ABSTRACT
Introduction: Studies in the 1970s and 1980s signaled concern that repeated influenza vaccination could affect vaccine protection. The antigenic distance hypothesis provided a theoretical framework to explain variability in repeat vaccination effects based on antigenic similarity between successive vaccine components and the epidemic strain.
Areas covered: A meta-analysis of vaccine effectiveness studies from 2010–11 through 2014–15 shows substantial heterogeneity in repeat vaccination effects within and between seasons and subtypes. When negative effects were observed, they were most pronounced for H3N2, especially in 2014–15 when vaccine components were unchanged and antigenically distinct from the epidemic strain. Studies of repeated vaccination across multiple seasons suggest that vaccine effectiveness may be influenced by more than one prior season. In immunogenicity studies, repeated vaccination blunts the hemagglutinin antibody response, particularly for H3N2.
Expert commentary: Substantial heterogeneity in repeated vaccination effects is not surprising given the variation in study populations and seasons, and the variable effects of antigenic distance and immunological landscape in different age groups and populations. Caution is required in the interpretation of pooled results across multiple seasons, since this can mask important variation in repeat vaccination effects between seasons. Multi-season clinical studies are needed to understand repeat vaccination effects and guide recommendations.
1. Introduction
In 1960, the US Surgeon General issued a report recommending routine annual vaccination for older adults and others at high risk for influenza complications [Citation1]. This recommendation was based on the assumption that vaccination would reduce the risk of death, and the recognition that seasonal epidemics are unpredictable from year to year [Citation2]. The policy of annual vaccination was adopted worldwide and continues to this day. In many countries, annual vaccination is still recommended only for high-risk individuals, but in North America the age criteria for annual vaccination has been incrementally expanded over the past two decades. In 2000, Ontario recommended universal annual influenza vaccination for all residents ≥6 months of age, and in 2010 the US Advisory Committee on Immunization Practices similarly recommended universal annual vaccination for all adults and children.
Annual vaccination has been justified on the basis of influenza virus antigenic drift requiring periodic vaccine updates, and by the gradual decline in vaccine-induced antibody over time. In the US, life expectancy at birth is approximately 75 years for men and 80 years for women, and a child born in 2017 can therefore expect to receive 70–80 annual influenza vaccinations based on current recommendations. Despite this, the effects of repeated annual vaccination on individual long-term protection, population immunity, and virus evolution remain largely unknown.
Signals of concern regarding potential negative effects of repeated vaccination were first raised four decades ago, and subsequent studies yielded inconclusive results. There was renewed interest in this topic after the test-negative design was first used to estimate vaccine effectiveness for preventing laboratory-confirmed influenza during the 2004–2005 season [Citation3]. After the 2009 pandemic, investigators in Canada and the US separately reported the effects of repeated vaccination during the 2010–2011 season [Citation4,Citation5]. Since then, multiple studies have assessed the effect of repeated vaccination. In this review, we describe the historical context for repeated vaccination effects, report original results of a meta-analysis based on recent studies, discuss recent immunogenicity studies and potential immunologic mechanisms, and propose a research agenda to address knowledge gaps.
2. Historical context
2.1. British boarding school outbreaks
The earliest reports regarding potential negative effects of repeated annual vaccination were published in a series of three papers describing influenza outbreaks at a British boarding school from 1972 to 1976 when only A/H3N2 and type B viruses were circulating. The initial publication described a 3-year randomized clinical trial that began in October 1970 [Citation6]. Participating youth, 11–19 years old, were randomly assigned to receive a subcutaneous dose of whole virus inactivated vaccine containing either influenza A or B. For each of the subsequent two seasons, previously enrolled boys were revaccinated with the same A/Hong Kong/1/68 vaccine and new entrants were randomized. An influenza H3N2 outbreak caused by A/England/42/72 occurred in December 1972, and the authors reported no significant difference in the attack rate for boys who had received one, two, or three doses of vaccine. However, the attack rates were not reported for each group.
A subsequent 1974 outbreak of A/Port Chalmers/1/73 demonstrated that vaccination in an earlier season can indirectly affect the risk of infection in a future season [Citation7]. The H3N2 attack rate in 1974 was significantly higher in boys who had previously received the A/Hong Kong vaccine in 1970–1972 compared with those who had not. The authors attributed this paradoxical finding of increased risk to the fact that ‘the A/Hong Kong vaccine, by reducing the proportion infected during the A/England outbreak, actually increased the proportion susceptible to attack during the subsequent outbreak of A/Port Chalmers’ (i.e. infection block hypothesis). However, even among boys uninfected in the 1972 outbreak, the attack rate in 1974 was still 50% higher in those who had previously received the A/Hong Kong vaccine (27/45, 60%) vs. the influenza B vaccine (11/26, 40%) (p = .15).
The third boarding school outbreak occurred in the spring of 1976 [Citation8]. The 1976 outbreak was caused by a new H3N2 antigenic cluster: A/Victoria/3/75. The investigators compared attack rates in 1976 for boys who had received the current A/Port Chalmers vaccine with no prior vaccination, those previously vaccinated, and unvaccinated boys. This analysis was restricted to 375 boys who attended school during all three outbreaks and was based on receipt of prior strains rather than number of vaccine doses. Accordingly, the total number of doses received in the ‘repeat vaccination’ group may have ranged from 3 to 5 ().
Table 1. Influenza A vaccines administered by season and year of matriculation at Christ’s Hospital Boarding School. Influenza A (H3N2) outbreaks occurred in December 1972, spring 1974, and spring 1976.
The attack rate among boys who received the A/Port Chalmers vaccine without prior vaccine doses was about 30% lower compared to boys who were completely unvaccinated (). Conversely, the attack rate was nearly 50% higher in those who had received all three influenza A vaccines: A/Port Chalmers + A/England + A/Hong Kong compared to the completely unvaccinated boys. The same patterns were observed among boys regardless of prior infection history during the first two outbreaks, suggesting that the infection block hypothesis does not explain all of the differences observed. However, the sample size was small in each vaccine group, and there were no statistically significant differences in attack rates across the three vaccine groups.
Figure 1. Influenza A attack rates and vaccination status at a British boarding school during the 1976 outbreak. The analysis was restricted to students attending school during all three outbreaks. We estimated the attack rates for boys not previously infected (light gray bars) by recalculating the denominators after excluding ‘Cases 1972: A/England’ and ‘Cases 1974: A/Port Chalmers’ as shown in the original publication. The A/Port Chalmers/1/73 vaccine (‘A/PC’) was co-administered with A/England/42/72 for the 1974–75 season, and with A/Scotland/840/74 for the 1975–76 season. Data for this figure were derived from of reference [Citation8] and reprinted from The Lancet, Vol 313/Issue 8106, T.W. Hoskins, Joan R. Davies, A.J. Smith, Christine L. Miller, Audrey Allchin, Assessment of inactivated influenza-a vaccine after three outbreaks of influenza a at christ’s hospital/p33-35, 1979, with permission from Elsevier.
![Figure 1. Influenza A attack rates and vaccination status at a British boarding school during the 1976 outbreak. The analysis was restricted to students attending school during all three outbreaks. We estimated the attack rates for boys not previously infected (light gray bars) by recalculating the denominators after excluding ‘Cases 1972: A/England’ and ‘Cases 1974: A/Port Chalmers’ as shown in the original publication. The A/Port Chalmers/1/73 vaccine (‘A/PC’) was co-administered with A/England/42/72 for the 1974–75 season, and with A/Scotland/840/74 for the 1975–76 season. Data for this figure were derived from Figure 2 of reference [Citation8] and reprinted from The Lancet, Vol 313/Issue 8106, T.W. Hoskins, Joan R. Davies, A.J. Smith, Christine L. Miller, Audrey Allchin, Assessment of inactivated influenza-a vaccine after three outbreaks of influenza a at christ’s hospital/p33-35, 1979, with permission from Elsevier.](/cms/asset/7cb8e8c5-4c18-4d17-a2ae-0afc0ce35e25/ierv_a_1334554_f0001_b.gif)
Figure 2. Allocation into placebo and intervention arms for five year clinical trial of repeated vaccination (from reference [Citation9]); TIV = trivalent inactivated whole virus vaccine.
