ABSTRACT
Introduction: An efficacious vaccine for HIV-1 has been sought for over 30 years to eliminate the virus from the human population. Many challenges have occurred in the attempt to produce a successful immunogen, mainly caused by the basic biology of the virus. Immunogens have been developed focusing on inducing one or more of the following types of immune responses; neutralizing antibodies, non-neutralizing antibodies, and T-cell mediated responses. One way to better present and develop an immunogen for HIV-1 is through the use of nanotechnology and nanoparticles.
Areas covered: This article gives a basic overview of the HIV-1 vaccine field, as well as nanotechnology, specifically nanovaccines. It then covers the application of nanovaccines made from biological macromolecules to HIV-1 vaccine development for neutralizing antibodies, non-neutralizing antibodies, and T-cell-mediated responses.
Expert opinion: Nanovaccines are an area that is ripe for further exploration in HIV-1 vaccine field. Not only are nanovaccines capable of carrying and presenting antigens in native-like conformations, but they have also repeatedly been shown to increase immunogenicity over recombinant antigens alone. Only through further research can the true role of nanovaccines in the development of an efficacious HIV-1 vaccine be established.
1. HIV-1 vaccine introduction
HIV-1 has remained a global pandemic since the 1980s. Despite the successes that have occurred with anti-retroviral therapies, it is unlikely that the virus will be eliminated from the population without an efficacious vaccine [Citation1]. The vaccine development process has been ongoing for almost as long as the pandemic has existed; however, the basic biology of the virus has consistently hampered vaccine development [Citation2]. At the most basic level, HIV-1 is a highly diverse retrovirus that has an error prone reverse transcriptase, which leads to high levels of viral diversity [Citation3]. In addition, HIV-1 DNA can be integrated into the genome of infected cells. Furthermore, there is not a complete understanding of the immune responses necessary to prevent an infection [Citation1]. Moreover, HIV-1 infects CD4+ cells, key components of the immune system, further hampering the immune response [Citation4,Citation5]. Classically, vaccines induce immunological memory, which becomes activated during the early stages of infection and results in a rapid clearing of the infection. The high viral diversity and the ability to of the HIV-1 DNA to be integrated into the genome make a broadly efficacious vaccine a difficult target to obtain.
Immunogen design for HIV-1 has focused on eliciting three different potential methods of protection. The major focus has been on the development of neutralizing antibodies (NAbs), the correlation of protection for many viral disease vaccines [Citation6]. These antibodies target surface-exposed proteins and prevent the virus from infecting the host cells [Citation7]. For HIV-1, the only potential target is the envelope protein (Env), the only exposed surface protein. However, Env is a difficult antigen for vaccine development [Citation7]. Besides high sequence diversity due to reverse transcriptase-induced mutations, Env naturally occurs as a metastable trimer present at low levels on the viral surface [Citation8]. There are less than 20 timers on the surface of each virion, which may potentially reduce the ability of B-cell receptors to recognize the virus [Citation9,Citation10]. Env is also heavily glycosylated, resulting in a glycan shield which reduces the accessibility of the B-cell receptors and antibodies to potential epitopes [Citation11].
While it is possible to raise these antibodies in animal models and in clinical trials, the diversity of the virus has generally meant that immunogens have only been able to induce NAbs against closely matching viral strains or to viruses in the same subtype [Citation3,Citation7,Citation12]. This has led to the search for broadly neutralizing antibodies (bNAbs), which are capable of neutralizing a wide range of different viral strains and subtypes [Citation13]. Some patients infected with HIV-1 can develop these antibodies naturally; however, it is generally late in the infection, after the bNAbs are no longer effective in preventing disease [Citation14,Citation15]. It has been hypothesized that, if it were possible to develop an immunogen capable of inducing these antibodies in a naïve individual, it would be possible to protect against infection, and thus, develop a successful vaccine to HIV-1 [Citation16].
A second target of HIV-1 vaccine design also relies on the development of antibodies; however, these antibodies are non-neutralizing in function [Citation17]. To date, the only Phase III HIV-1 vaccine clinical trial that has demonstrated efficacy is RV144 [Citation18]. In this study, there was a 31.2% reduction in the risk of infection which was correlated with the presence of antibodies that bound to the V1V2 loop of the Env protein [Citation18,Citation19]. These antibodies were not neutralizing, but rather functioned through other mechanisms such as antibody-dependent cell-mediated cytotoxicity (ADCC) [Citation19]. Different attempts have been made to recapitulate this study each attempting to focus the immune response on these different non-neutralizing functions [Citation20]. As is the case with developing bNAbs, a major problem with developing non-neutralizing antibodies is the diversity of the virus and identifying the correct sequence that will be broadly effective against multiple different viral subtypes [Citation12].
The final approach is through the development of T-cell mediated immune responses [Citation21]. In terms of vaccine development, the major goal is the induction of CD8+ cytotoxic lymphocytes (CTLs); however, CD4 + T helper cells are important in the development of memory CTL and B-cell responses [Citation22–25]. T-cell epitopes are present on Env, but also in other viral proteins such as Gag, Pol, and Nef. These proteins are all under stronger selective pressure than Env, because of their role in viral replication making them more conserved targets for vaccine development [Citation26–29]. In animal models, these targets have been demonstrated to be efficacious. However, in human clinical trials focused on CD8+ responses, there was either no protective efficacy demonstrated, or in the Phase IIb STEP trial, there was an increase in infections in the immunized volunteers [Citation26,Citation30–32]. Different and more modern approaches have been taken recently to increase the efficacy and the function of CD8 + T-cell-based vaccines, including the development of mosaic vaccines [Citation26]. This approach uses computational methods to develop immunogens that allow for maximal coverage of viral diversity and/or human haplotype diversity [Citation33,Citation34]. These can either be engineered as proteins that mimic the original structure of the HIV-1 protein and exist as a multivalent vaccine to account for the diversity, or they can exist as multiple epitopes on a de novo designed protein [Citation35–37].
Another important consideration that has become clear with HIV-1 vaccine development is that to generate efficacious immune responses, a heterogenous prime-boost response may be necessary [Citation38]. Studies in preclinical animal models have demonstrated that a prime-boost approach has the potential to increased antibody titers, development of functional antibodies, and increased cellular immune responses [Citation38]. These results are consistent with the findings from RV144 where two different immunogens were given in a prime-boost approach (ALVAC-HIV (vCP1521) and AIDSVAX B/E (gp120)) [Citation18].
It seems highly likely that to develop a broadly efficacious vaccine, two or maybe all three of these approaches need to be combined into one vaccine scheme [Citation16,Citation39]. Logically, it would make sense that an inactivated whole HIV-1 virus may seem like it would be best immunogen because it contains all parts of the virus that could elicit the above responses [Citation40]. However, major safety concerns exist in regards with inactivated vaccines, particularly HIV-1. If there is not 100% inactivation, a vaccinee could be potentially infected [Citation40]. These facts suggest that potentially a different more targeted approach to HIV-1 vaccine development maybe needed to achieve the goal of an end to the virus.
2. Nanovaccines
One modern approach that allows for targeted vaccine development is the use of nanotechnology, a term that refers to particles or assemblies that are between 1 nm to 999 nm in size. In general, nanovaccines are between 1 and 100 nm in size and can be composed of biological macromolecules, polymers, metals, or other materials [Citation41]. Many pathogens, particularly viruses, fall within this size range, which means the host immune system has evolved to deal with particles in this size range [Citation42]. Nanoparticles can be engineered to interact with different immune cells, such as B-cells and/or phagocytic cells making them an excellent platform for vaccine development [Citation43]. Most nanovaccines are decorated on their surface with antigens of interest allowing for direct interaction with B-cell receptors; however, some can carry antigens, or immunostimilatory molecules encapsulated or as a structural feature of the particle itself [Citation44]. It has been demonstrated repeatedly that the particulate display of antigens leads to a stronger immune response than the recombinant protein antigen alone, showing the potential benefits of the use of a nanovaccine in the development of immunogens [Citation45].
