ABSTRACT
Introduction
The discovery of neoantigens as mutated proteins specifically expressed in tumor cells but not in normal cells has led to improved cancer vaccines. Targeting neoantigens can induce anti-tumor T-cell responses to destroy tumors without damaging healthy cells. Extensive advances in genome sequencing technology and bioinformatics analysis have made it possible to discover and design effective neoantigens for use in therapeutic cancer vaccines. Neoantigens-based therapeutic personalized vaccines have shown promising results in cancer immunotherapy.
Areas covered
We discuss the types of cancer neoantigens that can be recognized by the immune system in this review. We also summarize the detection, identification, and design of neoantigens and their appliction in developing cancer vaccines. Finally, clinical trials of neoantigen-based vaccines, their advantages, and their limitations are reviewed. From 2015 to 2020, the authors conducted a literature search of controlled randomized trials and laboratory investigations that that focused on neoantigens, their use in the design of various types of cancer vaccines.
Expert opinion
Neoantigens are cancer cell-specific antigens, which their expression leads to the immune stimulation against tumor cells. The identification and delivery of specific neoantigens to antigen-presenting cells (APCs) with the help of anti-cancer vaccines promise novel and more effective cancer treatments.
1. Introduction
Cancer is now regarded as a genetic disease caused by the accumulation of mutations in cellular DNA. These mutations can alter signal transduction pathways that control apoptosis and cell proliferation, resulting in cellular immortality and tumor development [Citation1]. A range of non-synonymous genetic alterations, including single-nucleotide polymorphisms, sequence deletions and insertions, frameshift mutations, gene fusions, and structural variants, results in changes in the amino acid sequences of the encoded proteins, and these can then function as cancer neoantigens [Citation2]. Neoantigens are ideal therapeutic targets because they are cancer-specific and have no expression in normal cells. neoantigens have the potential to minimize the autoimmunity risk and maximize the therapeutic specificity as well as overcome immune tolerance [Citation3,Citation4]. Therefore, there is a crucial need to identify a wider range of neoantigens for more effective vaccines, especially for cancer immunotherapy.
Cancer immunotherapy can be either passive or active, but both approaches are based on stimulating the host immune system to fight against cancer cells (). Tumor-targeted monoclonal antibodies, and adoptive cell transfer, such as T cell therapies that enhance the intrinsic antineoplastic activity, are two important types of passive immunotherapy [Citation5]. Passive immunotherapy relies on the recognition of cancer cell antigens by administered agents. For example, there are increasing clinical trials using chimeric antigen receptor T (CAR-T) cell immunotherapy targeting tumor-associated antigens (TAAs) over-expressed on cancer cells relative to normal cells. Although CAR-T cell therapies have shown outstanding results in the treatment of some cancer types, such as acute lymphocytic leukemia, it is still challenging for the effective treatment of solid tumors. This is due to the immunosuppressive tumor microenvironment and the difficulty of identifying a specific TAA for many solid tumors [Citation6].
Figure 1. Types of cancer immunotherapy. immunotherapies are divided into passive and active approaches [Citation9].
![Figure 1. Types of cancer immunotherapy. immunotherapies are divided into passive and active approaches [Citation9].](/cms/asset/335b92a3-cd4f-46e1-be89-cc478584f712/ierv_a_1951246_f0001_oc.jpg)
Figure 2. Recognition of tumor neoantigens by CD8+ T cells. receptors on CD8 + T cells recognize neoantigens on the surface of tumor cells, which are presented by the major histocompatibility complex class I (MHCI). CD8 is a co-receptor that promotes antigen-receptor binding.
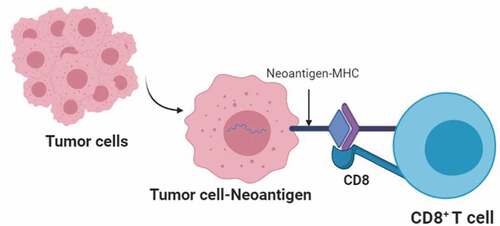
On the contrary, active immunotherapy is designed to engage the host immune system to attack tumor cells within the body [Citation7]. This approach can overcome immunosuppression and result in complete tumor clearance by the host immune system [Citation8]. Active immunotherapy includes agents called immune checkpoint inhibitors (ICIs) that can target the checkpoint blockade that prevents preexisting host T-cells from attacking the tumor [Citation9] and a variety of anti-cancer vaccines. Cancer vaccines appear to produce more effective and specific immune responses to fight cancer in comparison with other cancer immunotherapy types [Citation10]. Cancer vaccines rely on the recognition of highly specific tumor antigens; therefore, the identification of cancer neoantigens is critical for effective anti-tumor immunity in cancer patients [Citation11,Citation12].
Recent advances in sequencing technology and an explosion in bioinformatics algorithms have led to identifying specific tumor neoantigens that can be used in personalized therapeutic vaccines to treat cancer. In this review we focused on neoantigens as the main target of cancer vaccines, their design methods, and neoantigen utilizing in the cancer vaccine formulation. We also discussed the advantages, limitations, and prospects for this therapeutic revolution in cancer therapy.
2. An overview of neoantigens
The main feature of neoantigens is their specific expression in tumor cells [Citation13], and they can function as tumor-specific antigens (TSAs) presented by the major histocompatibility complex (MHC) expressed on cell membranes (). The immune system is stimulated by the expression of TSAs against tumor cells [Citation14]. Significant progress has been achieved in the finding of tumor antigens in order to produce cancer vaccines in recent years [Citation1,Citation15]. Tumor antigens are classified into several types, which are summarized in .
Table 1. Classification of tumor antigens.
