni G, Tanfoni S, Baud D, et al. 4-Amino-1,2,4-triazole-3-thione-derived schiff bases as metallo-β-lactamase inhibitors. Eur J Med Chem. 2020;208:112720.
- Yang SK, Kang JS, Oelschlaeger P, Yang KW. Azolylthioacetamide: a highly promising scaffold for the development of metallo-β-lactamase inhibitors. ACS Med Chem Lett. 2015;6(4):455–460.
- Xiang Y, Chang YN, Ge Y, Kang JS, Zhang YL, Liu XL, Oelschlaeger P, Yang KW. Azolylthioacetamides as a potent scaffold for the development of metallo-β-lactamase inhibitors. Bioorg Med Chem Lett. 2017;27(23):5225–5229.
- Liu XL, Xiang Y, Chen C, Yang KW. Azolylthioacetamides as potential inhibitors of New Delhi metallo-β-lactamase-1 (NDM-1). J Antibiot (Tokyo)). 2019;72(2):118–121.
- Feng L, Yang KW, Zhou LS, Xiao JM, Yang X, Zhai L, Zhang YL, Crowder MW. N-heterocyclic dicarboxylic acids: Broad-spectrum inhibitors of metallo-β-lactamases with co-antibacterial effect against antibiotic-resistant bacteria. Bioorg Med Chem Lett. 2012;22(16):5185–5189.
- Shin WS, Bergstrom A, Bonomo RA, Crowder MW, Muthyala R, Sham YY. Discovery of 1-hydroxypyridine-2(1h)-thione-6-carboxylic acid as a first-in-class low-cytotoxic nanomolar metallo β-lactamase inhibitor. ChemMedChem. 2017;12(11):845–849.
- Hinchliffe P, Tanner CA, Krismanich AP, Labbé G, Goodfellow VJ, Marrone L, Desoky AY, Calvopiña K, Whittle EE, Zeng F, et al. Structural and kinetic studies of the potent inhibition of metallo-β-lactamases by 6-phosphonomethylpyridine-2-carboxylates. Biochemistry. 2018;57(12):1880–1892.
- Wang Q, He Y, Lu R, Wang WM, Yang KW, Fan HM, Jin Y, Blackburn GM. Thermokinetic profile of NDM-1 and its inhibition by small carboxylic acids. Biosci Rep. 2018;38(2):BSR20180244.
- Shi XF, Wang MM, Huang SC, Han JX, Chu WC, Xiao C, Zhang E, Qin S. H(2)depda: an acyclic adjuvant potentiates meropenem activity in vitro against metallo-β-lactamase-producing enterobacterales. Eur J Med Chem. 2019;167:367–376.
- Jackson AC, Zaengle-Barone JM, Puccio EA, Franz KJ. A cephalosporin prochelator inhibits new delhi metallo-β-lactamase 1 without removing zinc. ACS Infect Dis. 2020;6(5):1264–1272.
- Thomas CA, Cheng Z, Yang K, Hellwarth E, Yurkiewicz CJ, Baxter FM, Fullington SA, Klinsky SA, Otto JL, Chen AY, et al. Probing the mechanisms of inhibition for various inhibitors of metallo-β-lactamases VIM-2 and NDM-1. J Inorg Biochem. 2020;210:111123.
- Gao H, Li JQ, Kang PW, Chigan JZ, Wang H, Liu L, Xu YS, Zhai L, Yang KW. N-acylhydrazones confer inhibitory efficacy against New Delhi metallo-β-lactamase-1. Bioorg Chem. 2021;114:105138.
- Li JQ, Sun LY, Jiang Z, Chen C, Gao H, Chigan JZ, Ding HH, Yang KW. Diaryl-substituted thiosemicarbazone: a potent scaffold for the development of New Delhi metallo-β-lactamase-1 inhibitors. Bioorg Chem. 2021;107:104576.
- Klingler FM, Wichelhaus TA, Frank D, Cuesta-Bernal J, El-Delik J, Müller HF, Sjuts H, Göttig S, Koenigs A, Pos KM, et al. Approved drugs containing thiols as inhibitors of metallo-β-lactamases: strategy to combat multidrug-resistant bacteria. J Med Chem. 2015;58(8):3626–3630.
- Wachino J, Yamaguchi Y, Mori S, Jin W, Kimura K, Kurosaki H, Arakawa Y. Structural insights into recognition of hydrolyzed carbapenems and inhibitors by subclass B3 metallo-β-lactamase SMB-1. Antimicrob Agents Chemother. 2016;60(7):4274–4282.