2.1. Protein-based nanovaccines
While nanovaccines can be made of any material that falls in the defined size range, in terms of vaccine development, much of the focus has been on particles derived from biological macromolecules [Citation46]. Due to the diversity of secondary structures and ease of manipulation, much of the work on nanovaccines has focused on protein-based assemblies [Citation47]. One approach is the use a virus-like particles (VLPs) ()). These particles are composed of virus capsid proteins capable of self-assembly that mimic their parent viruses, but lack genetic material making them noninfectious [Citation48]. VLPs can be either enveloped (eVLP) in a lipid bilayer or nonenveloped (non-eVLP) and can be made from different parent viral capsid proteins, modified capsid proteins, or from a mosaic of more than one capsid protein [Citation48,Citation49]. Another type of protein-based nanoparticle is the use of a naturally occurring protein assemblies such as ferritin, heat shock protein, or E2 subunit the pyruvate dehydrogenase ()) [Citation47]. These naturally occurring protein assemblies self-assemble into a nanostructures, and by applying basic molecular biology techniques antigens can be engineered to be expressed directly on the protein or can be added after the nanostructure has formed [Citation47].
Figure 1. Different types of nanoparticles made from biological macromolecules used in the development of nanovaccines
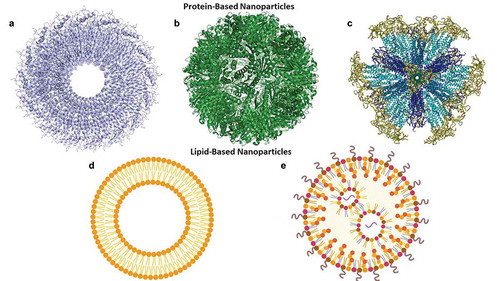
Finally, rationally designed protein assemblies can be used as vaccine platform [Citation46]. These are de novo designed protein assemblies that are engineered to take on the size of the size and shape of a small virus [Citation41]. The major advantage of these types of nanoassemblies is that they have all the advantages of the other protein nanovaccines, but because they are de novo designed, they allow for more control over the structure and number of antigens presented [Citation46]. An example of this technology is the Self-Assembling Protein Nanoparticles (SAPNs) which are composed of protein monomers that spontaneously self-assemble into larger structures in aqueous solutions ()) [Citation52]. SAPNs have repeatedly been demonstrated to be immunogenic and protective in animal models of malaria, influenza, and toxoplasmosis [Citation53–55].
2.2. Lipid-based nanovaccines
Lipids are another biological macromolecule that has been used in the development of nanovaccines ()) [Citation46]. Lipid-based nanoparticles can function in a similar manner as the protein-based nanoparticles, with their surfaces decorated with antigens of interest [Citation56]. These antigens can be added to, or anchored in the membrane with a fatty acid, diacylglycerol, or prenyl chains, or GPI (glycosylphosphatidylinositol) that allow for direct addition to the membrane during formation of the liposomes. Alternatively, the antigens can also be added using tags or chemically cross-linked to anchors present in the bilayer [Citation56]. One specific-type of a lipid-based nanoparticle are liposomes, which are defined as a phospholipid bilayer (unilamellar), or multiple phospholipid bilayers (multilamellar) surrounding an aqueous core, which have frequently been decorated on their surface with different antigens [Citation57]. Another major use of liposomes is to carry cargo, such as drugs, and in terms of vaccines, immunomodulatory molecules encapsulated in the aqueous core or anchored in the membrane [Citation58]. An example of the latter is Army Liposome Formulation containing monophosphoryl lipid A (MPLA) and QS-21 (ALFQ) in which the MPLA head group and QS-21 carbohydrate activates immune cells [Citation59].
A specific type of liposome used as a vaccine carrier is the virosome, which also contain a phospholipid bilayer. They also contain viral glycoproteins embedded in the membrane that enables the virosome to target different cell types [Citation60]. For example, an influenza-based virosome contains hemagglutinin, neuraminidase, as well as the antigen of interest. Just like liposomes, virosomes can contain drugs, or immunostimilatory molecules in their cores [Citation60].
Another major approach for lipid-based nanoparticles for the development of HIV-1 vaccines is as a carrier for nucleic acid vaccines ()) [Citation61,Citation62]. While similar in concept to liposomes, these particles do not have to contain a bilayer, but can also be a single-layer micelle and are referred to as lipid nanoparticles (LNP) [Citation63]. Composed of cationic lipids or ionizable lipids, these LNPs protect their nucleic acid cargo from nucleases. Depending on the lipid composition, LNPs can influence the interaction with host cells and proteins and facilitate the passage of the nucleic acid across the cell membrane and allow entry into the host cell [Citation63,Citation64].
2.3. Nanovaccines and humoral responses
Nanovaccines designed for the induction of humoral immune responses focus on the presentation of Env. Different candidates have focused on either the precursor protein gp160 one or multiple processed parts of Env (gp140, gp120 or gp41), or specific regions of Env such as the different variable loops or MPER [Citation65–69]. By presenting Env or its various subunits on nanoparticles, many of the factors that are believed to be important for HIV-1 immunogen development can be actively incorporated into the design, potentially allowing for better responses. Nanoparticle immunogens can also carry Env trimers, similar to native trimeric Env. Many Env were engineered into a native-like structures, known as SOSIPs, which were engineered from gp140 with disulfide bridges that lock the structure into a native-like prefusion conformation [Citation69–73]. These proteins can induce functional responses in immunized animals and have also been presented on the surface of nanoparticles [Citation67,Citation68,Citation74–76]. In addition, nanovaccines can be engineered to present the Env protein or subunits in native-like conformation without the addition of the extra disulfide bridges [Citation65,Citation77].
Nanoparticles can also be used to address the issue of the low density of Env presented on the surface of the virus. Different nanovaccines can be engineered with various symmetries and geometries that allow for increased presentation of the Env or specific Env subunit. Besides being presented at a higher density on these particles, the antigens can be engineered to be presented at a distance of 5–10 nm, the optimal distance for B-cell activation [Citation78–80]. This change allows for better cross-linking of the B-cell receptor, leading to increased immune responses. With this change, higher titers of antibodies can be generated in comparison to recombinant proteins, or even to the HIV-1 virus itself.
2.4. Nanovaccines and neutralization
Much of the focus of the development of nanovaccines for inducing antibodies against HIV-1 has focused on NAbs [Citation81]. Attempts have been made to make a VLP from the HIV-1 virus itself and, while capable of inducing Tier-2 NAbs, these types of vaccines have many of the same problems as the whole HIV-1 virus, including low-levels of Env presentation, unstable and incomplete Env spikes, and further than ideal distances between the spikes [Citation82,Citation83]. Other attempts have been made to generate fusion VLPs, fusing HIV-1 Gag or capsid proteins from other viruses with HIV-1 proteins such as gp160, gp120, trimeric gp140, V3 loop, V1V2 loop, and the CD4 binding site, resulted in mixed findings with only some capable of inducing NAbs in animal models [Citation84–94]. When focusing on responses specifically to gp41, animals immunized with a Gag-VLP presenting MPER did not induce high titers of NAbs. However, presentation of gp41 itself on non-eVLPs from other viruses resulted in some neutralization in animal models [Citation95,Citation96].