In principle, many cancer antigens could be used to design anti-cancer vaccines. However, there is a practical limit in their number because most cancer antigens are also found in normal tissues. TAAs are also expressed in normal cells and are considered to be self-antigens. T cells that bind to TAAs with high affinity are often removed from the immune repertoire because they recognize self-antigens by peripheral and central tolerance mechanisms; therefore, a cancer vaccine based on TAAs must be powerful enough to break tolerance [Citation31]. For this reason, tumor-specific antigens (TSAs) have become more popular in the design of cancer vaccines. TSAs are more specific antigens that are expressed in various stages of tumor cells isolated from different individual patients. TSAs include proteins resulting from mutations in tumor cells or derived from viral antigens in virus-associated tumors [Citation14,Citation32]. The peptides derived from TSAs are presented by HLA class I molecules on the surface of tumor cell and are subsequently detected by the neoantigen-specific TCRs [Citation33,Citation34]. In general, the targeting of TSAs does not induce autoimmunity. Neoantigens encoded by oncogenic driver mutations are referred to as ‘shared neoantigens’ since them detectable in a wide range of individuals and tumor types. The majority of neoantigens (private neoantigens) are specific to individual patients’ malignancies, necessitating the development of personalized vaccine preparation. As a result, neoantigens are regarded as ideal targets for therapeutic cancer vaccines and T cell-based cancer immunotherapy [Citation35].
These neoantigens can be classified into several categories: genomic variants (SNPa as well as small deletions and insertions); genomic gene fusions; transcriptomic alternative splicing; transcriptomic RNA editing; microRNA regulation; and transcriptomic alternative splicing [Citation36–39]. Compared with other kinds of immunotherapy, neoantigen-based vaccines induce a more specific immune response and can demonstrate a stable therapeutic response. In general, cancer vaccines based on neoantigen are relatively safe, reliably manufactured, and capable of inducing T cell responses [Citation2]. To use tumor-specific neoantigens in immunotherapy, they must first be identified at a molecular scale.
3. Identification of neoantigens: past and present
Identification of neoantigens is challenging because out of many possible mutations occurring in cancer cells, only a small percentage can produce neoantigens, which are recognized by T cells and induce an immune response [Citation40–42]. The strategies for the discovery of neoantigens include several key steps. The basic step is to compare the genome sequences of normal and tumor cells [Citation43]. Based on the applied strategy for filtering the candidate neoantigens, these tools are classified into two classes: classical approaches and next-generation sequencing techniques [Citation44,Citation45].
In classical approaches, majority of the neoantigens have been identified by screening of cDNA libraries. cDNA library screening is used to screen Tcell–reactive neoepitopes. In this method, the cDNA library and the relevant MHC molecules are up-regulated in cell lines, which are then co-cultured with T cells to identify the antigens that could induce T cell activation, as measured by cytokine secretion. Although the classical approach has led to the discovery of several neoantigens, it has low-throughput and is labor-intensive. CDK4 [Citation46] and Multiple Myeloma 1 (MUM1) [Citation47] were identified as the first neoantigens in melanoma using a cDNA library screening approach in 1995. Since melanoma tumors have high mutation rate, most of the neoantigens discovered by classical methods were in melanomas compared to other cancer types. However, some other mutations, such as HLA-A2 in renal cancer [Citation48], and the EF2 mutation in lung cancer [Citation49], were also detected by the cDNA library screening method.
The quick advancement of high-throughput sequencing technology, such as whole-exon and whole-genome sequencing, has led to this approach becoming less expensive and more convenient [Citation50]. Moreover, bioinformatics algorithms have also made considerable progress in the last two decades. Recently, whole-exome sequencing technology has been used to detect non-synonymous mutations in tumors. In silico analysis of these altered genes was used to anticipate possible high-affinity epitopes that may bind to MHC molecules [Citation51,Citation52]. Researchers have used in silico analysis to illuminate the role of MHC class I complexes in antigen presentation, and then they cloned and expressed the genes using molecular biology methods [Citation53] [Citation54]. These in silico approaches employed a series of algorithms to filter by the mutations identified in the sequencing data in order to predict those that were likely to lead to the neoantigens generation [Citation55,Citation56].
In next-generation sequencing approaches, the neoantigen identification process typically starts with the analysis of a routine clinical biopsy sample. Next, all the somatic non-synonymous mutations (NSM) within the tumor sample are mapped using next-generation sequencing (NGS). In this manner, the precise DNA mutations in the tumor cells that did not occur in healthy cells could be identified [Citation57,Citation58]. Then the quality of the sequencing read data must be investigated. Variant-calling software algorithms allow the alignment of normal, and tumor read sequences to sequences from the reference genome. To decrease false positives, computational analysis pipelines frequently utilize several variant callers and choose those somatic variations found by several independent variant callers. A personalized immunotherapy vaccine is manufactured in the final step, containing the relevant neoantigens, and administered back to the patient for inducing a specific T cell response () [Citation59,Citation60].
Figure 3. Overview of neoantigen identification. whole exome sequencing can be used to identify tumor-specific NSM (non-synonymous mutations). once NSMs are identified, the strategies can be used to select a list of candidate neoantigens to be assessed for immunogenicity. finally, the immunogenicity of the selected candidate peptides is evaluated using different immunological screening assays, and the personalized neoantigen cancer vaccine can be prepared.
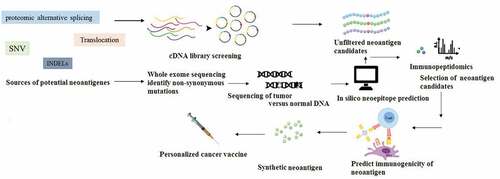
4. Novel approaches in detection, prediction, and integration of neoantigens
As mentioned before, there are numerous non-synonymous somatic mutations, which are specific in tumors. The notable points are their binding affinity to HLA molecules and their consequent immunogenicity [Citation61]. In this section the key steps of neoantigen prediction via NGS and novel bioinformatics approaches are discussed.