![Figure 2. Allocation into placebo and intervention arms for five year clinical trial of repeated vaccination (from reference [Citation9]); TIV = trivalent inactivated whole virus vaccine.](/cms/asset/16bee036-82a1-45b1-954e-48e182270537/ierv_a_1334554_f0002_b.gif)
The cumulative attack rate across all three outbreaks was similar among boys who were unvaccinated during the entire period (51%) and boys who were appropriately vaccinated before each outbreak (41%). These findings led the authors to question the net benefit of repeated annual vaccination: ‘These observations on three outbreaks of influenza A in a school suggest that annual revaccination with inactivated influenza A vaccine confers no long term advantage. The practice of offering annual revaccination to adults seems open to question.’
2.2. Repeated vaccination randomized trial
The concern that repeated annual vaccination might reduce vaccine efficacy led the US National Institutes of Health to fund a 5-year randomized clinical trial. The trial was conducted from 1983 to 1988 and final results published in 1997 [Citation9]. Healthy 30–60 year-old adults were recruited to receive either licensed, trivalent inactivated whole virus vaccine (TIV, Connaught Laboratories) or placebo. Some, but not all, study participants were randomized. New study participants were randomly assigned to receive TIV or placebo only if they had not received influenza vaccine in the past 3 years (first vac group). Those who had been vaccinated in the past three years were assigned to the multiple vaccination (multivac) group and TIV was administered each fall for the duration of the study. As a result, the multivac group included both randomized and non-randomized individuals. Each fall, participants who had previously received placebo were re-randomized to receive vaccine (first vac group) or placebo (). Serum samples were collected pre- and post-vaccination and after each season to assess hemagglutination inhibition (HI) antibody response to vaccine and epidemic strains. There was no active illness surveillance, but participants were encouraged to report respiratory illness episodes; nasal washes were obtained for virus isolation in cell culture.
The study period included two predominant H1N1 seasons, two H3N2 seasons, and three type B seasons. Overall, the influenza infection rate (defined by seroconversion and/or virus isolation) was significantly lower for each influenza vaccine group (first vac or multivac) compared with the placebo group in 10 of 14 comparisons. In two of the remaining four comparisons, there was a lower infection rate in the vaccine group that was not statistically significant (p = .07). These analyses included both randomized and non-randomized participants. In a series of logistic regression models restricted to randomized participants, influenza infection (virus isolation or seroconversion) was not associated with the number of sequential annual vaccinations. However, the majority of infections were identified based on seroconversion, which has been recognized as a source of bias since the 1940s by underestimating infections in vaccinated vs. unvaccinated individuals [Citation10,Citation11]. This ‘antibody ceiling’ effect limits the serologic response among vaccinated individuals who develop influenza infection.
In an analysis that was restricted to randomized participants and infections identified by virus isolation, the authors reported a significant association between number of vaccine doses and virus-confirmed infection during the H3N2 dominant 1987–1988 season (odds ratio = 1.48, p = .006). The incremental odds of virus detection with increasing doses were not linear, and the virus-confirmed attack rate in 1987–1988 among people who had received six vaccine doses was 2.7-fold higher compared to those who received placebo (p = .07, chi-squared) (). In 1987–1988, the H3N2 vaccine strain was updated but antigenically similar to the prior season strain. Both the updated and previous H3N2 vaccine strains were mismatched to the 1987–1988 epidemic strain. During the same season there was a significant reduction in postvaccination geometric mean titer (GMT) against the epidemic strain with increasing number of sequential vaccinations (p < .001). In contrast to 1987–1988, there was no association between number of vaccine doses and virus-confirmed influenza in the 1984–1985 season when the H3N2 vaccine strain was identical to the prior season and well-matched to the epidemic strain; none of the participants had received more than three cumulative vaccines doses in 1984–1985.
Figure 3. H3N2 virus shedding in 1984–85 and 1987–88 by total number of vaccine doses received in randomized clinical trial. Adapted from of reference [Citation9] and reprinted from Vaccine, Vol 15/Issue 10, Wendy A. Keitel, Thomas R. Cate, Robert B. Couch, Linda L. Huggins, Kenneth R. Hess, Efficacy of repeated annual immunization with inactivated influenza virus vaccines over a five year period, p 1114–22, 1997, with permission from Elsevier.
![Figure 3. H3N2 virus shedding in 1984–85 and 1987–88 by total number of vaccine doses received in randomized clinical trial. Adapted from Figure 1 of reference [Citation9] and reprinted from Vaccine, Vol 15/Issue 10, Wendy A. Keitel, Thomas R. Cate, Robert B. Couch, Linda L. Huggins, Kenneth R. Hess, Efficacy of repeated annual immunization with inactivated influenza virus vaccines over a five year period, p 1114–22, 1997, with permission from Elsevier.](/cms/asset/608e5563-8d27-4f80-85ac-db86fdd8e4bd/ierv_a_1334554_f0003_b.gif)
The authors concluded that the study results ‘provide reassuring evidence that annual vaccination of adults with inactivated influenza vaccine (IIV) confers significant protection against influenza each year.’ However, the trial results should be interpreted cautiously due to several limitations. The multivac group included both randomized and non-randomized participants – an important caveat since baseline influenza risk may have been different due to more frequent prior natural infection in those who were unvaccinated at the time of study entry. The primary clinical end point included serologic criteria (seroconversion), a well-recognized source of bias. In addition, relatively few cases of virus-confirmed influenza were identified within each vaccine exposure group and season, limiting power to detect differences. The generalizability of the study is further limited because the whole virus vaccine that was licensed at that time has been replaced with split or subunit vaccine formulations.
2.3. Antigenic distance hypothesis
In 1999, a potential explanation for the variation in repeated vaccination effects was proposed by Smith and colleagues based on a computer simulation of B-cell clones with different antigen specificities [Citation12]. According to this ‘antigenic distance hypothesis’, vaccine efficacy is influenced by the antigenic similarity between the prior season vaccine strain and the epidemic strain (v1–e), and the antigenic similarity between the current and prior season vaccine strains (v1–v2). In the theoretical model, negative and positive interference was determined by two competing immune processes: v2 antigen trapping by v1 antibody, and v2 stimulation of a v1 memory response that cross-reacts with the epidemic strain (e).
The computer model considered a simplified scenario with two influenza seasons and challenge with epidemic virus in the second season. The first season vaccine had varying antigenic distance to the second season vaccine (v1–v2 distance) and to the epidemic strain (v1–e distance). The antigenic distance between the second season vaccine (v2) and the epidemic strain was held constant (v2–e = 2, or antigenically similar). This was explored for other homologous vaccine scenarios (v1 = v2) for v2–e distances ranging from one to three. The simulation examined outcomes in four groups: (1) individuals not vaccinated either season; (2) those receiving v1 only; (3) those receiving v2 only; and (4) those vaccinated in both seasons (v1 + v2).
The model demonstrated that repeat vaccination could lead to higher or lower attack rates compared to first-time vaccination depending upon conditions of v1–v2 and v1–e relatedness. A lower attack rate (positive interference) was observed in repeat vaccinees than first-time vaccinees when v1 was antigenically close to the epidemic strain. A higher attack rate (negative interference) was observed in repeat vaccinees when the antigenic distance between v1 and v2 was small and v1 was antigenically distinct from the epidemic strain. This effect was pronounced when v1 and v2 strains were identical (i.e. homologous), and attack rates were higher in both single and repeat vaccinees with increasing antigenic distance between the vaccine and epidemic strain.
The model was tested using the results from the 1972 and 1974 (but not 1976) British boarding school outbreaks and the multiseason randomized clinical trial results based on serologic and virologic outcomes with randomized and non-randomized participants [Citation9]. The model did not predict absolute vaccine efficacy, but there was a high correlation (r = 0.87) between the observed results and the predicted ratio of efficacy in repeat vs. first-time vaccinees.