Env and Env derivatives have also been displayed on naturally occurring protein assemblies to elicit NAbs [Citation81]. One approach has been to use ferritin nanoparticles, which are naturally occurring iron binding nanocages composed of 24 different monomers that self-assemble [Citation97]. Presenting Env as a SOSIP on ferritin nanoparticles increases its immunogenicity over the matching soluble SOSIP; however, it did not always significantly increase neutralization [Citation74,Citation98,Citation99]. This could be related to the sequence of the SOSIP or to the fact that only eight timers are presented on the ferritin nanovaccine [Citation81]. Another naturally occurring protein assembly, lumazine synthase from Aquifex aeolicus, also self-assembles from 60 monomers to form a nanoparticle that presents 20 trimers [Citation100]. These particles have been decorated with the outer domain (eOD) of gp120, which was engineered to stimulate the predicted germ-line precursors of VRC01 type bNAbs [Citation101]. Transgenic mice could raise broadly neutralizing antibodies after vaccination and this candidate is currently waiting to be tested Phase I clinical trials [Citation81,Citation102].
Liposomes have also been used in a similar capacity as protein-based nanoparticles to induce the production of NAbs [Citation103]. Much of the work with this type of nanovaccines has focused on the presentation of different peptides or proteins to the host immune system to increase their immunogenicity [Citation81]. One of the major goals has been the induction of antibodies to the MPER region of gp41 because bnAbs have been recovered from infected individuals, which bind not only to the protein but also to the viral envelope [Citation104–106]. Studies that included either the entire gp41 subunit or MPER peptides on the surface of liposomes resulted in the higher titers of antibodies than peptide or protein by itself and could induce weak to moderate levels of neutralization with some cross reactivity [Citation66,Citation107,Citation108]. Virosomes have also been used to present gp41 in a mucosal vaccination scheme that has led to the induction of neutralizing antibodies in the vagina of immunized NHPs and protection against challenge in most animals [Citation109]. In a Phase I clinical trial, mucosal antibodies were also identified in most trial participants [Citation110].
Another approach has been to use liposomes to present Env trimers on their surface. Different studies have attempted the presentation of a SOSIP on a liposome [Citation67,Citation111]. As expected, the presentation on a liposome increased the immunogenicity, and resulted in neutralization activity [Citation67,Citation111]. A liposome-based nanovaccine was also engineered to overcome the obstacle of the glycan shield [Citation111]. In attempt to focus the response on the CD4 binding site, glycans were modified and a prime boost approach was taken with different glycan profiles ultimately leading to the development of cross-neutralizing Abs, with the recovery of one bNAb capable of binding to a new glycan/protein epitope [Citation111].
mRNA vaccines encapsulated by lipid nanoparticles have emerged as one of the potentially most important areas in the field of vaccinology [Citation112]. In terms of HIV-1, two studies have examined the ability of mRNA vaccines encoding for Env to induce neutralizing antibodies [Citation113,Citation114]. In the first study, rabbits and NHPs were able to raise neutralizing antibodies, with NHPs capable of raising tier 2 NAbs [Citation113]. These results were further expanded upon to demonstrate that nucleoside modified mRNA induced NAbs and with a durability of at least 41 weeks to a sequence-matched protein-based immunogen [Citation114].
2.5. Nanovaccines and non-neutralizing antibodies
While most of the attention on the development of humoral responses from an HIV-1 vaccine has focused on NAbs, non-neutralizing antibodies are the only antibodies that have correlated with protection in a human clinical trial (RV144) [Citation18,Citation19]. Non-neutralizing effects are mediated by the Fc region of the induced antibody binding to the Fc receptor on effector cells leading to ADCC, Antibody-dependent phagocytosis (ADCP), Antibody-dependent cell-mediated virus inhibition (ADCVI), and Antibody-dependent complement activation (ADC) [Citation20]. In RV144, ADCC was the response that was observed in individuals that had a lower rate of infection [Citation18,Citation19]. Potentially, the importance of these responses may have been underestimated or not thoroughly studied due to the difficulty and variability in the assays used for measurement [Citation115,Citation116]. Antibodies also do not fall distinctly into either the neutralization or the non-neutralization categories, but rather can function through multiple pathways at the same time [Citation117–121]. Studies have indicated that 25–45% of the antiviral activity from the passive transfer of previously isolated neutralizing mAbs into NHP models is correlated with non-neutralizing responses, indicating its important role in successful vaccine development [Citation122–124].
One of the first studies to examine non-neutralizing function induced by a nanovaccine focused on a VLP that presented different fragments of gp41 [Citation95]. VLPs that presented the HR2 region were capable of inducing ADCC activity, while VLPs containing other regions could not [Citation95]. VLPs were again used in a prime-boost immunization study utilizing two DNA prime immunizations, followed by two immunizations with a Gag-VLP. This immunization regimen resulted in the development of ADCC and ADCIV activity in mice [Citation125]. In another study, immunization with a SAPN presenting the V1V2 loop of Env resulted in ADCP activity in immunized animals [Citation77]. NHPs immunized with a mucosal vaccine were capable of generating antibodies in the vagina capable of inducing ADCC [Citation60]. Rabbits and NHPs that were immunized with mRNA vaccines encapsulated in LNPs both produced antibodies capable of inducing ADCC [Citation113]. A further mRNA study demonstrated that in immunized NHPs induce antibodies that were capable of inducing both ADCC and ADCP activity [Citation114].
3. Nanovaccines and cell-based immunity
Some of the first work on developing nanovaccines designed to induce either CD4+ or CD8+ responses focused on VLPs [Citation126]. Much of the initial work focused on the development Gag nanoparticles with or without other antigens such as Env attached. These studies demonstrated an increase in CTL, Th responses, and depending on attached antigens, increased antibody titers [Citation90–92,Citation94]. Additional strategies were developed to improve on these results. The first was to incorporate CD40L into the Gag-VLP, which was intended to bind to CD40 and lead to activation of dendritic cells with the goal of increased immunogenicity [Citation127,Citation128]. There was an increase in CD4+ and CD8+ cell responses to p24 in mice that were immunized with the CD40L containing VLPs in comparison to the VLPs by themselves [Citation127]. Immunization of NHPs with a SHIV Gag-VLP that also contained Env and CD40L resulted in both an increase in cellular and humoral responses when compared to the SHIV Gag-VLP lacking CD40L [Citation129]. A conceptually similar VLP containing Env and CD40L was used in a SIV challenge model. Immunization resulted in increased anti-Env antibodies and increased CD4+ and CD8+ responses including increased breadth but was unable to prevent infection. However, animals immunized with the SHIV Gag-VLP containing CD40L had had better control of viremia [Citation129].
The major role of VLPs in T-cell-based vaccines has been as the boost in a prime-boost approach [Citation130–134]. One study used a DNA prime and boosted with either a Gag-VLP that presented either reverse transcriptase or a Tat:Nef fusion protein. VLP boosting increased CD4+ and CD8+ responses in comparison to two immunizations with just DNA [Citation134]. In another similar approach, there was an increase in CD4+ and CD8+ responses in mice that received two doses of a DNA vaccine followed by two doses of a Gag-VLP [Citation125,Citation131]. Other investigations demonstrated that in mice, animals that were boosted with either a VLP presenting gp120 and a Recombinant Modified Vaccinia Virus Ankara vector resulted in increased CD4+ and CD8+ responses in comparison to mice that were only immunized with DNA further demonstrating the importance of prime-boost in development of functional immune responses to HIV-1 [Citation130].