- Brem J, van Berkel SS, Zollman D, Lee SY, Gileadi O, McHugh PJ, Walsh TR, McDonough MA, Schofield CJ. Structural basis of metallo-β-lactamase inhibition by captopril stereoisomers. Antimicrob Agents Chemother. 2016;60(1):142–150.
- Yusof Y, Tan DTC, Arjomandi OK, Schenk G, McGeary RP. Captopril analogues as metallo-β-lactamase inhibitors. Bioorg Med Chem Lett. 2016;26(6):1589–1593.
- Tehrani KHME, Martin NI. Thiol-containing metallo-β-lactamase inhibitors resensitize resistant Gram-negative bacteria to meropenem. ACS Infect Dis. 2017;3(10):711–717.
- Ma G, Wang S, Wu K, Zhang W, Ahmad A, Hao Q, Lei X, Zhang H. Structure-guided optimization of D-captopril for discovery of potent NDM-1 inhibitors. Bioorg Med Chem. 2021;29:115902.
- Ishii Y, Eto M, Mano Y, Tateda K, Yamaguchi K. In vitro potentiation of carbapenems with ME1071, a novel metallo-β-lactamase inhibitor, against metallo-β-lactamase-producing Pseudomonas aeruginosa clinical isolates. Antimicrob Agents Chemother. 2010;54(9):3625–3629.
- Livermore DM, Mushtaq S, Morinaka A, Ida T, Maebashi K, Hope R. Activity of carbapenems with ME1071 (disodium 2,3-diethylmaleate) against Enterobacteriaceae and Acinetobacter spp. With carbapenemases, including ndm enzymes. J Antimicrob Chemother. 2013;68(1):153–158.
- Yamada K, Yanagihara K, Kaku N, Harada Y, Migiyama Y, Nagaoka K, Morinaga Y, Nakamura S, Imamura Y, Miyazaki T, et al. In vivo efficacy of biapenem with ME1071, a novel metallo-β-lactamase (MBL) inhibitor, in a murine model mimicking ventilator-associated pneumonia caused by MBL-producing Pseudomonas aeruginosa. Int J Antimicrob Agents. 2013;42(3):238–243.
- Hiraiwa Y, Morinaka A, Fukushima T, Kudo T. Metallo-β-lactamase inhibitory activity of 3-alkyloxy and 3-amino phthalic acid derivatives and their combination effect with carbapenem. Bioorg Med Chem. 2013;21(18):5841–5850.
- Hiraiwa Y, Saito J, Watanabe T, Yamada M, Morinaka A, Fukushima T, Kudo T. X-ray crystallographic analysis of IMP-1 metallo-β-lactamase complexed with a 3-aminophthalic acid derivative, structure-based drug design, and synthesis of 3,6-disubstituted phthalic acid derivative inhibitors. Bioorg Med Chem Lett. 2014;24(20):4891–4894.
- Li N, Xu Y, Xia Q, Bai C, Wang T, Wang L, He D, Xie N, Li L, Wang J, et al. Simplified captopril analogues as NDM-1 inhibitors. Bioorg Med Chem Lett. 2014;24(1):386–389.
- Parkova A, Lucic A, Krajnc A, Brem J, Calvopiña K, Langley GW, McDonough MA, Trapencieris P, Schofield CJ. Broad spectrum β-lactamase inhibition by a thioether substituted bicyclic boronate. ACS Infect Dis. 2020;6(6):1398–1404.
- Everett M, Sprynski N, Coelho A, Castandet J, Bayet M, Bougnon J, Lozano C, Davies DT, Leiris S, Zalacain M, et al. Discovery of a novel metallo-β-lactamase inhibitor that potentiates meropenem activity against carbapenem-resistant Enterobacteriaceae. Antimicrob Agents Chemother. 2018;62(5):e00074-18.
- Zalacain M, Lozano C, Llanos A, Sprynski N, Valmont T, De Piano C, Davies D, Leiris S, Sable C, Ledoux A, et al. Novel specific metallo-β-lactamase inhibitor ANT2681 restores meropenem activity to clinically effective levels against NDM-positive Enterobacterales. Antimicrob Agents Chemother. 2021;65(6):e00203-21.