4.1. Detection of variant and mutations in tumor cells
Identification of specific mutated proteins in tumor cells is the first step of neoantigen detection. MUTECT, as a part of the Genome Analysis Toolkit, is a powerful tool for the detection of variants [Citation62]. MUTECT works by analyzing whole-genome, whole-exome, and RNA sequencing data. EBCall and VarScan are other servers, which are used for mutated protein detection [Citation63]. Read misalignments and false-positive mutations are the main challenges encountered in this step, which can be solved by increasing the accuracy of the algorithms employed [Citation64]
4.2. HLA typing
The next step is HLA typing that is carried out using serologic methods. Several programs that can predict HLA type using RNA sequencing data are being developed because of the growth of NGS methodology. Some new programs such as Optitype [Citation65] and Polysolver [Citation66] are replacements for older software tools like Seq2HLA [Citation67] and HLAminer [Citation68], which now have higher accuracy and less errors. To decide HLA type, these modern HLA typing algorithms compare RNAseq or whole genome sequencing data with reported reference sequences, choosing the alignments with the fewest mismatches [Citation67].
4.3. Affinity of MHC binding
For prediction of neoantigen–MHC binding affinity there are several tools and methods available. However, most of them use sequence-based methods rather than structure-based methods. The first methods emphasize protein sequencing, while in structure-based methods, the three-dimensional structure of the protein is considered [Citation61]. Initially, these methods relied on linear techniques to measure each particular amino acid binding affinity in theoretically possible binding locations. There are now more sophisticated nonlinear approaches employing higher machine learning. Nowadays NetMHC is a popular tool for prediction of allele-specific epitopes [Citation69]. NetMHCpan is another tool for determination of affinity of MHC binding using panspecific machine learning methods [Citation70].
4.4. Integration of all the steps
There are powerful tools for this stage, one of the most well known of which is pVAC-Seq (personalized variant antigens by cancer sequencing), introduced by the Hundal team in 2016 [Citation38]. In this program, a list of somatic mutations and the patient haplotype are used as input data. The authors constructed a variant list annotated with amino acid modifications and transcript sequences using a genome modeling method for alignment and variant calling. CloudNeo, TIminer and INTEGRAT-Neo are alternative pipelines. These pipelines all carry out similar tasks, but the procedures and outcomes differ. It is critical that users test several pipelines, select the best one for their needs, and be aware of possible pitfalls such as neoantigen false positive and false negative predictions [Citation61].
MuPeXI is another novel mutant peptide extractor and can detect neoantigens. A file containing somatic mutations in cancer cells is the MuPeXI input data, as well as a list of HLA types and a profile of gene expression. The output is a table containing a list of all mutated peptides plus annotations including HLA types, and homology to other peptides. Neo-peptides are ordered based on their potential immunogenicity [Citation71].
The combination of NGS technology and in silico approaches can identify candidate-mutated peptides, which can then be synthesized and screened to detect neoantigens. These methods are extremely effective and can identify neoantigens where the previous cDNA library screening approach has failed to discover [Citation53,Citation54,Citation72]. Mutated GART, CIRH1A, RND3, ASAP1, RPS12, TNIK, LEMD2, and ZC3H18 are examples of T cell neoantigen epitopes that have been identified using NGS approaches [Citation72].
5. Potential application of neoantigens in anti-tumor vaccines
Currently, neoantigens have the potential to be used in cancer immunotherapy, in particular for anti-tumor vaccine design. Anti-tumor vaccines targeting neoantigens are designed to induce T cell-based immune responses in patients with cancer by amplifying and presenting the individual neoantigens [Citation73]. Differences in the expression or non-expression of targeted neoantigens determine the efficacy and effectiveness of cancer vaccines. Therapeutic vaccines targeting tumor-specific neoantigens increase both CD4+ and CD8+ tumor-specific T-cell responses and sensitize naive T-cells to become tumor-specific [Citation74]. The main advantages of utilizing neoantigens in cancer vaccines are that they are less likely to induce autoimmunity and have the possibility for designing personalized vaccines [Citation75–77]. Moreover, the genome-based identification of immunogenic neoantigens using NGS and computational analysis can be coupled with T-cell reactivity assays or mass spectrometry to maximize the immunogenicity of the vaccines [Citation78]. Firstly neoantigen vaccines were studied in mouse models, and then clinical trials in glioblastoma and melanoma patients were carried out to demonstrate the safety and feasibility of these vaccines [Citation79–81]. Previous studies have confirmed the beneficial effects of neoantigen-based vaccines in melanoma treatment [Citation82,Citation83]. Apart from melanoma, the potential benefits of neoantigen-based vaccines have also been confirmed in the treatment of human glioblastoma. The number of neoantigen-specific CD4+ and CD8+ cells was increased in glioblastoma patients who received multi-epitope neoantigen vaccination. This finding suggested that neoantigen-based vaccines could be feasible for glioblastoma [Citation84,Citation85].
For designing effective cancer vaccines, multiple factors have to be considered, including formulation, selection of appropriate delivery system as well as immune adjuvants, due to their potential strongly effect on the immunogenicity and vaccination efficacy [Citation86,Citation87]. Accordingly, neoantigen vaccines can be formulated based on genetic sequences (DNA or RNA vaccines), synthetic long peptide (SLP) vaccines, virus-based vaccines, or dendritic cell vaccines. In these anticancer vaccines, the designed epitopes, or their corresponding DNA or RNA sequences are delivered into cancer cells or into dendritic cells using appropriate carriers () [Citation88,Citation89].
Table 2. Clinical trials of different anti-cancer vaccine platforms [Citation92].
There are significant benefits to using neoantigens for cancer vaccines. Because these epitopes are considered as non-self, central tolerance mechanisms will not restrict the affinity and quantity of T cells that recognize non-self epitopes, as is the case with vaccines targeting self-epitopes. Off-target autoimmune adverse effects will also be decreased because the immune responses are restricted to the tumor. Because of these potential advantages, much research has been done to see if targeting neoantigens might improve the effectiveness of anticancer vaccines. Although the use of neoantigens in cancer vaccines seems promising, unsolved challenges still remain. Challenges such as the rarity of mutations between individual patients, the diversity of HLAs and the T cell repertoire, underline the need to personalize anti-cancer vaccines. As the practicality of this method has become evident, the issue today is whether the tremendous work necessary to build personalized vaccines against neoantigens results in a considerably better outcome than self-antigen vaccines. The development of neoantigen vaccines and their use in early stage clinical trials has so far been promising.