3. Meta-analysis of repeated influenza vaccination and vaccine effectiveness
For this review, we undertook a meta-analysis of studies conducted after the 2009 pandemic to evaluate the effects of prior season vaccination on current season vaccine effectiveness (VE) by type/subtype. We restricted this analysis to studies that considered the effect of current and/or prior season vaccination using a four-level exposure variable: vaccinated current season only (not prior), vaccinated prior season only (not current), vaccinated both seasons, or unvaccinated in both seasons (referent group).
3.1. Search strategy and selection criteria
We searched for English language studies published from 1 January 2009 to 3 August 2016 that reported vaccine efficacy or effectiveness against RT-PCR-confirmed influenza illness. We searched Medline, EMBASE, clinicaltrials.gov, and other databases using combinations of search terms that included ‘influenza’, ‘vaccines’, ‘treatment outcomes’, ‘efficacy’, and ‘effectiveness’. The specific search terms are shown in Supplementary Figure 1. We included studies conducted in the outpatient, inpatient, or community setting, but inpatient studies were summarized separately since VE for preventing influenza hospitalization may not be comparable to VE in the outpatient setting. We included observational studies using the test-negative design, case-control studies, cohort studies, and randomized controlled trials. To reduce bias, we restricted the analysis to studies that applied standard symptom or syndrome criteria for enrollment. The specific symptom eligibility criteria were variable, but studies were excluded if they used a convenience sample of clinical diagnostic tests rather than predefined screening criteria. Studies were included if they reported VE against H3N2, H1N1pdm09, or type B for a single season and assessed vaccination status in the current and immediate past season. We excluded cost-effectiveness studies, review articles, and studies using nonspecific clinical or serologic end points. Studies reporting current season VE for either pre-2009 seasonal vaccines or monovalent H1N1pdm09 vaccine were excluded to ensure maximum relevance for current seasonal vaccination. The end points for the meta-analysis were vaccine effectiveness against RT-PCR-confirmed influenza illness by type or subtype.
We included one publication after 3 August 2016 because results were available to us before online publication on 4 October 2016 [Citation13]. The specific search terms are shown in the online appendix, and the protocol is available from the authors on request.
3.2. Data analysis
Two reviewers abstracted data for eligibility criteria, study characteristics, and VE estimates using a structured electronic data collection form. Discrepancies were reviewed and corrected by one author with approval of all reviewers. Pooled estimates were generated using separate simple random-effects models [Citation14] to calculate the weighted pooled log odds ratio and corresponding VE and 95% confidence interval (CI) for each level of vaccine exposure compared to unvaccinated in both seasons. Vaccination in the prior season only was interpreted as residual protection. Weights for each model were calculated using inverse variances that incorporated the between-study variance [Citation14,Citation15]. Heterogeneity among studies was assessed using the χ2-based Q test and I2 statistic [Citation15].
The primary analysis included studies for all seasons with no age restriction beyond childhood. Pooled estimates were also generated when there were ≥3 individual estimates for each season. All analyses were performed using SAS version 9.4.
3.3. Results
We identified 4636 unduplicated publications and selected 183 for full-text review for eligibility (). Eighteen studies met the eligibility criteria [Citation4,Citation13,Citation16–Citation31]. Of these, one study was restricted to hospitalized patients and was therefore excluded from the meta-analysis [Citation31]. All studies originated in the Northern Hemisphere, with the majority from North America (). Most (82%) studies enrolled outpatients with medically attended acute respiratory illness, 1 enrolled both outpatients and inpatients, and 2 enrolled participants from the community in a household cohort study. The 17 publications included 31 sets (current only, prior only, and both seasons) of VE estimates that included unrestricted age groups. Characteristics of included studies by season, subtype, and age group are shown in Supplementary Table 1.
Table 2. Characteristics of 17 published studies that met eligibility criteria for assessment of current and prior season vaccine effectiveness.
Figure 4. Flow chart for screening and selection of articles for inclusion in meta-analysis of repeated vaccination and vaccine effectiveness.
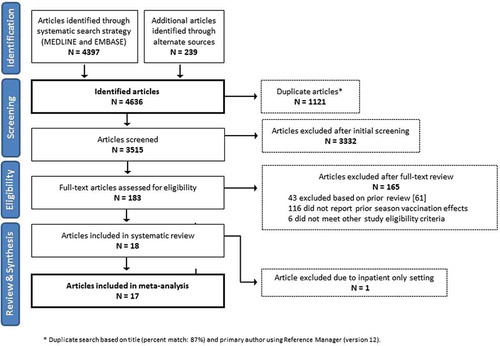
VE estimates were consistently lowest for H3N2 across all vaccine groups. There was substantial heterogeneity in repeat vaccination effects within and between seasons and subtypes. When negative effects of prior vaccination were observed, they were most pronounced for H3N2, notably driven by two studies in the 2014–2015 season. The pooled estimates should be interpreted with caution since the antigenic relatedness of vaccine and virus strains varies by season and subtype.
3.3.1. H3N2
H3N2 VE estimates by current and prior vaccination status were available from seven studies and three seasons, including 2011–2012 (n = 2), 2012–2013 (n = 2), and 2014–2015 (n = 3). The vaccine strain was unchanged in two of these seasons (2011–2012 and 2014–2015). There was evidence of antigenic mismatch with the vaccine strain in two seasons. In 2012–2013, circulating viruses were well matched to the WHO cell-propagated reference strain, but antigenic mismatch occurred from mutations in high-growth reassortant viruses used for vaccine manufacturing [Citation25]. In 2014–2015, the vaccine reference strain was poorly matched to the dominant circulating strain from a new antigenic cluster [Citation32]. Studies were contributed from Canada (n = 3), the US (n = 3), and European multi-country (n = 1).
In general, H3N2 estimates were highest for vaccination in the current season only. Pooled estimates for H3N2 were 39%, 17%, and 9%, respectively, for current season only, current and prior season, and prior season only vaccination (). The pooled VE was statistically significant (i.e. greater than zero) for current season vaccination only; pooled VE was not significant for repeated vaccination. However, heterogeneity was high for vaccination in current season only (I2 = 73%, p = .003) and in both prior and current season (I2 = 86%, p < .0001) suggesting important underlying variability for which pooled analysis may not be appropriate. Conversely, the observation of no residual protection from prior season vaccination was more consistent, with lower heterogeneity (I2 = 48%, p = .1). Of note, two of three studies reported pronounced difference between repeated versus current season only vaccination during the 2014–2015 season [Citation28,Citation30], and this difference was statistically significant in one study [Citation28]. Vaccine strains were unchanged and there was well-recognized mismatch between vaccine and epidemic strains, a pattern consistent with the antigenic distance hypothesis. However, this difference was not observed in the 2014–2015 U.S. Flu VE Network Study which had more influenza cases with repeated vaccination and single season vaccination than the other two studies combined [Citation13].
3.3.2. H1N1pdm09
H1N1pdm09 VE estimates by current and prior season vaccination status were available from ten studies and four seasons, including 2010–2011 (n = 5), 2011–2012 (n = 1), 2013–2014 (n = 3), and 2014–2015 (n = 1). Studies were contributed from more diverse countries than for H3N2, including Canada (n = 3), Spain (n = 2), the US (n = 2), Finland (n = 1), the UK (n = 1), and European multi-country (n = 1). Recommended vaccine strains were identical across all seasons () and considered antigenically similar to circulating H1N1pdm09 viruses across this period despite the emergence of a new genetic variant (clade 6B) in 2013 [Citation27]. It is noteworthy that studies included a mix of countries that had used mostly AS03-adjuvanted monovalent H1N1pdm09 vaccine in response to the 2009 pandemic (e.g. Canada, Finland, Spain, or UK), or exclusively non-adjuvanted product (e.g. US), or a combination (e.g. multi-country European study). The impact of adjuvanted monovalent vaccine use on the magnitude or duration of repeated vaccination effects is unknown. Prior to 2013–2014, H1N1pdm09 estimates were contributed solely from outside the US and were generally higher for repeat vaccinees than current season only vaccine recipients, with suggestion also of substantial residual protection from prior season vaccination.
Table 3. WHO vaccine reference strains and corresponding egg-adapted high-growth reassortant viruses for vaccine production from 2009–2010 through 2014–2015.