Liposomes and lipid nanoparticle-based immunogens have also been demonstrated to increase CD4+ and CD8+ responses in immunized preclinical models [Citation135–138]. Studies that encapsulated either a V3 peptide or HIV-1 Gag p24 in liposomes containing different immunostimulatory molecules both demonstrated the capability of inducing cellular immune response in mouse models [Citation137,Citation138]. The encapsulated HIV-1 Gag p24 was capable able to induce Gag-specific central memory CD8 + T-cells and effector CD4+ [Citation138]. Lipid nanoparticles containing mRNA vaccines have also been used to induce CD4+ and CD8 + T-cell responses in mouse models [Citation135,Citation136]. A self-amplifying mRNA encoding a bivalent mosaic vaccine immunogen tHIVconsvX was able to induce both CD4+ and CD8+ responses in immunized animals while also inducing a memory response [Citation136,Citation139]. Similar results were obtained when the vaccine formulation was increased to a tetravalent mosaic vaccine allowing for increased coverage of circulating HIV-1 strains [Citation135].
4. Conclusions
Despite years of work, an HIV-1 vaccine has remained extremely challenging to develop. HIV-1 is a difficult pathogen to target, and attempts have been made to induce both antibody and cell-mediated responses by immunization. It seems likely that in the end a successful vaccine will most likely need to induce both responses concurrently to protect the host from infection. Nanotechnology offers a potential solution for the development of immunogens for HIV-1 vaccine. Different types of nanovaccines have been developed such as VLPs, naturally occurring protein assemblies, rationally designed protein assemblies, liposomes, and lipid nanoparticles. These nanovaccines can be engineered to present antigens on their surface to increase immunogenicity or carry antigens or immunostimulatory molecules in their cores all increasing the efficacy of the vaccine over soluble antigens alone. Many different nanovaccine technologies have been applied to studies seeking the development of a successful HIV-1 vaccines in preclinical models and these candidates have been able to induce NAbs, non-neutralizing antibodies, CD4+, and CD8+ responses. They have also functioned as single immunogens and as part of prime-boost vaccine schemes. The role and potential of nanovaccines in the development of an HIV-1 vaccine has only really begun to be explored and further studies may demonstrate even a greater role in the development of an efficacious vaccine.
5. Expert opinion
The development of an efficacious HIV-1 vaccine has and remains a major goal of the scientific community to reduce global morbidity and mortality. Different approaches have come and gone as to what ultimately may work as a successful immunogen. These debates have been made more difficult by challenges that occur caused by the sheer viral diversity, the lack of clues as to what is the most important immune response that results in prevention of infection or clearance of the virus, and the lack of a good animal model that completely replicates HIV-1 infection. The knowledge that has been amassed over the last almost 40 years has allowed for the development of different strategies such as native-like trimers, mosaic sequences, and prime-boost vaccine schedules that may result in a better potentially more efficacious immune response.
The major function of nanotechnology previously and moving forward in vaccine development is as a delivery system. Nanovaccines in countless preclinical and clinical trials have demonstrated that they can induce stronger immune responses then soluble protein alone. In the HIV-1 field, this approach has been taken; however, there is still a lot of work that can be done. Modern approaches to antigen design such as the native-like SOSIPs and mosaic vaccines all can or have been conducted with different nanoparticles. Besides the benefit as particulate displays, nanotechnology can also be engineered to directly interact with specific cell-types based on factors such as size, shape, or molecules contained on the particle. Nanovaccines can also be engineered to present specific epitopes in native-like conformation, reducing the off-target, or nonproductive immune responses and instead focusing only on the desired immune response. As technologies continue to be developed and improved upon, the role of nanovaccines in the development of an HIV-1 vaccine will only continue to grow.
The nanovaccine field is extremely diverse and broad field. No one nanoparticle will be the perfect fit for every single antigen, or desired immune response. Many factors need to be considered when choosing the best approach for development of the nanovaccine. It is a dynamic process and should not be looked at as simply sticking an antigen on a preexisting nanoparticle, by rather a series of optimization steps of the antigen, carrier, adjuvant, and vaccine formulation. Both the nanoparticle and the antigen/antigens need to be biophysically optimized, to make sure that the antigen is properly displayed, the optimal density and distance is obtained, and that the formulation is stable and immunogenic. Only by addressing all these issues will an efficacious nanovaccine formulation be generated. It has been consistently demonstrated prime-boost approaches may be the way forward with HIV-1 vaccine. Nanovaccines can play an important role in this regard forming either the prime or boost. The opportunities and combinations are endless for the different strategies to use, including priming with a larger antigen, such as a SOSIP presented on a nanoparticle, and the boosting with regions of the antigen, such as the native-like presentation of the V2 loop, on a properly engineered nanoparticle can potentially lead to the further the development of antibodies to this specific region. This approach also can also be applied to allow for coverage of a wider range of the circulating HIV-1 diversity using different boosts from different strains of HIV-1 leading to a more broadly efficacious vaccine.
For HIV-1 as with all vaccines, the addition of an adjuvant can not only increase the response to an immunogen but also change that response in many ways [Citation140,Citation141]. Adjuvants come in many different forms including aluminum salts, pathogen-associated molecular patterns (PAMPs) or PAMP-like agonists, or nanoparticles themselves. While many of these have been used for years, there is not a complete understanding of the mechanism of action, because in most cases it is immunogen specific [Citation142]. In considering any nanoparticle-based vaccine formulation for use as a prophylactic HIV-1 vaccine beyond the choice of immunogen, the choice of adjuvant will also need to be considered. One of the major changes with HVTN702 from RV144 was the change of adjuvant from aluminum hydroxide to MF59 which could have been one of the reasons for the lack of protective efficacy in the HVTN702 study. In moving forward with HIV-1 nanoparticle-based vaccine development, there needs to be a greater consideration of not only the immunogen, but also the adjuvant as well. Some groups have already started this work leading to effects such as increased titers and avidity; however, it does warrant further study [Citation65,Citation143]. Potentially, by including more modern adjuvant systems such as ALFQ, an efficacious vaccine can be developed [Citation59].
The use of nanovaccines in the field of HIV-1 vaccinology will continue to expand over the next five years. There are still many opportunities for the development and application of current nanovaccine technologies in combination with new antigens and new vaccine delivery schemes. It is also clear that the field of vaccinology has entered a new phase with emergence of mRNA vaccines. In most cases, it is essential to deliver mRNA vaccines with a nanoparticle, and the rate of application will only increase over the next couple of years. While the mRNA vaccine approach has been demonstrated to be efficacious in application to COVID-19, the long-term durability of responses is still an unknown factor. Potentially, combining an mRNA vaccine prime with a nanoparticle-delivered protein boost could result in a better immune response. For the HIV-1 field, this could lead to boosting with different antigens to allow for development of responses against the ever-changing HIV-1 virus.
Article highlights
HIV-1 vaccine development has been hampered by the mutation rate, viral diversity, and lack of a complete understanding of the immune response necessary to prevent the infection among other reasons.
Immunogens for HIV-1 vaccines have focused primarily on inducing neutralizing antibodies; however, work has been done on inducing both non-neutralizing and T-cell-mediated responses as well.
Nanoparticles have been used as a platform for the development of nanovaccines, which generally increase the immunogenicity of antigens.
While many different types of nanoparticles exist, the ones derived from biological macromolecules have been the most commonly used to date in the development of vaccine candidates.
Different nanovaccine platforms have been used for HIV-1 vaccine development, all resulting in different levels of success and immune responses based upon antigen choice, animal models, and the types of assays run.
Declaration of interest
The authors have no relevant affiliations or financial involvement with any organization or entity with a financial interest in or financial conflict with the subject matter or materials discussed in the manuscript. This includes employment, consultancies, honoraria, stock ownership or options, expert testimony, grants or patents received or pending, or royalties.