6. Cancer vaccines
6.1. Genetic approaches in cancer vaccines
The use of RNA or DNA sequences encoding the amino acids of the identified neoantigen is one approach to induce APCs to present the designed neoantigen and induce an immune response. DNA-based vaccines are DNA constructs that have the ability to encode one or more neoantigens and can be adminstered either as a naked DNA sequence or contained within a vector. The vectors used mainly comprise nonpathogenic viruses, yeast cells, or bacterial vectors. The aim of a DNA-based vaccine is to stimulate the production of neoantigens by the cells in an immunostimulatory environment, eliciting a tumor-specific immune response [Citation90]. The use of the cytomegalovirus immediate-early promoter and optimizing the codon usage, can both improve the expression level of the neoantigens encoded by the plasmid [Citation91,Citation92]. Numerous evidence have indicated that immune responses could be induced by the intramuscular, intradermal, or intravenous injection of naked DNA. DNA vaccines also are injected directly into the lymph nodes to increase antigen uptake by antigen-presenting cells and promote local inflammatory signals [Citation93,Citation94]. These types of neoantigen vaccines are considered flexible platforms because they can be easily synthesized, and the costs of production are comparatively modest.
Moreover, synthetic DNA-based vaccines can target multiple neoantigens at the same time. These DNA platforms may have unique advantages to prime T cell activation as neoantigen-based vaccines. Tumor neoantigens encoded by DNA vaccines can generate potent tumor-specific cytotoxic CD8 + T-cell responses [Citation95].
In contrast, RNA molecules coding for neoantigen peptides can also be used as a vaccine platform to target cancer cells in different approach. Due to the general instability of RNA molecules, RNA-based vaccines can cause fewer side effects, show a lower risk of triggering the autoimmune disease, and have a lower probability of integration into the host cell genome compared to DNA-based vaccines [Citation96,Citation97]. Although only a few RNA vaccines have been tested in phase I and II clinical trials, for the first time, Sahin et al. employed a tailored RNA vaccination to treat melanoma. Thirteen patients received RNA vaccination combined with anti-PD-1 checkpoint blockade therapy and showed increased numbers of neoantigen-specific T cells [Citation83]. PD-1 (programmed cell death-1) is an immune checkpoint protein. The expression of PD-1 on the surface of activated T cells, and the expression of its ligands, PD-L1 and PD-L2 on dendritic cells or macrophages, both inhibit the development of T cell responses against tumor cells [Citation98].
Some coronavirus vaccines were recently designed based on the identification of SARS-CoV-2 spike (S) protein, which binds to its cellular receptor, angiotensin-converting enzyme 2. These are basically divided into two types: 1) vaccines that disrupt the expression of S protein in the virus; and 2) integrated nucleotide (RNA or DNA) sequences to synthesize the S protein within host cells [Citation99]. These RNA-based vaccines were successful, and were approved after phase III clinical trials. Due to the instability of the mRNA encoding the virus S protein, the RNA sequences must be loaded within a protective capsule. For this purpose, lipid nanoparticles such as liposomes have been used that can protect mRNA from destruction by physical, chemical or biological agents [Citation100]. Employing a similar strategy to deliver mRNA encoding neoantigens could be a promising approach for the development of RNA-based anti-cancer vaccines [Citation101].
6.2. Peptide and protein-based cancer vaccines
The immune response of T cells is based on the recognition of small antigenic peptide sequences, known as epitopes. Peptide-based vaccines are based on the laboratory synthesis of these epitope peptide sequences to induce an immune response. The first attempt to use a synthetic gp100 epitope in a vaccine for melanoma treatment was unsatisfactory [Citation102].
Recent research has demonstrated that the use of synthesized long peptides (SLPs) can induce a more potent immune response. SLPs are peptides ranging from 20–35 amino acids in length, derived from tumor-specific mutant antigens (TSMAs), with the potential to trigger an immune response [Citation103]. Unlike small peptides (8–12 amino acids), SLPs are only presented by professional APCs, resulting in a more specific immune response. On the other hand, SLPs have shown more effective results in inducing an immune response compared to whole proteins. Unlike proteins, these peptides do not require any folding and are more efficiently taken up by endocytosis [Citation104].
A practical strategy to improve the uptake of SLPs by APCs and to increase activation is to integrate ligands for pattern-recognition receptors (PRRs), such as C-type lectin (CLR), toll-like receptors (TLR), or NOD-like-receptors. These receptors are over-expressed on APCs and their purpose is to stimulate the immune response toward infectious pathogens. In general, SLP-based vaccines are easy to manufacture, and the storage conditions do not require expensive equipment. The T cell immune responses can be improved by the addition of powerful pro-inflammatory adjuvants. One successful clinical trial [Citation82,Citation105], tested an SLP vaccine in six melanoma patients post-surgery. The results showed that there was no tumor recurrence after 25 months. However, peptide vaccines only have limited efficacy for inhibiting tumor growth, and even less for causing tumor regression, and multiple peptides need to be used at the same time to improve the efficacy, as each peptide targets one (or a few) TAA epitopes. Nevertheless, vaccines based on proteins or peptides are less expensive compared to other kinds of vaccines [Citation103].
6.3. Anti-cancer vaccines based on dendritic cells
Dendritic cells (DC) are considered as the most effective type of APCs. Antigens are taken up by DCs and are processed, presented in MHC molecules on the cell surfaces, stimulating the T cells’ response. However, Exogenous vaccine antigens must gain access to the endogenous MHCI presentation pathway of DCs to activate CD8+ cytotoxic T cell responses, a process known as antigen cross-presentation. The professional APCs, DCs, play a primary role in bridging the gap between adaptive and innate immunity. DCs are noted for their cross-presentation ability since they digest and present foreign antigens on MHCI molecules considerably more effectively than other phagocytes. The efficacy of CD8 + T cell priming by DCs, known as cross-priming, is determined by both antigen cross-presentation efficiency (the amount of MHCI/peptide complex on the cell surface) and DC maturation (expression levels of co-stimulatory molecules and cytokines). Cross-presentation is critical for activating T cell responses against tumor antigens. However, it is still unclear how exogenous antigens are processed inside DCs and presented on MHCI to CD8 + T lymphocytes [Citation106].