Pooled estimates in 2010–2011 for current season only, current and prior season, and prior season only vaccination were 54%, 69%, and 61%, respectively (). These were each statistically significant relative to no vaccination. Conversely, studies from Canada and the US in 2013–2014 showed similar or slightly lower VE among persons repeatedly vaccinated vs. those vaccinated in the current season only; VE was lower but still significant for those vaccinated only in the prior (2012–2013) season. In the overall pooled analysis across all seasons, heterogeneity was high for repeated vaccination (I2 = 69%, p = .002), with more consistency in findings for vaccination in the current season only (I2 = 0%, p = .9) or prior season only (I2 = 40%, p = .1).
3.3.3. Type B
Influenza B VE estimates by current and prior vaccination history were available for both lineages (combined) from five studies and four seasons including 2011–2012 (n = 1), 2012–2013 (n = 1), 2013–2014 (n = 1), and 2014–2015 (n = 2). Lineage-specific estimates for B/Yamagata were available from six studies and four seasons including 2011–2012 (n = 1), 2012–2013 (n = 2), 2013–2014 (n = 1), and 2014–2015 (n = 2). For B/Victoria, there were only three studies involving two seasons: 2011–2012 (n = 1) and 2012–2013 (n = 2). Influenza B studies were contributed from Canada (n = 10), the US (n = 3), and European multi-country (n = 1).
Quadrivalent inactivated vaccines containing both B lineages were first licensed in the US in 2013, but most vaccines administered during the study period were trivalent and contained only one B lineage. The same B/Victoria lineage strain (Brisbane/60/2008) was included as trivalent vaccine component from 2009–2010 to 2011–2012, and a B/Yamagata lineage was included from 2012–2013 through 2014–2015 (). There was circulation of both lineages in 2011–2012 and 2012–2013, whereas the B/Yamagata lineage dominated in 2013–2014 and 2014–2015 with clade variation relative to vaccine in both seasons.
VE estimates for B overall were similar or slightly higher for repeat vaccination compared to current season only during 2011–2012 and 2012–2013 seasons of mixed lineage circulation, but were lower during 2013–2014 and 2014–2015 seasons of lineage-matched (B/Yamagata) but clade-mismatched circulation (). Consequently, pooling across the four seasons resulted in overall minimal differences between the two vaccine groups for influenza B. Pooled estimates for type B were 61%, 55%, and 25%, respectively, for current season only, current and prior season, and prior season only vaccination. The lineage-level analysis demonstrated a similar pattern for B/Yamagata in 2013–2014 and 2014–2015, with lower VE estimates in repeat vaccinees. With so few studies and seasons, a pattern for B/Victoria is difficult to discern.
Figure 7. Vaccine effectiveness for type B (all), B/Yamagata, and B/Victoria studies without age restriction. For each VE estimate, the comparison group included individuals who were unvaccinated in both the current and prior season.
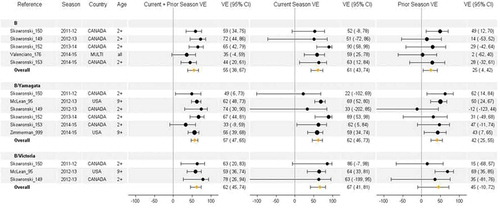
In most studies and in pooled analyses, both groups of current vaccine recipients (with or without prior season vaccination) had higher VE than prior season only recipients although residual protection from prior season vaccination was evident and statistically significant in pooled analyses for influenza B and B/Yamagata. Low to moderate heterogeneity was observed for type B overall (I2 = 0–52%, p ≥ .1), B/Yamagata (I2 = 7–20%, p ≥ .4), and B/Victoria (I2 = 0–56%, p ≥ .2).
4. VE studies of repeated vaccination in multiple prior seasons
Few studies have evaluated the impact of repeated vaccination on clinical efficacy or effectiveness since the randomized trial was conducted in the 1980s [Citation9]. A large observational study on repeat vaccination effects was conducted over eight seasons in Marshfield, Wisconsin [Citation33]. In this rural community, the Marshfield Clinic Research Institute has conducted annual studies of influenza vaccine effectiveness using the test-negative design since 2004–2005 [Citation18,Citation23,Citation34–Citation36]. Each season, eligible individuals are enrolled and swabbed when seeking outpatient care for acute respiratory illness. Influenza vaccination dates over a 5-year period were determined using a validated, web-based electronic immunization registry [Citation37].
To analyze prior vaccination effects over multiple seasons, multivariate models evaluated the association between RT-PCR-confirmed influenza (outcome) and exposure variables for current season vaccination and 5-year vaccination history. The latter was classified as frequent vaccinee (at least four of five past seasons), infrequent vaccinee (1–3 vaccines), or nonvaccinee (unvaccinated in prior five seasons). The comparison group included individuals who were unvaccinated in the current season and all of the preceding five seasons.
The analysis included 5800 study participants who were in the community cohort for at least five continuous years. Current season VE against H3N2 was significantly higher (65%; 95% CI 36–80) among nonvaccinees (i.e. those vaccinated in current season but not any of the prior 5 years) compared to frequent vaccinees (24%; 95% CI 3–41). A secondary analysis stratified by H3N2 season (2007–2008 and 2012–2013) yielded results similar to the overall analysis, but interpretation was limited by the smaller sample size and wide CIs.
For influenza B, VE was significantly higher for vaccinated individuals who were unvaccinated during the prior five seasons (VE 75%; 95% CI 50–87) compared to those who were frequent vaccinees (VE 48%; 95% CI 29–62). There was evidence of residual protection against type B among frequent and infrequent vaccinees who did not receive the current season vaccine.
An additional study assessed the impact of prior vaccination in one and/or two seasons on vaccine effectiveness against H3N2 during the 2014–2015 season in Canada [Citation28]. In this study, patients were enrolled by sentinel physicians, and current and prior vaccinations were determined by physician records and/or self-report. In the analysis that considered only current and prior season vaccination, there was a borderline significant elevated risk of influenza (negative VE) among individuals repeatedly vaccinated (2013–2014 and 2014–2015) compared to those unvaccinated in both seasons (VE −32%; 95% CI −75 to 0). In the analysis that considered vaccine receipt in two prior seasons, there was a significant increased risk of influenza among those who were vaccinated for three consecutive seasons (including the 2014–2015 season) compared to those who were unvaccinated during all three seasons (VE −54%; 95% CI −108 to −14).
A study using the test-negative design evaluated VE in Navarra, Spain, during the 2014–2015 season with vaccine exposure assessed over a three-season period: 2012–2013 through 2014–2015 [Citation38]. This study found no significant protection against H3N2 in any vaccine group, but protection against influenza B was high (>80%) among current season only vaccinees and among patients who were vaccinated in two prior seasons only (not current season). Conversely, VE against influenza B was lower (43%) but still significant among patients who were vaccinated all three seasons. Generalizability of these results is limited because the study pooled inpatient and outpatient cases of influenza, and vaccine formulations were systematically different in the prior seasons (split vaccine only) versus the current season (subunit vaccine only).
Finally, three Canadian test-negative studies conducted in 2011–2012 through 2013–2014 reported VE against H1N1pdm09 for current and/or prior season TIV receipt, with or without 2009 monovalent pandemic vaccine (mostly containing AS03 adjuvant) [Citation25–Citation27]. During the first two seasons, there was nonsignificant residual protection (VE ~50%) against H1N1pdm09 from prior 2009 monovalent vaccine receipt despite no subsequent TIV doses. By 2013–2014 this residual monovalent vaccine protection was essentially zero (VE ~1%). Across the study period, repeat vaccine recipients were better protected than the consistently unvaccinated or those who received only the 2009 monovalent vaccine.
5. Immunogenicity studies of repeated vaccination
Several studies since the 2009 pandemic have assessed the impact of repeated vaccination on adaptive immune response. Although earlier studies have shown that prior vaccinees are less likely to seroconvert after vaccine receipt than first-time vaccinees, the more recent studies demonstrate that repeated vaccination can blunt the antibody response to hemagglutinin even after adjusting for prevaccination titer.