Reviewer disclosures
Peer reviewers on this manuscript have no relevant financial or other relationships to disclose.
Author Contributions
CPK and GRM conceived of the manuscript. CPK wrote the initial draft and both CPK and GRM edited it.
Disclaimer
The views expressed are those of the authors and should not be construed to represent the positions of the U.S. Army or the Department of Defense or the Henry M. Jackson Foundation.
Acknowledgments
The authors would like to thank Peter Burkhard for supplying the model of the Self-Assembling Protein Nanoparticle.
Additional information
Funding
References
- Ng’uni T, Chasara C, Ndhlovu ZM. Major scientific hurdles in HIV vaccine development: historical perspective and future directions. Front Immunol. 2020;11:590780.
- Hsu DC, O’Connell RJ. Progress in HIV vaccine development. Hum Vaccin Immunother. 2017;13(5):1018–1030.
- Hemelaar J. The origin and diversity of the HIV-1 pandemic. Trends Mol Med. 2012;18(3):182–192.
- Ventura JD. Human immunodeficiency virus 1 (HIV-1): viral latency, the reservoir, and the cure. Yale J Biol Med. 2020;93(4):549–560.
- Rios A. Fundamental challenges to the development of a preventive HIV vaccine. Curr Opin Virol. 2018;29:26–32.
- Amanna IJ, Slifka MK. Successful vaccines. Curr Top Microbiol Immunol. 2020;428:1–30.
- Klasse PJ, Ozorowski G, Sanders RW, et al. Env exceptionalism: why are HIV-1 Env glycoproteins atypical immunogens? Cell Host Microbe. 2020;27(4):507–518.
- Del Moral-Sanchez I, Sliepen K. Strategies for inducing effective neutralizing antibody responses against HIV-1. Expert Rev Vaccines. 2019;18(11):1127–1143.
- Zhu P, Chertova E, Bess J Jr, et al. Electron tomography analysis of envelope glycoprotein trimers on HIV and simian immunodeficiency virus virions. Proc Natl Acad Sci U S A. 2003;100(26):15812–15817.
- Schiller J, Chackerian B. Why HIV virions have low numbers of envelope spikes: implications for vaccine development. Plos Pathog. 2014;10(8):e1004254.
- Crispin M, Ward AB, Wilson IA. Structure and immune recognition of the HIV glycan shield. Annu Rev Biophys. 2018;47:499–523.
- Stefic K, Bouvin-Pley M, Braibant M, et al. Impact of HIV-1 diversity on its sensitivity to neutralization. Vaccines (Basel). 2019;7(3).
- Pauthner MG, Hangartner L. Broadly neutralizing antibodies to highly antigenically variable viruses as templates for vaccine design. Curr Top Microbiol Immunol. 2020;428:31–87.
- Doria-Rose NA, Schramm CA, Gorman J, et al. Developmental pathway for potent V1V2-directed HIV-neutralizing antibodies. Nature. 2014;509(7498):55–62.
- Liao HX, Lynch R, Zhou T, et al. Co-evolution of a broadly neutralizing HIV-1 antibody and founder virus. Nature. 2013;496(7446):469–476.
- Haynes BF, Burton DR, Mascola JR. Multiple roles for HIV broadly neutralizing antibodies. Sci Transl Med. 2019;11(516).
- Excler JL, Ake J, Robb ML, et al. Nonneutralizing functional antibodies: a new “old” paradigm for HIV vaccines. Clin Vaccine Immunol. 2014;21(8):1023–1036.
- Rerks-Ngarm S, Pitisuttithum P, Nitayaphan S, et al. Vaccination with ALVAC and AIDSVAX to prevent HIV-1 infection in Thailand. N Engl J Med. 2009;361(23):2209–2220.
- Haynes BF, Gilbert PB, McElrath MJ, et al. Immune-correlates analysis of an HIV-1 vaccine efficacy trial. N Engl J Med. 2012;366(14):1275–1286.
- Su B, Dispinseri S, Iannone V, et al. Update on Fc-mediated antibody functions against HIV-1 beyond neutralization. Front Immunol. 2019;10:2968.
- Korber B, Fischer W. T cell-based strategies for HIV-1 vaccines. Hum Vaccin Immunother. 2020;16(3):713–722.
- Hel Z, Nacsa J, Tryniszewska E, et al. Containment of simian immunodeficiency virus infection in vaccinated macaques: correlation with the magnitude of virus-specific pre- and postchallenge CD4+ and CD8+ T cell responses. J Immunol. 2002;169(9):4778–4787.
- Ostrowski MA, Justement SJ, Ehler L, et al. The role of CD4+ T cell help and CD40 ligand in the in vitro expansion of HIV-1-specific memory cytotoxic CD8+ T cell responses. J Immunol. 2000;165(11):6133–6141.
- Shedlock DJ, Shen H. Requirement for CD4 T cell help in generating functional CD8 T cell memory. Science. 2003;300(5617):337–339.
- Ahrends T, Busselaar J, Severson TM, et al. CD4(+) T cell help creates memory CD8(+) T cells with innate and help-independent recall capacities. Nat Commun. 2019;10(1):5531.
- Mothe B, Brander C. HIV T-cell vaccines. Adv Exp Med Biol. 2018;1075:31–51.
- Mothe B, Llano A, Ibarrondo J, et al. Definition of the viral targets of protective HIV-1-specific T cell responses. J Transl Med. 2011;9:208.
- Letourneau S, Im EJ, Mashishi T, et al. Design and pre-clinical evaluation of a universal HIV-1 vaccine. plos One. 2007;2(10):e984.
- Borthwick N, Ahmed T, Ondondo B, et al. Vaccine-elicited human T cells recognizing conserved protein regions inhibit HIV-1. Mol Ther. 2014;22(2):464–475.
- Duerr A, Huang Y, Buchbinder S, et al. Extended follow-up confirms early vaccine-enhanced risk of HIV acquisition and demonstrates waning effect over time among participants in a randomized trial of recombinant adenovirus HIV vaccine (Step Study). J Infect Dis. 2012;206(2):258–266.
- McElrath MJ, De Rosa SC, Moodie Z, et al. HIV-1 vaccine-induced immunity in the test-of-concept step study: a case-cohort analysis. Lancet. 2008;372(9653):1894–1905.
- Buchbinder SP, Mehrotra DV, Duerr A, et al. Efficacy assessment of a cell-mediated immunity HIV-1 vaccine (the step study): a double-blind, randomised, placebo-controlled, test-of-concept trial. Lancet. 2008;372(9653):1881–1893.
- Korber BT, Letvin NL, Haynes BF. T-cell vaccine strategies for human immunodeficiency virus, the virus with a thousand faces. J Virol. 2009;83(17):8300–8314.
- Fischer W, Perkins S, Theiler J, et al. Polyvalent vaccines for optimal coverage of potential T-cell epitopes in global HIV-1 variants. Nat Med. 2007;13(1):100–106.
- Barouch DH, O’Brien KL, Simmons NL, et al. Mosaic HIV-1 vaccines expand the breadth and depth of cellular immune responses in rhesus monkeys. Nat Med. 2010;16(3):319–323.
- Santra S, Liao HX, Zhang R, et al. Mosaic vaccines elicit CD8+ T lymphocyte responses that confer enhanced immune coverage of diverse HIV strains in monkeys. Nat Med. 2010;16(3):324–328.
- Nkolola JP, Bricault CA, Cheung A, et al. Characterization and immunogenicity of a novel mosaic M HIV-1 gp140 trimer. J Virol. 2014;88(17):9538–9552.