- González MM, Kosmopoulou M, Mojica MF, Castillo V, Hinchliffe P, Pettinati I, Brem J, Schofield CJ, Mahler G, Bonomo RA, et al. Bisthiazolidines: a substrate-mimicking scaffold as an inhibitor of the NDM-1 carbapenemase. ACS Infect Dis. 2015;1(11):544–554.
- Hinchliffe P, González MM, Mojica MF, González JM, Castillo V, Saiz C, Kosmopoulou M, Tooke CL, Llarrull LI, Mahler G, et al. Cross-class metallo-β-lactamase inhibition by bisthiazolidines reveals multiple binding modes. Proc Natl Acad Sci U S A. 2016;113(26):E3745–3754.
- Rossi MA, Martinez V, Hinchliffe P, Mojica MF, Castillo V, Moreno DM, Smith R, Spellberg B, Drusano GL, Banchio C, et al. 2-Mercaptomethyl-thiazolidines use conserved aromatic-S interactions to achieve broad-range inhibition of metallo-β-lactamases. Chem Sci. 2021;12(8):2898–2908.
- Yang KW, Feng L, Yang SK, Aitha M, LaCuran AE, Oelschlaeger P, Crowder MW. New β-phospholactam as a carbapenem transition state analog: synthesis of a broad-spectrum inhibitor of metallo-β-lactamases. Bioorg Med Chem Lett. 2013;23(21):5855–5859.
- Lassaux P, Hamel M, Gulea M, Delbrück H, Mercuri PS, Horsfall L, Dehareng D, Kupper M, Frère JM, Hoffmann K, et al. Mercaptophosphonate compounds as broad-spectrum inhibitors of the metallo-β-lactamases. J Med Chem. 2010;53(13):4862–4876.
- Liu S, Zhou Y, Niu X, Wang T, Li J, Liu Z, Wang J, Tang S, Wang Y, Deng X. Magnolol restores the activity of meropenem against NDM-1-producing escherichia coli by inhibiting the activity of metallo-β-lactamase. Cell Death Discov. 2018;4:28.
- Guo Y, Yang Y, Xu X, Li L, Zhou Y, Jia G, Wei L, Yu Q, Wang J. Metallo-β-lactamases inhibitor fisetin attenuates meropenem resistance in NDM-1-producing Escherichia coli. Eur J Med Chem. 2022;231:114108.
- Romero E, Oueslati S, Benchekroun M, D'Hollander ACA, Ventre S, Vijayakumar K, Minard C, Exilie C, Tlili L, Retailleau P, et al. Azetidinimines as a novel series of non-covalent broad-spectrum inhibitors of β-lactamases with submicromolar activities against carbapenemases KPC-2 (class A), NDM-1 (class B) and OXA-48 (class D). Eur J Med Chem. 2021;219:113418.
- Naas T, Oueslati S, Bonnin RA, Dabos ML, Zavala A, Dortet L, Retailleau P, Iorga BI. Beta-lactamase database (BLDB) - structure and function. J Enzyme Inhib Med Chem. 2017;32(1):917–919.
- Piccirilli A, Segatore B, Brisdelli F, Amicosante G, Perilli M. Potent inhibitory activity of taniborbactam towards NDM-1 and NDM-1(Q119X) mutants, and in vitro activity of cefepime/taniborbactam against mbls producing enterobacterales. Int J Antimicrob Agents. 2021;57(1):106228.
- Wachino J, Yamaguchi Y, Mori S, Kurosaki H, Arakawa Y, Shibayama K. Structural insights into the subclass B3 metallo-β-lactamase SMB-1 and the mode of inhibition by the common metallo-β-lactamase inhibitor mercaptoacetate. Antimicrob Agents Chemother. 2013;57(1):101–109.
- Lomovskaya O, Tsivkovski R, Totrov M, Dressel D, Castanheira M, Dudley M. New boronate drugs and evolving NDM-mediated β-lactam resistance. Antimicrob Agents Chemother. 2023;67(9):e0057923.
- Qin Y, Duan X, Peng Y, Rui Y. Rapid detection of a novel B1-β-lactamase gene, blaAFM-1 using a loop-mediated isothermal amplification (LAMP) assay. Ann Clin Microbiol Antimicrob. 2021;20(1):80.
- Teufel F, Almagro Armenteros JJ, Johansen AR, Gíslason MH, Pihl SI, Tsirigos KD, Winther O, Brunak S, von Heijne G, Nielsen H. SignalP 6.0 predicts all five types of signal peptides using protein language models. Nat Biotechnol. 2022;40(7):1023–1025.