5.1. Michigan clinical trial
A study at the University of Michigan demonstrated that blunting of the serologic response to IIV can persist up to 18 months after repeat vaccination. Investigators collected serial serum samples from adults who participated in a randomized trial comparing IIV and live attenuated influenza vaccine (LAIV) efficacy in 2004–2005 and 2005–2006 [Citation39]. Subjects were randomized to receive LAIV, IIV, or placebo in 2004–2005, and each group received the same intervention during the second season while new recruits were randomized. In the second season, nearly all IIV recipients had postvaccination HI titers ≥32 for both H3N2 and B/Yamagata, and the estimated time to a 50% (2-fold) decrease in titer was 410 days for H3N2 and 424 days for B/Yamagata. The GMTs remained significantly lower (p < .001) in the repeat IIV group compared to the single IIV group at all four time points up to 18-month postvaccination.
5.2. Michigan household cohort study
Another study at the University of Michigan found blunting of the H3N2 vaccine response in a cohort study with 321 households (1426 members) during the 2012–2013 season [Citation21]. Subjects with any vaccine exposure had higher titers than those who were unvaccinated in both 2012–2013 and the prior season. The HI titer against H3N2 was significantly higher (p = .03) in single vaccinees (current season only) compared to repeat vaccinees. There were no significant differences for the other antigens or for neuraminidase titers. A similar household cohort study in 2013–2014 found no difference in preseason HI titers against H1N1pdm09 among persons vaccinated in two consecutive seasons (2012–2013 and 2013–2014) and those vaccinated only in the current (2013–2014) season [Citation22]. Neuraminidase inhibition titers were also similar in these two groups.
5.3. Serologic response to repeated vaccination in health-care workers
In 2010–2011, blunting of the serologic response to the H3N2 vaccine strain was demonstrated in a cohort of 816 health-care workers [Citation40]. The investigators aimed to determine if there was a dose–response relationship between the number of prior influenza vaccinations (up to four) and the HI response to A/Perth/16/2009, the WHO-recommended H3N2 component of the 2010–2011 vaccine.
Health-care workers who had received one or more doses of IIV in the past 4 years all had similar adjusted prevaccination titers, but the adjusted geometric mean fold rise (MFR) after vaccination was inversely associated with the number of prior vaccinations. The MFR was 6.2 (95% CI 3.4–11.3) among those with no prior vaccinations, 4.3 (95% CI 3.3–5.5) among those with one prior vaccination, and 2.3 (95% CI 2.1–2.6) among those with four prior vaccinations. The findings were similar when the analysis was stratified into groups with low (<40) and high (≥40) baseline titers against H3N2. Post-season H3N2 titers were highest in participants who received only one vaccination in the prior four seasons. There was a consistent inverse association between vaccine serologic response to A/Perth/16/2009 and the number of prior vaccine doses containing A/Wisconsin/67/2005 used in 2006–2007 and 2007–2008 but not the more recent A/Brisbane/10/2007 vaccine strain used in 2008–2009 and 2009–2010. The authors proposed differential responses to historic vaccines to explain this apparent paradox.
5.4. Serologic response in young and older adults with repeated vaccination
An open-label clinical trial over four seasons suggested that the declining serologic response to influenza vaccination in older adults may be partially attributed to repeat vaccination effects rather than immunosenescence [Citation41]. Each year, healthy young adults (20–40 years) who had never received influenza vaccine and healthy older adults (≥65 years) who had not been vaccinated in the prior 2 years were recruited. All new and returning participants received licensed IIV in the fall, and paired serum samples were collected. For all seasons and subtypes combined, the initial prevaccination GMTs were nearly identical in the young and old cohorts, but postvaccination GMT was nearly twice as high in the younger vs. older adults. This age effect diminished with increasing number of repeated vaccinations. After an individual had received two or more vaccine doses within the study, the age effect disappeared, and this was observed for all three vaccine subtypes.
6. Possible immune mechanisms for repeated vaccination effects
All vaccines are intended to trigger an immunological response, but influenza is the only vaccine for which components must be updated routinely due to virus antigenic evolution. It is the only vaccine given every year. This has poorly understood effects that may vary depending on whether the vaccine strain has been changed compared to the prior season(s), changes in the genetic and antigenic characteristics of circulating viruses, and the unique ‘antibody landscape’ determined by cumulative lifetime experience with influenza infection or vaccination [Citation42]. As noted in the 1974 British boarding school outbreak [Citation7], natural infection appears to convey broader and more durable immunity relative to vaccination [Citation43]. As proposed by Hoskins et al., vaccine-induced protection in a given season may block the greater infection-induced immunity and thereby contribute to higher risk in a subsequent season (infection block hypothesis). However, the infection block hypothesis is unlikely to explain all of the increased risk among repeat vaccinees in the British boarding school outbreaks.
The antigenic distance hypothesis has been the major theoretical framework for understanding variability in repeat influenza vaccination effects. It remains a useful tool for interpreting repeated vaccination effects, but it is likely an incomplete conceptualization. The predictions of this hypothesis are based entirely on the HI antibody response, but the adaptive immune response to other virus components (i.e. HA2 or neuraminidase) is likely to contribute. It was intended to predict relative but not absolute vaccine effectiveness, and it incorporates only a single prior season. It therefore does not consider more distant virus or vaccine exposures that may also shape the immune response to influenza vaccine. The potential for increased risk among repeatedly vaccinated individuals and the heterosubtypic effects of trivalent or quadrivalent vaccine were also not considered by the antigenic distance hypothesis [Citation12].
Other immunological mechanisms that extend or complement the antigenic distance hypothesis should be considered. It has long been recognized that prominent recall of memory responses to distant priming (imprinting) antigens can shape hierarchical antibody responses to influenza (a.k.a. original antigenic sin). Annual vaccination, as opposed to less frequent infection exposure, may hasten such ‘sinful’ antibody refocusing toward prior versus evolved epitopes, preferentially selecting for cross-reactive but non-neutralizing memory responses [Citation44–Citation47]. In the context of pre-existing antibody, immune complex formation and Fc-receptor activation can lead to suppression of B cell responses to subsequent influenza vaccination [Citation48]. Whether antibody-dependent mechanisms may also suppress T cell responses to influenza, as described in other virus models, is an area requiring further research [Citation49]. The preferential induction or recruitment of regulatory cells upon re-exposure has been proposed as an explanatory mechanism for original antigenic sin responses and may also contribute to antagonistic T cell responses with repeat influenza vaccination [Citation50]. There is evidence from animal studies that repeated vaccination can interfere with the development of cross-reactive immunity against other subtypes, likely mediated by reduced virus-specific CD8+ T cell response [Citation51,Citation52]. In children, annual vaccination has also been demonstrated to hamper development of virus-specific CD8+ T cell immunity [Citation53]. The implications remain unclear, but these findings raise the possibility that repeated vaccination of low-risk individuals could affect the occurrence or severity of illness caused by a new pandemic virus or a new seasonal virus antigenic cluster.
Scientific investigation into the immunologic effects of annual vaccination remains severely lacking, particularly the long-term effects of repeated vaccination over decades and the specific preconditions for negative interference in some seasons. Ultimately, the mechanisms to explain the variable and potential negative effects of repeat vaccination remain unknown, but they are likely multi-factorial and will require a coordinated research strategy and a complex systems biology perspective to address comprehensively [Citation54].
7. Methodological challenges for evaluation of repeated vaccination effects
Experimental studies can randomize participants to ensure that study arms are comparable in their risk of acquiring the disease and prognostic factors outside vaccination. This is not possible with observational studies, which have methodological challenges such as selection bias and residual confounding. For analysis of repeat vaccination effects, this translates into some specific challenges. Most individuals habitually engage in the same pattern of vaccination (or nonvaccination) every year. Only a small proportion are vaccinated for the first time in a given season, seriously limiting the statistical power for VE comparisons between first-time vaccinees and repeat vaccinees. Direct comparison of the influenza risk between first-time and repeat vaccinees has greater statistical power, but the limited number of first-time vaccinees is still a concern.