- Excler JL, Kim JH. Novel prime-boost vaccine strategies against HIV-1. Expert Rev Vaccines. 2019;18(8):765–779.
- Haynes BF, Kelsoe G, Harrison SC, et al. B-cell-lineage immunogen design in vaccine development with HIV-1 as a case study. Nat Biotechnol. 2012;30(5):423–433.
- Kang CY, Gao Y. Killed whole-HIV vaccine; employing a well established strategy for antiviral vaccines. AIDS Res Ther. 2017;14(1):47.
- Doll TA, Raman S, Dey R, et al. Nanoscale assemblies and their biomedical applications. J R Soc Interface. 2013;10(80):20120740.
- Kelly HG, Kent SJ, Wheatley AK. Immunological basis for enhanced immunity of nanoparticle vaccines. Expert Rev Vaccines. 2019;18(3):269–280.
- Zaheer T, Pal K, Zaheer I. Topical review on nano-vaccinology: biochemical promises and key challenges. Process Biochem. 2021;100:237-244.
- Facciola A, Visalli G, Lagana P, et al. The new era of vaccines: the “nanovaccinology.” Eur Rev Med Pharmacol Sci. 2019;23(16):7163–7182.
- Irvine DJ, Read BJ. Shaping humoral immunity to vaccines through antigen-displaying nanoparticles. Curr Opin Immunol. 2020;65:1–6./mixed-citation>
- Karch CP, Burkhard P. Vaccine technologies: from whole organisms to rationally designed protein assemblies. Biochem Pharmacol. 2016;120:1–14.
- Butkovich N, Li E, Ramirez A, et al. Advancements in protein nanoparticle vaccine platforms to combat infectious disease. Wiley Interdiscip Rev Nanomed Nanobiotechnol. 2020;13:e1681.
- Roldao A, Mellado MC, Castilho LR, et al. Virus-like particles in vaccine development. Expert Rev Vaccines. 2010;9(10):1149–1176.
- Donaldson B, Lateef Z, Walker GF, et al. Virus-like particle vaccines: immunology and formulation for clinical translation. Expert Rev Vaccines. 2018;17(9):833–849.
- Weis F, Beckers M, von der Hocht I, et al. Elucidation of the viral disassembly switch of tobacco mosaic virus. EMBO Rep. 2019;20(11):e48451.
- Zhang X, Meining W, Fischer M, et al. X-ray structure analysis and crystallographic refinement of lumazine synthase from the hyperthermophile aquifex aeolicus at 1.6 A resolution: determinants of thermostability revealed from structural comparisons. J Mol Biol. 2001;306(5):1099–1114.
- Karch CP, Matyas GR, Burkhard P, et al. Self-assembling protein nanoparticles: implications for HIV-1 vaccine development. Nanomedicine (Lond). 2018;13(17):2121–2125.
- El Bissati K, Zhou Y, Paulillo SM, et al. Engineering and characterization of a novel self assembling protein for toxoplasma peptide vaccine in HLA-A*11:01, HLA-A*02:01 and HLA-B*07:02 transgenic mice. Sci Rep. 2020;10(1):16984.
- Karch CP, Li J, Kulangara C, et al. Vaccination with self-adjuvanted protein nanoparticles provides protection against lethal influenza challenge. Nanomedicine. 2017;13(1):241–251.
- Kaba SA, Karch CP, Seth L, et al. Self-assembling protein nanoparticles with built-in flagellin domains increases protective efficacy of a Plasmodium falciparum based vaccine. Vaccine. 2018;36(6):906–914.
- Nisini R, Poerio N, Mariotti S, et al. The multirole of liposomes in therapy and prevention of infectious diseases. Front Immunol. 2018;9(155):1–29.
- Alving CR, Beck Z, Matyas GR, et al. Liposomal adjuvants for human vaccines. Expert Opin Drug Deliv. 2016;13(6):807–816.
- Filipczak N, Pan J, Yalamarty SSK, et al. Recent advancements in liposome technology. Adv Drug Deliv Rev. 2020;156:4-22.
- Alving CR, Peachman KK, Matyas GR, et al. Army liposome formulation (ALF) family of vaccine adjuvants. Expert Rev Vaccines. 2020;19(3):279–292.
- Corthesy B, Bioley G. Lipid-based particles: versatile delivery systems for mucosal vaccination against infection. Front Immunol. 2018;9:1-20.
- Kim J, Eygeris Y, Gupta M, et al. Self-assembled mRNA vaccines. Adv Drug Deliv Rev. 2021;170:83–112.
- Tejeda-Mansir A, Garcia-Rendon A, Guerrero-German P. Plasmid-DNA lipid and polymeric nanovaccines: a new strategic in vaccines development. Biotechnol Genet Eng Rev. 2019;35(1):46–68.
- Kraft JC, Freeling JP, Wang Z, et al. Emerging research and clinical development trends of liposome and lipid nanoparticle drug delivery systems. J Pharm Sci. 2014;103(1):29–52.
- Samaridou E, Heyes J, Lutwyche P. Lipid nanoparticles for nucleic acid delivery: current perspectives. Adv Drug Deliv Rev. 2020;154-155:37–63.
- Karch CP, Bai H, Torres OB, et al. Design and characterization of a self-assembling protein nanoparticle displaying HIV-1 Env V1V2 loop in a native-like trimeric conformation as vaccine antigen. Nanomedicine. 2019;16:206–216.
- Shao S, Huang WC, Lin C, et al. An engineered biomimetic MPER peptide vaccine induces weakly HIV neutralizing antibodies in mice. Ann Biomed Eng. 2020;48(7):1991–2001.
- Ingale J, Stano A, Guenaga J, et al. High-density array of well-ordered HIV-1 spikes on synthetic liposomal nanoparticles efficiently activate B cells. Cell Rep. 2016;15(9):1986–1999.
- Brouwer PJM, Antanasijevic A, Berndsen Z, et al. Enhancing and shaping the immunogenicity of native-like HIV-1 envelope trimers with a two-component protein nanoparticle. Nat Commun. 2019;10(1):4272.
- Sanders RW, Derking R, Cupo A, et al. A next-generation cleaved, soluble HIV-1 Env trimer, BG505 SOSIP.664 gp140, expresses multiple epitopes for broadly neutralizing but not non-neutralizing antibodies. PLoS Pathog. 2013;9(9):e1003618.
- Sok D, Burton DR. Recent progress in broadly neutralizing antibodies to HIV. Nat Immunol. 2018;19(11):1179–1188.
- Sanders RW, Moore JP. Native-like Env trimers as a platform for HIV-1 vaccine design. Immunol Rev. 2017;275(1):161–182.
- Escolano A, Dosenovic P, Nussenzweig MC. Progress toward active or passive HIV-1 vaccination. J Exp Med. 2017;214(1):3–16.
- Dey AK, Cupo A, Ozorowski G, et al. cGMP production and analysis of BG505 SOSIP.664, an extensively glycosylated, trimeric HIV-1 envelope glycoprotein vaccine candidate. Biotechnol Bioeng. 2018;115(4):885–899.
- Sliepen K, Ozorowski G, Burger JA, et al. Presenting native-like HIV-1 envelope trimers on ferritin nanoparticles improves their immunogenicity. Retrovirology. 2015;12(82):1–5.
- He L, De Val N, Morris CD, et al. Presenting native-like trimeric HIV-1 antigens with self-assembling nanoparticles. Nat Commun. 2016;7(12041):1–36.
- Antanasijevic A, Ueda G, Brouwer PJM, et al. Structural and functional evaluation of de novo-designed, two-component nanoparticle carriers for HIV Env trimer immunogens. PLoS Pathog. 2020;16(8):e1008665.