Many types of cancer immunotherapy target DCs, either directly or indirectly, to induce antigen-specific immune responses. Different DC subsets have been shown to govern the proliferation of distinct T-cell populations, and control different types of immune response in vivo. In one animal study, CD8+ CD205+ DCs presented antigens through both MHC class I and MHC class II molecules, which was considered crucial for highly effective induction of an immune response [Citation107–109]. DC vaccines are considered to be an exciting type of immunotherapy. Their advantages include the potential to trigger strong immune responses, low risk of toxicity, and the ability to activate other cell types like natural killer (NK) cells [Citation110]. Preclinical research has recently been conducted and has revealed the efficacy and viability of neoantigen-targeted cancer vaccines in animal models of various cancers such as colon carcinoma, glioma, and melanoma [Citation76,Citation111,Citation112]. An investigation in a mouse model of glioma indicated that a neopeptide vaccine containing the IDH1 (R132H) p123–142 mutated region led to an effective anti-tumor immune response [Citation34,Citation82]. The encouraging results from preclinical studies have motivated the development of clinical trials using neoantigen-based vaccines. The first reported phase I clinical trial using a DC-based vaccine, and showed it triggered a variety of neoantigen-specific T cells in three patients with advanced melanoma [Citation113].
The advantages of DC vaccines include the induction of polyclonal immune responses against multiple antigens and activation of both the adaptive and innate immune responses. However, there are also disadvantages, such as being time-intensive, having a costly production process, and poor immunogenicity, which have limited their broad application in cancer treatment [Citation114].
6.4. Anti-cancer vaccines based on viral vectors
Utilizing of viral vectors is another approach that has been employed to prepare cancer vaccines. Replication-defective poxviruses, adenoviruses, and alphaviruses are the most commonly used viral vectors in vaccines. The delivery and presentation of neoantigens is the main goal of viral vector vaccines. Since the immune system is inherently on guard against viruses, their application as vaccines can trigger a dual adaptive and innate immune response [Citation115]. Nevertheless, the immune system can neutralize the vector itself and can therefore limit the possibility of repeat vaccination. Virus-based prime-boost regimens with heterologous viral backbones expressing tumor antigens could overcome this limitation. A successful example of the use of a viral vector in a cancer vaccine was in patients with prostate cancer. This viral vector expressed the TAA PSA plus the co-stimulatory molecule B7–1 (CD80) [Citation116]. The results were promising in late-phase clinical trials. Despite the success of viral vaccines in clinical trials, they could have mutagenic potential due to insertion into the genome. Moreover, viral vaccines require an additional boosting strategy to enhance immunogenicity, which remains challenging [Citation117].
7. Induction of cell death combined with cancer vaccine approaches
When any anti-cancer therapy can cause an immunogenic type of cancer cell death (ICD), it is likely to be more effective than an approach that causes non-immunogenic cell death. As a result, it is critical to use anti-cancer approaches that stimulate ICD and thereby trigger an anti-tumor immune response, resulting in the simultaneous destruction of the tumor, while at the same time stimulating anti-cancer immunity.
Necroptosis is an alternative mode of programmed cell death with features of both apoptosis and necrosis. In a similar manner to necrosis, it is considered to be pro-inflammatory, but is more effective in triggering the maturation of dendritic cells, cross-priming of cytotoxic T cells, and the secretion of IFN-gamma. This leads to better sensitization to tumor antigens contained within the necroptotic cancer cells [Citation118]. Aaes et al. developed a method to produce necroptotic cells using direct dimerization of FADD combined with inducible expression of RIPK3 (receptor interacting protein kinase-3). These necroptotic cells enabled successful prophylactic vaccination of mice against CT26 colorectal tumors [Citation118]. In another study by Turubanova et al. they used photodynamic therapy (PDT) mediated by two different photosensitizers, Photosens (PS) and Photodithazine (PD) against murine glioma GL261 and fibrosarcoma MCA205 cells. The type of cell death induced was analyzed by flow cytometry. The results indicated that PDT with PS or PD were novel ICD inducers, and could be used as a potential approach to prepare cancer vaccines [Citation119].
Dying cells trigger adaptive immunity by delivering antigens and inflammatory stimuli at the same time to DCs that then stimulate CD8(+) T cells via antigen cross-priming. Yatim et al. created in vitro models of apoptosis and necroptosis, in which dying cells were generated by receptor-interacting protein kinase-3 and caspase-8 dimerization, respectively, to determine how different types of programmed cell death affected immunity. According to their findings, inflammatory mediators releasing by dying cells, like damage-associated molecular patterns, was unnecessary for CD8(+) T cell cross-priming. Instead, robust cross-priming required RIPK1 signaling and nuclear factor κB (NF-κB)-induced transcription within dying cells. The priming efficiency and tumor immunity were decreased when NF-κB signaling was decoupled from inflammatory apoptosis or necroptosis. These findings showed that adaptive immunity was orchestrated by synchronized inflammatory and cell death signaling pathways in dying cells [Citation120].
Anti-tumor immunity is activated by inducing immunogenic necroptosis or apoptosis in cancer cells, but resistance to these cell death modalities is common. As a result, discovering alternative ways to destroy tumor cells is important. Ferroptosis was recently discovered as a novel, iron-dependent mechanism of controlled cell death, but it is unclear if ferroptotic cancer cells are highly immunogenic [Citation120]. In a study by Efimova et al, RAS-selective lethal 3 was used to cause ferroptotic cell death in murine fibrosarcoma MCA205 or glioma GL261 cells. The ferroptosis was studied using flow cytometry, atomic force microscopy, and confocal microscopy. Efimova and colleagues showed inhibition of glutathione peroxidase 4 could also cause ferroptosis in cancer cells, as demonstrated by confocal and atomic force microscopy and use of inhibitor. For the first time, they showed the immunogenicity of ferroptosis as a cell death mechanism, both in vitro and in vivo. These findings will prepare the way for the development of new cancer immunotherapy strategies focused on ferroptosis, necroptosis, and apoptosis as types of cancer cell death, broadening the existing understanding of immunogenic cell death [Citation120].