The reasons driving individuals to remain unvaccinated, choose to be vaccinated for the first time, or to be vaccinated repeatedly can be associated with their risk of disease or complications. If a substantial proportion of first-time vaccinees are individuals who had influenza infection in the previous season, the natural immunity acquired with this prior infection will decrease the risk of influenza compared to repeat vaccinees and may lead to the erroneous conclusion that vaccine protection decreases with multiple doses. First-time vaccinees are necessarily younger than repeat vaccinees and their immunological background may differ in terms of the first influenza virus encountered (imprinting), lifetime number of natural infections, and the overall influenza antibody landscape [Citation42]. The usual adjustment for age using continuous or dummy variables may not completely address this problem and residual confounding may persist.
Misclassification of vaccination status can occur from both self-report and immunization registries. The potential impact of this misclassification will be greater for studies that assess individual vaccination status over multiple seasons compared to a single season. Self-report is likely to be accurate for the current season and at least one prior season [Citation37], as well as for individuals with more habitual vaccination patterns, but may be subject to recall bias for more distant seasons and for individuals with irregular vaccinations. For first-time vaccinees and irregularly vaccinated individuals, the magnitude of misclassification from self-report is unclear. Immunization registries are incomplete and may not contain all relevant current or prior vaccination records, contributing to greater misclassification for missing vaccination when used to classify vaccination status over multiple seasons. Misclassification will be mostly non-differential between influenza cases and test-negative controls, driving any association toward the null value and underestimating differences in risk between different groups of vaccinated and unvaccinated individuals.
Epidemiological analyses of repeat immunization must consider the classical host–agent–environment triad: immunological landscapes that vary by age, birth cohort, and jurisdiction (host), vaccine strains (agent), and antigenic drift of circulating viruses (environment). Pooling VE over several years for single season vaccinees vs. repeat vaccinees assumes that vaccines with different antigenic profiles have the same immunologic effects in populations with variable immunological landscapes and different circulating viruses, a far-fetched assumption. Pooling VE within a specific influenza season controls for the effect of vaccine updates, but it does not eliminate differences in immunological landscape and characteristics of circulating strains/clades in different jurisdictions. Overall, pooling will tend to mask important variability and diminish the overall magnitude of repeat vaccination effects.
8. Expert commentary
After more than four decades since concerns were initially raised about repeat vaccination effects, the magnitude and mechanisms remain poorly understood. During this time, the target groups recommended for annual vaccination have expanded in many countries and now include nearly all citizens in the US and most parts of Canada. The multiseason randomized clinical trial during the 1980s did not provide definitive answers [Citation9], and concerns resurfaced after the 2009 pandemic when VE studies began to assess repeat vaccination effects. Similar to findings in the 1970s and 1980s, more recent observational studies from 2010–2011 through 2014–2015 show variable effects but suggest that repeat vaccination may adversely affect vaccine-induced serological response and effectiveness in some seasons, particularly for H3N2. This is generally consistent with multiple studies demonstrating that repeated vaccination can blunt the HI antibody response to vaccine strains.
Our systematic review and meta-analysis covered a relatively short time period, and there were few studies that met our inclusion criteria. Most analyses of repeated vaccination were conducted as a secondary analysis, and the precision of the estimates was low. In addition to the small number of studies and small sample sizes by vaccination group, there was heterogeneity in study design, patient population, study setting, vaccination type and ascertainment, age groups, and seasons. Not surprisingly, we found substantial heterogeneity in repeated vaccination effects. Heterogeneity of repeated vaccination effects is plausible given the variability of the immunological landscape in different age groups and populations and given variability in the relatedness between successive vaccine components and the circulating strain, by season and subtype. The antigenic distance hypothesis predicts variability even in the context of a single prior season’s antigenic exposures, but the actual immune response is undoubtedly far more complex. Pooling of VE estimates across multiple seasons can mask important differences at the individual season level, and studies conducted in only three or four seasons are inadequate to understand repeated vaccination effects that may be positive, negative, or neutral for any given season, subtype, or population subgroup.
Within the limited number of seasons assessed, the signal of concern was greatest for H3N2. The pooled effect of repeated vaccination for H3N2 was driven by the 2014–2015 season when there was a major antigenic drift in the epidemic strain and the vaccine strain was unchanged from the prior year. In that context, two of three studies identified negative interference and in one of these studies there was an increased risk in repeat vaccinees; the difference between repeat and single season vaccine groups was statistically significant. Also during the 2014–2015 season, a study in Italy found a significantly increased influenza risk (negative VE) in a population where repeated vaccination was common, but VE was not reported separately for single season vaccination and repeated vaccination [Citation55]. This study was therefore not included in the meta-analysis.
The antigenic distance model predicts a higher attack rate in repeat vs. first-time vaccinees when v1≈v2 and v1 ≠ e. Although blunting of cross-protection should be greater with homologous vaccines and greater antigenic drift, the original antigenic distance model did not assess scenarios with homologous vaccine components and a high level of mismatch comparable to 2014–2015 (v–e > 3). During the other two recent seasons (2011–2012 and 2012–2013) for which non-homologous H3N2 vaccine antigens were used in prior and current seasons, the effects of repeated vaccination were less consistent or pronounced and none showed significant variation by vaccine group.
The egg-based manufacturing process can introduce antigenically important mutations in high-growth reassortant viruses used for vaccine production [Citation25]. The long-term immune effects of repeated exposure to egg-adapted vaccine strains that do not exactly match circulating strains and of blocking natural infection in healthy individuals with a low risk of influenza complications are unknown. The public health impact of repeated immunization and the immunologic mechanisms leading to reduced protection or increased risk remain poorly understood, and a major research effort is justified given the substantial disease burden associated with influenza (especially H3N2), the large public investment in influenza vaccination programs, and the need to maintain public confidence in vaccines. Our current understanding of repeated vaccination effects is inadequate to inform vaccine policy recommendations, but future research may shed light on the optimal strategies for vaccine strain updates and/or vaccination intervals in different age cohorts and populations.
9. Five-year view
An increase in basic and applied research is needed over the next 5 years to gain greater understanding of immunologic mechanisms and clinical significance of repeated vaccination effects. The emerging field of ‘systems vaccinology’ has the potential to identify T cell, B cell, and transcriptional factors that distinguish the responses to a single vaccine and repeated vaccination [Citation56]. Most influenza vaccines are derived from egg-adapted high-growth reassortant viruses, and the contribution of egg-induced mutations to repeated vaccination effects should be elucidated. In addition, animal and computational investigations suggest that some adjuvanted vaccines may reduce or eliminate original antigenic sin effects [Citation50,Citation57,Citation58]. Additional research is needed to understand mechanisms and determine if this may be observed in a sustainable way in humans.
Large-scale, multiseason community cohort studies were conducted more than 30 years ago to understand the epidemiology of influenza [Citation59]. Since that time there have been advances in molecular diagnostic tests, changes in vaccine policies, and greater understanding of antigenic and genetic evolution of influenza viruses. There is a compelling need for new multiseason studies that will include detailed assessment of vaccine immune response (including B cell and T cell), and active surveillance for RT-PCR-confirmed influenza in children and adults who have received a single dose or repeated annual vaccine doses. The detailed genetic and antigenic characterization of circulating variants in relation to vaccine strains (both egg- and cell-passaged) will be important to document annually in order to assess whether results are consistent with the antigenic distance hypothesis, and/or to formulate additional hypotheses.
Observational studies are more feasible and cost-efficient than clinical trials, but they may not provide the robust evidence needed to validate or modify vaccine policy recommendations. Given the global impact of influenza and the expansion of annual vaccination programs, there is a need for government or private foundation funding to support a large multiseason randomized trial. Due to ethical constraints, a placebo-controlled trial would be challenging to implement in countries or jurisdictions where universal annual vaccination is recommended. The trial design should take advantage of new knowledge and lessons learned since the prior clinical trial in the 1980s. Evaluation of the adaptive immune response to single and repeated vaccination should include modern immunological concepts and assays, including anti-HA stalk and neuraminidase antibodies, antibody-dependent cellular cytotoxicity, and measures of epitope-specific T and B cell response. The study should be sufficiently powered to detect significant positive or negative interference from repeat vaccination in a single season of moderate to high severity. Given the unpredictability of influenza seasons, enrollment and randomization should be planned for five or more seasons to achieve a high probability of success.