- Karch CP, Paquin-Proulx D, Eller MA, et al. Impact of the expression system on the immune responses to self-assembling protein nanoparticles (SAPNs) displaying HIV-1 V1V2 loop. Nanomedicine. 2020;29(102255):1–13.
- Bachmann MF, Zinkernagel RM. Neutralizing antiviral B cell responses. Annu Rev Immunol. 1997;15:235–270.
- Vogelstein B, Dintzis RZ, Dintzis HM. Specific cellular stimulation in the primary immune response: a quantized model. Proc Natl Acad Sci U S A. 1982;79(2):395–399.
- Dobrovolskaia MA, Aggarwal P, Hall JB, et al. Preclinical studies to understand nanoparticle interaction with the immune system and its potential effects on nanoparticle biodistribution. Mol Pharm. 2008;5(4):487–495.
- Brinkkemper M, Sliepen K. Nanoparticle vaccines for inducing HIV-1 neutralizing antibodies. Vaccines (Basel). 2019;7(3):1–14.
- Crooks ET, Osawa K, Tong T, et al. Effects of partially dismantling the CD4 binding site glycan fence of HIV-1 envelope glycoprotein trimers on neutralizing antibody induction. Virology. 2017;505:193–209.
- Crooks ET, Tong T, Chakrabarti B, et al. Vaccine-elicited tier 2 HIV-1 neutralizing antibodies bind to quaternary epitopes involving glycan-deficient patches proximal to the CD4 binding site. PLoS Pathog. 2015;11(5):e1004932.
- Kim M, Qiao Z, Yu J, et al. Immunogenicity of recombinant human immunodeficiency virus type 1-like particles expressing gp41 derivatives in a pre-fusion state. Vaccine. 2007;25(27):5102–5114.
- Wagner R, Deml L, Schirmbeck R, et al. Construction, expression, and immunogenicity of chimeric HIV-1 virus-like particles. Virology. 1996;220(1):128–140.
- Griffiths JC, Harris SJ, Layton GT, et al. Hybrid human immunodeficiency virus Gag particles as an antigen carrier system: induction of cytotoxic T-cell and humoral responses by a Gag: V3fusion. J Virol. 1993;67(6):3191–3198.
- Tagliamonte M, Visciano ML, Tornesello ML, et al. HIV-Gag VLPs presenting trimeric HIV-1 gp140 spikes constitutively expressed in stable double transfected insect cell line. Vaccine. 2011;29(31):4913–4922.
- Buonaguro L, Buonaguro FM, Tornesello ML, et al. High efficient production of Pr55(gag) virus-like particles expressing multiple HIV-1 epitopes, including a gp120 protein derived from an Ugandan HIV-1 isolate of subtype A. Antiviral Res. 2001;49(1):35–47.
- Chen CW, Saubi N, Joseph-Munne J. Design concepts of virus-like particle-based HIV-1 vaccines. Front Immunol. 2020;11(573157):1–8.
- Deml L, Schirmbeck R, Reimann J, et al. Recombinant human immunodeficiency Pr55gag virus-like particles presenting chimeric envelope glycoproteins induce cytotoxic T-cells and neutralizing antibodies. Virology. 1997;235(1):26–39.
- Haffar OK, Smithgall MD, Moran PA, et al. HIV-specific humoral and cellular immunity in rabbits vaccinated with recombinant human immunodeficiency virus-like gag-env particles. Virology. 1991;183(2):487–495.
- Buonaguro L, Racioppi L, Tornesello ML, et al. Induction of neutralizing antibodies and cytotoxic T lymphocytes in Balb/c mice immunized with virus-like particles presenting a gp120 molecule from a HIV-1 isolate of clade A. Antiviral Res. 2002;54(3):189–201.
- Rovinski B, Rodrigues L, Cao SX, et al. Induction of HIV type 1 neutralizing and env-CD4 blocking antibodies by immunization with genetically engineered HIV type 1-like particles containing unprocessed gp160 glycoproteins. AIDS Res Hum Retroviruses. 1995;11(10):1187–1195.
- Kang CY, Luo L, Wainberg MA, et al. Development of HIV/AIDS vaccine using chimeric gag-env virus-like particles. Biol Chem. 1999;380(3):353–364.
- Pastori C, Tudor D, Diomede L, et al. Virus like particle based strategy to elicit HIV-protective antibodies to the alpha-helic regions of gp41. Virology. 2012;431(1–2):1–11.
- Zhai Y, Zhong Z, Zariffard M, et al. Bovine papillomavirus-like particles presenting conserved epitopes from membrane-proximal external region of HIV-1 gp41 induced mucosal and systemic antibodies. Vaccine. 2013;31(46):5422–5429.
- Demchuk AM, Patel TR. The biomedical and bioengineering potential of protein nanocompartments. Biotechnol Adv. 2020;41(107547):1–21.
- Sliepen K, Han BW, Bontjer I, et al. Structure and immunogenicity of a stabilized HIV-1 envelope trimer based on a group-M consensus sequence. Nat Commun. 2019;10(1):2355.
- Georgiev IS, Joyce MG, Chen RE, et al. Two-component ferritin nanoparticles for multimerization of diverse trimeric antigens. ACS Infect Dis. 2018;4(5):788–796.
- Ladenstein R, Morgunova E. Second career of a biosynthetic enzyme: lumazine synthase as a virus-like nanoparticle in vaccine development. Biotechnol Rep (Amst). 2020;27(e00494):1–21.
- Jardine J, Julien JP, Menis S, et al. Rational HIV immunogen design to target specific germline B cell receptors. Science. 2013;340(6133):711–716.
- Jardine JG, Ota T, Sok D, et al. HIV-1 VACCINES. Priming a broadly neutralizing antibody response to HIV-1 using a germline-targeting immunogen. Science. 2015;349(6244):156–161.
- Gao Y, Wijewardhana C, Mann JFS. Virus-like particle, liposome, and polymeric particle-based vaccines against HIV-1. Front Immunol. 2018;9:345.
- Zwick MB, Labrijn AF, Wang M, et al. Broadly neutralizing antibodies targeted to the membrane-proximal external region of human immunodeficiency virus type 1 glycoprotein gp41. J Virol. 2001;75(22):10892–10905.
- Muster T, Steindl F, Purtscher M, et al. A conserved neutralizing epitope on gp41 of human immunodeficiency virus type 1. J Virol. 1993;67(11):6642–6647.
- Caillat C, Guilligay D, Sulbaran G, et al. Neutralizing antibodies targeting HIV-1 gp41. Viruses. 2020;12(11):1–19.
- Matyas GR, Wieczorek L, Beck Z, et al. Neutralizing antibodies induced by liposomal HIV-1 glycoprotein 41 peptide simultaneously bind to both the 2F5 or 4E10 epitope and lipid epitopes. AIDS. 2009;23(16):2069–2077.
- Lai RP, Hock M, Radzimanowski J, et al. A fusion intermediate gp41 immunogen elicits neutralizing antibodies to HIV-1. J Biol Chem. 2014;289(43):29912–29926.
- Bomsel M, Tudor D, Drillet AS, et al. Immunization with HIV-1 gp41 subunit virosomes induces mucosal antibodies protecting nonhuman primates against vaginal SHIV challenges. Immunity. 2011;34(2):269–280.
- Leroux-Roels G, Maes C, Clement F, et al. Randomized phase I: safety, immunogenicity and mucosal antiviral activity in young healthy women vaccinated with HIV-1 Gp41 P1 peptide on virosomes. Plos One. 2013;8(2):e55438.