8. Pathways toward the use of neoantigen vaccines in patients
The different types of cancer vaccines and how they work to induce anti-tumor T cell responses in the human body are numerous. Processes include cancer antigen presentation, T cell priming and activation, recognition of cancer cells by T cells, and several immune effector mechanisms to eliminate tumors [Citation121]. Through the use of an adjuvant and antigen to activate both innate and adaptive response, anticancer vaccines can stimulate both innate and adaptive immunity. Pattern recognition receptors, such as Toll-like receptors, trigger nonspecific innate immune responses by recognizing and responding to pathogen- or damage-associated molecular patterns. Activation of the transcription factor nuclear factor kB (NF-kB), stimulation of chemokine and cytokine production, and lymphocyte activation are the results of engagement of Toll-like receptors [Citation122]. There are several mechanisms for inducing a compatible antitumor response by cancer vaccines, which can be categorized as follows:
APCs can present and recognize immunogenic tumor antigens;
APCs can undergo maturation for efficient processing and presentation of antigens;
APCs can induce cytokines and expression of T cell costimulatory signals;
APCs can interact with the adaptive immune system to prime and activate CD8 + T cells;
The localization of these separate elements within tumors is shown in [Citation123].
Figure 4. The mechanisms of vaccines and neoantigens in the T cell immune response. vaccines (DNA, RNA, peptide, DC-based, or viral vaccines) are administered into the human body remote from the tumor. antigens are presented by APCs and recognized by T cells to accomplish priming, expansion and circulation of effector T cells that then attack the tumor. in this step, the T cells are divided into effector T cells which circulate in the blood and destroy the tumor, and memory T cells, which remain able to proliferate again if they encounter their cognate antigen.
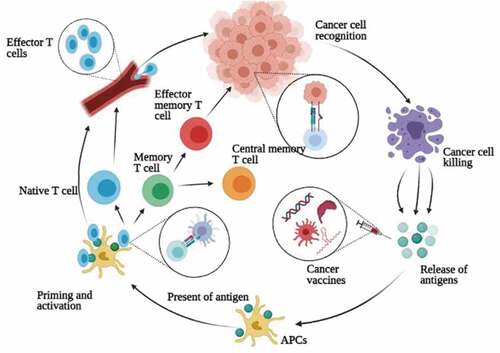
Cancer vaccines can produce long-lasting immunological memory through these mechanisms, and can prevent tumor growth over the long-term, and prevent relapse and metastasis. Long-lived memory T cell responses have been demonstrated in preclinical experiments, which can revive effector T cells after some time, which can still destroy tumor cells.
9. Combination approaches involving cancer vaccine platforms
Each cancer vaccine platform has its own advantages and disadvantages. However, the various challenges and disadvantages have tended to limit their use as a definitive type of cancer immunotherapy. A combination of two different therapies could be a more effective way to overcome these challenges to achieve a better outcome. The ability of tumor cells to interfere with or suppress all specific immune responses has limited the use of cancer vaccines in patients [Citation124].
In one study by Yadav and colleague [Citation78], mutated, REPS1, ADPGK, and DPAGT1 peptide vaccines were developed that could inhibit the tumors growth. However, after administering these vaccines, the neoantigen-specific T cells also showed high levels expression of TIM3 and PD-1 that are negative regulators of the immune response. This negative feedback loop was probably mediated by the production of interferon-gamma (IFNγ) from activated CD8 T cells [Citation125]. IFNγ is the primary regulator of PD-L1 expression, which is a functional inhibitor of cancer vaccines [Citation126]. To solve these challenges, a combination of checkpoint inhibitor therapy, using monoclonal antibodies like anti-PD-1 and anti-PD-L1 combined with cancer vaccines has been investigated [Citation127].
Combination approaches including neoantigen vaccination plus adoptive T cell treatment have also been investigated to enhance the anti-tumor immune response [Citation128]. Matthias team suggested that mutation-specific TCRs introduced into adoptive T cells might give an effective anti-tumor response under proper conditions [Citation129].
Traditional tumor therapy approaches, such as radiotherapy, chemotherapy, and even photodynamic therapy (PDT) can improve the effectiveness of neoantigen vaccines [Citation14,Citation130]. Chemotherapy, radiotherapy, and PDT can induce immunogenic tumor cell death involving the release of additional amounts of tumor antigens. The mix of neoantigen vaccination and chemoradiotherapy could be important when the quantity of tumor neoantigens in the vaccine is too low to activate a T-cell response [Citation131]. Radiotherapy can also increase the possibility of neoantigens being taken up by APCs to be presented to T cells to stimulate the immune response [Citation132]. Multiple studies have suggested the positive benefits of combining chemotherapy drugs in combination with cancer vaccines. For example, the use of CTX (cyclophosphamide) increased the activity of neoantigen-specific T cells, probably by inhibiting the function of regulatory T cells. When the chemotherapeutic drug dosage is lower than the dose at which cytopenia and immune suppression is triggered, the optimum immunomodulatory dose could be achieved [Citation133].
Moreover, in the microenvironment of tumor chemotherapy and radiotherapy can decrease immune suppression [Citation133]. Even though the use of radiotherapy or chemotherapy alone cannot eliminate the whole tumor mass in a typical tumor, the combination with neoantigen vaccines could show unique possibilities [Citation134]. ()
Figure 5. Anti-cancer vaccines combined with other types of cancer therapy. Anti-cancer vaccines based on DNA, RNA, SLPs, DCs, or viral vectors can be combined with traditional cancer therapies such as immunotherapy, radiotherapy, or chemotherapy to achieve a more effective anti-cancer response.