It is possible that changes in vaccine technology, including development of a universal vaccine, could fundamentally alter influenza vaccine program options in the coming decade and reduce concerns about repeated vaccination effects [Citation60]. Even in this optimistic scenario, the knowledge gained regarding influenza immune response will be valuable, and it will be critical if inactivated egg-based vaccines continue to be used by vaccination programs in many countries.
Key issues
Observational studies conducted after the 2009 pandemic show variable effects but suggest that repeat vaccination may adversely affect vaccine-induced protection in some seasons, particularly for H3N2. Interpretation is limited by the short time frame, limited number of studies, and high heterogeneity in some VE estimates. Pooling of VE estimates across multiple seasons can mask important differences at the individual season level, and studies conducted since 2009 are inadequate to guide program or policy recommendations at this time.
Few studies have assessed the effects of repeated vaccination across multiple seasons, but these suggest that vaccine effectiveness may be influenced by vaccination patterns over at least several seasons.
Immunonogenicity studies indicate that repeated vaccination can blunt the HI antibody response against H3N2, even after adjusting for prevaccination titer.
A more detailed understanding of the immunological basis for vaccine-induced protection is needed. The antigenic distance hypothesis provides a simplified theoretical framework for understanding the immunological effects of repeated vaccination, but the model considers only two vaccine exposures.
The long term immunological effects of repeated exposure to egg-adapted vaccine strains that do not exactly match circulating strains, and of blocking natural infection in healthy individuals with a low risk of influenza complications are unknown. These questions merit further investigation, particularly since annual vaccination is recommended for healthy working age adults in much of North America.
An increase in basic and applied research is needed over the next decade. Multiseason clinical studies should include both observational (cohort) studies and a randomized clinical trial. These studies should incorporate modern immunological concepts and assays, including HA stalk and NA antibodies, antibody-dependent cellular cytotoxicity, and measures of epitope-specific T and B cell response. The findings from these studies will be important to guide future influenza vaccine policies and recommendations.
Declaration of interest
E Belongia and H Mclean received research support from MedImmune and U.S centers for Disease Control and Prevention. GD Serres received investigator- initiated grants from GSK and Pfizer, travel reimbursement to attend an ad hoc advisory board meeting of GSK and honorarium from the Ontario Nurse Association for providing expert testimony during legal challenge of enforced healthcare worker influenza vaccination. D Skowronski received funding from the Public Health Agency of Canada and the Bill & Melinda Gates foundation to convene the I-ReV (Influenza – Repeat Vaccination) Symposium held in Vancouver, British Columbia, Canada in October 2016 to support multi-disciplinary discussion of related epidemiological findings and their possible immunological mechanisms. The authors have no other relevant affiliations or financial involvement with any organization or entity with a financial interest in or financial conflict with the subject matter or materials discussed in the manuscript apart from those disclosed.
Supplementary_Material.zip
Download Zip (28.2 KB)Acknowledgements
The authors thank Lisan Kwindt (BC Centre for Disease Control) for data management support of the meta-analysis and Ursula Ellis (University of British Columbia) for assistance with the search strategy.
Supplemental data
Supplemental data for this article can be accessed here.
Additional information
Funding
References
- Burney LE. Influenza immunization: statement. Public Health Rep. 1960;75(10):944.
- Langmuir AD, Henderson DA, Serfling RE. The epidemiological basis for the control of influenza. Am J Public Health Nations Health. 1964;54:563–571.
- Skowronski DM, Gilbert M, Tweed SA, et al. Effectiveness of vaccine against medical consultation due to laboratory-confirmed influenza: results from a sentinel physician pilot project in British Columbia, 2004–2005. Can Commun Dis Rep. 2005;31(Sep. 18):181–192.
- Skowronski DM, Janjua NZ, De Serres G, et al. A sentinel platform to evaluate influenza vaccine effectiveness and new variant circulation, Canada 2010–2011 season. Clin Infect Dis. 2012;55(3):332–342.
- Ohmit SE, Petrie JG, Malosh RE, et al. Influenza vaccine effectiveness in the community and the household. Clin Infect Dis. 2013;56(10):1363–1369.
- Hoskins TW, Davies JR, Allchin A, et al. Controlled trial of inactivated influenza vaccine containing the a-Hong Kong strain during an outbreak of influenza due to the a-England-42-72 strain. Lancet. 1973;2(7821):116–120.
- Hoskins TW, Davies JR, Smith AJ, et al. Influenza at Christ’s Hospital: March, 1974. Lancet. 1976;1(7951):105–108.
- Hoskins TW, Davies JR, Smith AJ, et al. Assessment of inactivated influenza-A vaccine after three outbreaks of influenza A at Christ’s Hospital. Lancet. 1979;1(8106):33–35.
- Keitel WA, Cate TR, Couch RB, et al. Efficacy of repeated annual immunization with inactivated influenza virus vaccines over a five year period. Vaccine. 1997;15(10):1114–1122.
- Eaton MD, Martin WP. An analysis of serological reactions after vaccination and infection with the virus of influenza A. Am J Epidemiol. 1942;36:255–263.
- McDonald JC, Andrews BE. Diagnostic methods in an influenza vaccine trial. Br Med J. 1955;2(4950):1232–1235.
- Smith DJ, Forrest S, Ackley DH, et al. Variable efficacy of repeated annual influenza vaccination. Proc Natl Acad Sci U S A. 1999;96(24):14001–14006.
- Zimmerman RK, Nowalk MP, Chung J, et al. 2014–2015 influenza vaccine effectiveness in the United States by vaccine type. Clin Infect Dis. 2016;63(12):1564–1573.
- DerSimonian R, Laird N. Meta-analysis in clinical trials. Control Clin Trials. 1986;7(3):177–188.
- Higgins JPT, Thompson SG. Quantifying heterogeneity in a meta-analysis. Stat Med. 2002;21(11):1539–1558.
- Fu C, Xu J, Lin J, et al. Concurrent and cross-season protection of inactivated influenza vaccine against A(H1N1)pdm09 illness among young children: 2012–2013 case-control evaluation of influenza vaccine effectiveness. Vaccine. 2015;33(25):2917–2921.
- Gaglani M, Pruszynski J, Murthy K, et al. Influenza vaccine effectiveness against 2009 pandemic influenza A(H1N1) virus differed by vaccine type during 2013–2014 in the United States. J Infect Dis. 2016;213(10):1546–1556.
- McLean HQ, Thompson MG, Sundaram ME, et al. Influenza vaccine effectiveness in the United States during 2012–2013: variable protection by age and virus type. J Infect Dis. 2015;211(10):1529–1540.
- Jimenez-Jorge S, Savulescu C, Pozo F, et al. Effectiveness of the 2010-11 seasonal trivalent influenza vaccine in Spain: cycEVA study. Vaccine. 2012;30(24):3595–3602.
- Martinez-Baz I, Martinez-Artola V, Reina G, et al. Effectiveness of the trivalent influenza vaccine in Navarre, Spain, 2010–2011: a population-based test-negative case-control study. BMC Public Health. 2013;13:191.
- Ohmit SE, Petrie JG, Malosh RE, et al. Influenza vaccine effectiveness in households with children during the 2012–2013 season: assessments of prior vaccination and serologic susceptibility. J Infect Dis. 2015;211(10):1519–1528.
- Ohmit SE, Petrie JG, Malosh RE, et al. Substantial influenza vaccine effectiveness in households with children during the 2013–2014 influenza season, when 2009 pandemic influenza A(H1N1) virus predominated. J Infect Dis. 2016;213(8):1229–1236.
- Ohmit SE, Thompson MG, Petrie JG, et al. Influenza vaccine effectiveness in the 2011–2012 season: protection against each circulating virus and the effect of prior vaccination on estimates. Clin Infect Dis. 2014;58(3):319–327.
- Pebody RG, Andrews N, Fleming DM, et al. Age-specific vaccine effectiveness of seasonal 2010/2011 and pandemic influenza A(H1N1) 2009 vaccines in preventing influenza in the United Kingdom. Epidemiol Infect. 2013;141(3):620–630.