- Dubrovskaya V, Tran K, Ozorowski G, et al. Vaccination with glycan-modified HIV NFL envelope trimer-liposomes elicits broadly neutralizing antibodies to multiple sites of vulnerability. Immunity. 2019;51(5):915–929 e917.
- Pardi N, Hogan MJ, Weissman D. Recent advances in mRNA vaccine technology. Curr Opin Immunol. 2020;65:14–20.
- Pardi N, LaBranche CC, Ferrari G, et al. Characterization of HIV-1 nucleoside-modified mRNA vaccines in rabbits and rhesus macaques. Mol Ther Nucleic Acids. 2019;15:36–47.
- Saunders KO, Pardi N, Parks R, et al. Lipid nanoparticle encapsulated nucleoside-modified mRNA vaccines elicit polyfunctional HIV-1 antibodies comparable to proteins in nonhuman primates. NPJ Vaccines. 2021;6(1):50.
- Lewis GK, Ackerman ME, Scarlatti G, et al. Knowns and unknowns of assaying antibody-dependent cell-mediated cytotoxicity against HIV-1. Front Immunol. 2019;10(1025):1–12.
- Sips M, Krykbaeva M, Diefenbach TJ, et al. Fc receptor-mediated phagocytosis in tissues as a potent mechanism for preventive and therapeutic HIV vaccine strategies. Mucosal Immunol. 2016;9(6):1584–1595.
- Mujib S, Liu J, Rahman A, et al. Comprehensive cross-clade characterization of antibody-mediated recognition, complement-mediated lysis, and cell-mediated cytotoxicity of HIV-1 envelope-specific antibodies toward eradication of the HIV-1 reservoir. J Virol. 2017;91:16.
- Tay MZ, Liu P, Williams LD, et al. Antibody-mediated internalization of infectious HIV-1 virions differs among antibody isotypes and subclasses. PLoS Pathog. 2016;12(8):e1005817.
- von Bredow B, Arias JF, Heyer LN, et al. Comparison of antibody-dependent cell-mediated cytotoxicity and virus neutralization by HIV-1 env-specific monoclonal antibodies. J Virol. 2016;90(13):6127–6139.
- Hessell AJ, Jaworski JP, Epson E, et al. Early short-term treatment with neutralizing human monoclonal antibodies halts SHIV infection in infant macaques. Nat Med. 2016;22(4):362–368.
- Liu J, Ghneim K, Sok D, et al. Antibody-mediated protection against SHIV challenge includes systemic clearance of distal virus. Science. 2016;353(6303):1045–1049.
- Parsons MS, Lee WS, Kristensen AB, et al. Fc-dependent functions are redundant to efficacy of anti-HIV antibody PGT121 in macaques. J Clin Invest. 2019;129(1):182–191.
- Asokan M, Dias J, Liu C, et al. Fc-mediated effector function contributes to the in vivo antiviral effect of an HIV neutralizing antibody. Proc Natl Acad Sci U S A. 2020;117(31):18754–18763.
- Wang P, Gajjar MR, Yu J, et al. Quantifying the contribution of Fc-mediated effector functions to the antiviral activity of anti-HIV-1 IgG1 antibodies in vivo. Proc Natl Acad Sci U S A. 2020;117(30):18002–18009.
- Yang L, Song Y, Li X, et al. HIV-1 virus-like particles produced by stably transfected Drosophila S2 cells: a desirable vaccine component. J Virol. 2012;86(14):7662–7676.
- Zhao C, Ao Z, Yao X. Current advances in virus-like particles as a vaccination approach against HIV infection. Vaccines (Basel). 2016;4(1):1–20.
- Franco D, Liu W, Gardiner DF, et al. CD40L-containing virus-like particle as a candidate HIV-1 vaccine targeting dendritic cells. J Acquir Immune Defic Syndr. 2011;56(5):393–400.
- Zhang R, Zhang S, Li M, et al. Incorporation of CD40 ligand into SHIV virus-like particles (VLP) enhances SHIV-VLP-induced dendritic cell activation and boosts immune responses against HIV. Vaccine. 2010;28(31):5114–5127.
- Kwa S, Lai L, Gangadhara S, et al. CD40L-adjuvanted DNA/modified vaccinia virus Ankara simian immunodeficiency virus SIV239 vaccine enhances SIV-specific humoral and cellular immunity and improves protection against a heterologous SIVE660 mucosal challenge. J Virol. 2014;88(17):9579–9589.
- Gangadhara S, Kwon YM, Jeeva S, et al. Vaccination with combination DNA and virus-like particles enhances humoral and cellular immune responses upon boost with recombinant modified vaccinia virus ankara expressing human immunodeficiency virus envelope proteins. Vaccines (Basel). 2017;5(4):1–14.
- Huang X, Zhu Q, Huang X, et al. In vivo electroporation in DNA-VLP prime-boost preferentially enhances HIV-1 envelope-specific IgG2a, neutralizing antibody and CD8 T cell responses. Vaccine. 2017;35(16):2042–2051.
- Poteet E, Lewis P, Li F, et al. A novel prime and boost regimen of HIV virus-like particles with TLR4 adjuvant MPLA induces Th1 oriented immune responses against HIV. PLoS One. 2015;10(8):e0136862.
- Iyer SS, Gangadhara S, Victor B, et al. Virus-like particles displaying trimeric simian immunodeficiency virus (SIV) envelope gp160 enhance the breadth of DNA/modified vaccinia virus ankara SIV vaccine-induced antibody responses in rhesus macaques. J Virol. 2016;90(19):8842–8854.
- Pillay S, Shephard EG, Meyers AE, et al. HIV-1 subtype C chimaeric VLPs boost cellular immune responses in mice. J Immune Based Ther Vaccines. 2010;8(7):1–6.
- Moyo N, Wee EG, Korber B, et al. Tetravalent immunogen assembled from conserved regions of HIV-1 and delivered as mRNA Demonstrates potent preclinical T-cell immunogenicity and breadth. Vaccines (Basel). 2020;8(3):1–10.
- Moyo N, Vogel AB, Buus S, et al. Efficient induction of T cells against conserved HIV-1 regions by Mosaic vaccines delivered as self-amplifying mRNA. Mol Ther Methods Clin Dev. 2019;12:32–46.
- Ahmad N, Khan MA, Owais M. Liposome mediated antigen delivery leads to induction of CD8+ T lymphocyte and antibody responses against the V3 loop region of HIV gp120. Cell Immunol. 2001;210(1):49–55.
- Steers NJ, Peachman KK, McClain S, et al. Liposome-encapsulated HIV-1 Gag p24 containing lipid A induces effector CD4+ T-cells, memory CD8+ T-cells, and pro-inflammatory cytokines. Vaccine. 2009;27(49):6939–6949.
- Hanke T. Aiming for protective T-cell responses: a focus on the first generation conserved-region HIVconsv vaccines in preventive and therapeutic clinical trials. Expert Rev Vaccines. 2019;18(10):1029–1041.
- Rao M, Alving CR. Adjuvants for HIV vaccines. Curr Opin HIV AIDS. 2016;11(6):585–592.
- Ratnapriya S, Perez-Greene E, Schifanella L, et al. Adjuvant-mediated enhancement of the immune response to HIV vaccines. FEBS J. 2021:1–18.
- Alving CR, Peachman KK, Rao M, et al. Adjuvants for human vaccines. Curr Opin Immunol. 2012;24(3):310–315.
- Kasturi SP, Rasheed MAU, Havenar-Daughton C, et al. 3M-052, a synthetic TLR-7/8 agonist, induces durable HIV-1 envelope-specific plasma cells and humoral immunity in nonhuman primates. Sci Immunol. 2020;5:48.