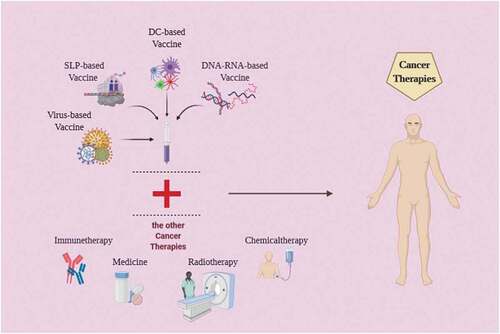
Although cancer vaccines have more advantages and fewer side effects than traditional types of cancer therapy, their use as monotherapy has not yielded very good results overall [Citation134]. Therefore, combinations with different strategies, for example, checkpoint inhibitors or mediators of immunogenic tumor cell death, could trigger a more effective anti-tumor response.
10. Conclusions and future perspectives
Neoantigens are defined are expressed mutated antigens by the tumor cells, specifically. The development of bioinformatics and sequencing technology has improved the recognition and identification of neoantigens. Neoantigen vaccines, including DNA, RNA, SLP, DC, and viral-based vaccines, have shown a promising therapeutic impact in several trials. The results suggest that tumor neoantigens play an essential role in immune escape, anti-tumor immune response, and successful cancer immunotherapy. Personalized cancer vaccines can either be utilized alone or combined with other treatments to increase anti-tumor immunity and enhance survival and quality of life in cancer patients. Further research is needed to elucidate in detail the mechanisms of immune suppression and tumor escape from immune attack.
Recent studies have studied the role of cell death signals involving apoptosis, necroptosis and ferroptosis in increasing the immune response against neoantigens. Immunogenic cell death (ICD) involves the release of pro-inflammatory cytokines and damage-associated molecular patterns from dying tumor cells. These released agents act as adjuvants, leading to the improved presentations of antigens, the maturation of DCs, and the induction of a stronger immune response. Necropotosis has been shown to be even more immunogenic than apoptosis. Combining ICDs and anti-cancer vaccines is a novel approach to achieve more effective results in the use of neoantigens in cancer vaccines [Citation135].
More effective neoantigen identification strategies will likely supply a wider range of candidate vaccines that could be tested in clinical trials. Nevertheless, there is still a long way to go before functional neo-antigen-based vaccines can be used. For example, there are still challenges in identifying and predicting the best epitopes with the most ideal immunogenicity using bioinformatics algorithms. Cheaper and faster vaccine manufacturing is also critical for providing reliable and prompt care to patients. Addressing these issues may help immunotherapies treat a wider range of patients and types of cancer with greater effectiveness than they do now.
11. Expert opinion
Targeting cancer cells without damaging normal cells is one of the main goals of researchers in the treatment of various types of cancer. Anti-cancer vaccines have emerged as therapeutic approaches to allow the immune system to specifically recognize and destroy cancer cells. Neoantigens, which are specifically expressed in cancer cells, mark these cells for destruction by the immune system. However, neoantigens that are spontaneously presented by cancer cells have several limitations. For example, although neoantigens are expressed in cancer cells, their degree of immunogenicity is insufficient. For this reason, the design of neoantigens with higher immunogenicity as well as more specific expression can mask the disadvantages of natural neoantigens. The pairing of next generation sequencing technology with advanced bioinformatics approaches has paved the way for the identification and design of cancer neoantigens. Despite many advances in bioinformatics, there is still the possibility of errors in neoantigen sequencing and structure prediction.
Based on the expression and delivery of the designed neonantigens, various types of anti-cancer vaccines have been developed that are being evaluated in clinical trials. These vaccines have included DNA, RNA, peptide, dendritic cell, and virus-based vaccines. Advantages of dendritic cell-based vaccines include their high immunogenicity and better control of antigen presentation. However, this method is very expensive and is often laborious to carry out. On the other hand, peptide vaccines can be produced cheaply and easily, and they generally show low toxicity. However, their immunogenicity is not very strong and depends on the individual patient’s HLA type.
Vaccines based on oncolytic or other viruses show high immunogenicity, and their ease of production has made them very popular [Citation136]. Nevertheless, there are disadvantages to viral vaccines, such as the potential for high toxicity, the risk of unwanted infections, and the development of an immune response against the vector itself (not the cancer antigen). Since genetic vaccines contain DNA sequences that encode antigens, several different antigens can be incorporated in a single vaccine. In addition, these vaccines are able to stimulate both humoral and cellular immunity and will not be dependent on the patient’s HLA type. In contrast, they are rather weak in terms of immunogenicity. Moreover, RNA-based vaccines require a delivery vehicle that can protect this unstable molecule from rapid degradation. In the meantime, due to the disadvantages of viral vectors, attention has been drawn to nanoparticles for vaccine delivery. In particular, nano-peptide particles were able to successfully transmit RNA encoding the novel coronavirus S-protein. Therefore, this strategy could be used to further improve cancer vaccines.
Although some vaccines against cancer neoantigens have advanced to the third phase of clinical trials, researchers still have challenges. In particular, for better results, these vaccines should continue to be used in combination with traditional cancer treatment methods such as chemotherapy or radiotherapy. However, chemotherapy and radiotherapy will have additional adverse effects which may damage the patient’s health.
It is necessary to improve the bioinformatics methods to better identify and predict neoantigens. Also, considering the advantaged of RNA-based vaccines, we can also focus on finding more efficient vectors to open a bright horizon for researchers and, of course, cancer patients.
Interestingly, recent findings have shown that the combination of induction of immunogenic cell death and cancer immunotherapy can increase the effectiveness of treatment many times over. This means that the cancer vaccines can stimulate the immune system against cancer cells, and in contrast to methods of inducing cell death such as apoptosis and necrosis, the immune response will be better. Although the path to achieving effective cancer vaccines in the clinical arena is still challenging, achieving significant improvements in the future does not seem far-fetched.
Article highlights
Neoantigens specifically stimulate the immune response against cancer cells when presented via MHC class I on the surface of tumor cell.
For stronger and more specific stimulation of the immune system, neoantigens with higher activity can be designed and inserted into cancer cells, which is possible using various carriers, including viruses.
The recognition of existing neoantigens and generation of vaccine antigens that can be used for anti-cancer vaccine production can be accomplished by next generation sequencing technology and advanced bioinformatics methods, which are more efficient and cheaper than the previous methods such as the cDNA libraries.