- Skowronski DM, Janjua NZ, De Serres G, et al. Low 2012–13 influenza vaccine effectiveness associated with mutation in the egg-adapted H3N2 vaccine strain not antigenic drift in circulating viruses. Plos One. 2014;9(3):e92153.
- Skowronski DM, Janjua NZ, Sabaiduc S, et al. Influenza A/subtype and B/lineage effectiveness estimates for the 2011–2012 trivalent vaccine: cross-season and cross-lineage protection with unchanged vaccine. J Infect Dis. 2014;210(1):126–137.
- Skowronski DM, Chambers C, Sabaiduc S, et al. Integrated sentinel surveillance linking genetic, antigenic and epidemiologic monitoring of influenza vaccine-virus relatedness and effectiveness, 2013-14 season. J Infect Dis. 2015;212:726–739.
- Skowronski DM, Chambers C, Sabaiduc S, et al. A perfect storm: impact of genomic variation and serial vaccination on low influenza vaccine effectiveness during the 2014–2015 season. Clin Infect Dis. 2016;63(1):21–32.
- Syrjanen RK, Jokinen J, Ziegler T, et al. Effectiveness of pandemic and seasonal influenza vaccines in preventing laboratory-confirmed influenza in adults: a clinical cohort study during epidemic seasons 2009–2010 and 2010–2011 in Finland. Plos One. 2014;9(9):e108538.
- Valenciano M, Kissling E, Reuss A, et al. Vaccine effectiveness in preventing laboratory-confirmed influenza in primary care patients in a season of co-circulation of influenza A(H1N1)pdm09, B and drifted A(H3N2), I-MOVE Multicentre Case-Control Study, Europe 2014/15. Euro Surveill. 2016;21(7).
- Rondy M, Launay O, Puig-Barberà J, et al. 2012/13 influenza vaccine effectiveness against hospitalised influenza A(H1N1)pdm09, A(H3N2) and B: estimates from a European network of hospitals. Eurosurveillance. 2015;20(2):21011.
- Flannery B, Zimmerman RK, Gubareva LV, et al. Enhanced genetic characterization of influenza A(H3N2) viruses and vaccine effectiveness by genetic group, 2014-2015. J Infect Dis. 2016;214(7):1010–1019.
- McLean HQ, Thompson MG, Sundaram ME, et al. Impact of repeated vaccination on vaccine effectiveness against influenza A(H3N2) and B during 8 seasons. Clin Infect Dis. 2014;59(10):1375–1385.
- Belongia EA, Kieke BA, Donahue JG, et al. Influenza vaccine effectiveness in Wisconsin during the 2007-08 season: comparison of interim and final results. Vaccine. 2011;29(38):6558–6563.
- Belongia EA, Kieke BA, Donahue JG, et al. Effectiveness of inactivated influenza vaccines varied substantially with antigenic match from the 2004–2005 season to the 2006–2007 season. J Infect Dis. 2009;199(2):159–167.
- Treanor JJ, Talbot HK, Ohmit SE, et al. Effectiveness of seasonal influenza vaccines in the United States during a season with circulation of all three vaccine strains. Clin Infect Dis. 2012;55(7):951–959.
- Irving SA, Donahue JG, Shay DK, et al. Evaluation of self-reported and registry-based influenza vaccination status in a Wisconsin cohort. Vaccine. 2009;27(47):6546–6549.
- Castilla J, Navascués A, Fernández-Alonso M, et al. Effectiveness of subunit influenza vaccination in the 2014–2015 season and residual effect of split vaccination in previous seasons. Vaccine. 2016;34(11):1350–1357.
- Petrie JG, Ohmit SE, Johnson E, et al. Persistence of antibodies to influenza hemagglutinin and neuraminidase following one or two years of influenza vaccination. J Infect Dis. 2015;212(12):1914–1922.
- Thompson MG, Naleway A, Fry AM, et al. Effects of repeated annual inactivated influenza vaccination among healthcare personnel on serum hemagglutinin inhibition antibody response to A/Perth/16/2009 (H3N2)-like virus during 2010-11. Vaccine. 2016;34(7):981–988.
- Mosterín Höpping A, McElhaney J, Fonville JM, et al. The confounded effects of age and exposure history in response to influenza vaccination. Vaccine. 2016;34(4):540–546.
- Fonville JM, Wilks SH, James SL, et al. Antibody landscapes after influenza virus infection or vaccination. Science. 2014;346(6212):996–1000.
- Davies JR, Grilli EA. Natural or vaccine-induced antibody as a predictor of immunity in the face of natural challenge with influenza viruses. Epidemiol Infect. 1989;102(2):325–333.
- Miller MS, Gardner TJ, Krammer F, et al. Neutralizing antibodies against previously encountered influenza virus strains increase over time: a longitudinal analysis. Sci Transl Med. 2013;5(198):198ra07.
- Morens DM, Burke DS, Halstead SB. The wages of original antigenic sin. Emerg Infect Dis. 2010;16(6):1023–1024.
- Skowronski DM, Hottes TS, McElhaney JE, et al. Immuno-epidemiologic correlates of pandemic H1N1 surveillance observations: higher antibody and lower cell-mediated immune responses with advanced age. J Infect Dis. 2011;203(2):158–167.
- Skowronski DM, Janjua NZ, De Serres G, et al. Cross-reactive and vaccine-induced antibody to an emerging swine-origin variant of influenza A virus subtype H3N2 (H3N2v). J Infect Dis. 2012;206(12):1852–1861.
- O’Gorman WE, Huang H, Wei Y-L, et al. The split virus influenza vaccine rapidly activates immune cells through Fcγ receptors. Vaccine. 2014;32(45):5989–5997.
- Halstead SB, Mahalingam S, Marovich MA, et al. Intrinsic antibody-dependent enhancement of microbial infection in macrophages: disease regulation by immune complexes. Lancet Infect Dis. 2010;10(10):712–722.
- Ndifon W. A simple mechanistic explanation for original antigenic sin and its alleviation by adjuvants. J R Soc Interface. 2015;12(112):20150627.
- Bodewes R, Fraaij PLA, Kreijtz JHCM, et al. Annual influenza vaccination affects the development of heterosubtypic immunity. Vaccine. 2012;30(51):7407–7410.
- Bodewes R, Kreijtz JH, Rimmelzwaan GF. Yearly influenza vaccinations: a double-edged sword? Lancet Infect Dis. 2009;9(12):784–788.
- Bodewes R, Fraaij PLA, Geelhoed-Mieras MM, et al. Annual vaccination against influenza virus hampers development of virus-specific CD8⁺ T cell immunity in children. J Virol. 2011;85(22):11995–12000.
- Tsang JS. Utilizing population variation, vaccination, and systems biology to study human immunology. Trends Immunol. 2015;36(8):479–493.
- Rizzo C, Bella A, Alfonsi V, et al. Influenza vaccine effectiveness in Italy: age, subtype-specific and vaccine type estimates 2014/15 season. Vaccine. 2016;34(27):3102–3108.
- Garcia-Sastre A. Systems vaccinology informs influenza vaccine immunogenicity. Proc Natl Acad Sci U S A. 2016;113(7):1689–1691.
- Kim JH, Davis WG, Sambhara S, et al. Strategies to alleviate original antigenic sin responses to influenza viruses. Proc Natl Acad Sci U S A. 2012;109(34):13751–13756.
- Kim JH, Skountzou I, Compans R, et al. Original antigenic sin responses to influenza viruses. J Immunol. 2009;183(5):3294–3301.
- Monto AS, Koopman JS, Longini IM Jr. Tecumseh study of illness. XIII. Influenza infection and disease, 1976-1981. Am J Epidemiol. 1985;121(6):811–822.
- Wheatley AK, Kent SJ. Prospects for antibody-based universal influenza vaccines in the context of widespread pre-existing immunity. Expert Rev Vaccines. 2015;14(9):1227–1239.
- Belongia EA, Simpson MD, King JP, et al. Variable influenza vaccine effectiveness by subtype: a systematic review and meta-analysis of test-negative design studies. Lancet Infect Dis. 2016 Aug;16(8):942–951. doi: 10.1016/S1473-3099(16)00129-8. Epub 2016 Apr 6. PMID: 27061888.