Vaccines can be designed based on RNA, DNA, specific peptides, or viruses, each with its advantages and disadvantages in the fight against cancer.
Declaration of statement
MRH declares the following potential conflicts of interest. Scientific Advisory Boards: Transdermal Cap Inc, Cleveland, OH; Hologenix Inc. Santa Monica, CA; Vielight, Toronto, Canada; JOOVV Inc, Minneapolis-St. Paul MN; Consulting; USHIO Corp, Japan; Sanofi-Aventis Deutschland GmbH, Frankfurt am Main, Germany. No potential conflict of interest was reported by the other author(s).
Reviewer disclosures
Peer reviewers on this manuscript have no relevant financial or other relationships to disclose.
Author’s contribution
N.E.,M.A, M.GH., P.R., S.A., M.BB and E.Y. contributed to the collecting data and writing the manuscript. A.A. and M.R.H contributed to revision and supervised the project
Additional information
Funding
References
- Havrilov A, Trehub Y. Oncolytic viruses as immunotherapeutic agents. Tehran, Iran: Tehran University of Medical Sciences; 2020.
- Coulie P, Van den Eynde B, van der Bruggen P, et al. Tumour antigens recognized by T lymphocytes: at the core of cancer immunotherapy. Nat Rev Cancer. 2014;14(2):135–146.
- Bobisse S, Foukas PG, Coukos G, et al. Neoantigen-based cancer immunotherapy. Ann Transl Med. 2016;4(14):262.
- Ward JP, Gubin MM, Schreiber RD. The role of neoantigens in naturally occurring and therapeutically induced immune responses to cancer. Adv Immunol. 2016;130:25–74.
- Topper MJ, Vaz M, Marrone KA, et al. The emerging role of epigenetic therapeutics in immuno-oncology. Nat Rev Clin Oncol. 2020;17(2):75–90.
- Efremova M, Finotello F. Rieder D and Trajanoski Z, Neoantigens generated by individual mutations and their role in cancer immunity and immunotherapy. Front Immunol. 2017;8:1679.
- Galluzzi L, Humeau J, Buqué A, et al. Immunostimulation with chemotherapy in the era of immune checkpoint inhibitors. Nat Rev Clin Oncol. 2020;17(12):725–741.
- Lesterhuis WJ, Haanen JB, Punt CJ. Cancer immunotherapy–revisited. Nat Rev Drug Discov. 2011;10(8):591–600.
- Vacchelli E, Prada N, Kepp O, et al. Current trends of anticancer immunochemotherapy. Milton Park, UK: Taylor & Francis; 2013.
- Garg NK, Dwivedi P, Prabha P, et al. RNA pulsed dendritic cells: an approach for cancer immunotherapy. Vaccine. 2013;31(8):1141–1156.
- Li Q, Ding Z-Y. The ways of isolating neoantigen-specific T cells. Front Oncol. 2020;10:1347.
- Rohaan MW, Wilgenhof S, Haanen JB. Adoptive cellular therapies: the current landscape. Virchows Arch. 2019;474(4):449–461.
- Alatrash G, Crain AK, Molldrem JJ. Tumor-associated antigens, in Immune biology of allogeneic hematopoietic stem cell transplantation. - 2019, Cambridge, MA, USA: Elsevier; p. -143-164.
- Hollingsworth RE, Jansen K. Turning the corner on therapeutic cancer vaccines. NPJ Vaccines. 2019;4(1):1–10.
- Wang R-F. Tumor antigens discovery: perspectives for cancer therapy. Mol Med. 1997;3(11):716–731.
- De S, Lurquin C, van der Bruggen C, et al. Sequence and expression pattern of the human MAGE2 gene. Immunogenetics. 1994;39(2):121–129.
- Gnjatic S, Ritter E, Büchler WM, et al. Seromic profiling of ovarian and pancreatic cancer. Proc Natl Acad Sci. 2010;107(11):5088–5093.
- Hofmann O, Caballero O, Stevenson B, et al. Genome-wide analysis of cancer/testis gene expression. Proc Natl Acad Sci. 2008;105(51):20422–20427.
- Simpson A, Caballero O, Jungbluth A, et al. Cancer/testis antigens, gametogenesis and cancer. Nat Rev Cancer. 2005;5(8):615–625.
- Bakker A, Schreurs M, De Boer AJ, et al. Melanocyte lineage-specific antigen gp100 is recognized by melanoma-derived tumor-infiltrating lymphocytes. J Exp Med. 1994;179(3):1005–1009.
- Kawakami Y, Eliyahu S, Delgado CH, et al. Cloning of the gene coding for a shared human melanoma antigen recognized by autologous T cells infiltrating into tumor. Proc Natl Acad Sci. 1994;91(9):3515–3519.
- Parkhurst MR, Fitzgerald EB, Southwood S, et al. Identification of a shared HLA-A* 0201-restricted T-cell epitope from the melanoma antigen tyrosinase-related protein 2 (TRP2). Cancer Res. 1998;58(21):4895–4901.
- Correale P, Nieroda C, Zaremba S, et al. In vitro generation of human cytotoxic T lymphocytes specific for peptides derived from prostate-specific antigen. J Natl Cancer Inst. 1997;89(4):293–300.
- Lam K-W, Li C-Y, Yam LT, et al. Improved immunohistochemical detection of prostatic acid phosphatase by a monoclonal antibody. Prostate. 1989;15(1):13–21.
- Vonderheide RH, Hahn WC, Schultze JL, et al. The telomerase catalytic subunit is a widely expressed tumor-associated antigen recognized by cytotoxic T lymphocytes. Immunity. 1999;10(6):673–679.
- Disis ML, Wallace DR, Gooley TA, et al. Concurrent trastuzumab and HER2/neu-specific vaccination in patients with metastatic breast cancer. J Clin Oncol. 2009;27(28):4685.
- Chang K, Pastan I. Molecular cloning of mesothelin, a differentiation antigen present on mesothelium, mesotheliomas, and ovarian cancers. Proc Natl Acad Sci. 1996;93(1):136–140.
- Finn OJ, Gantt KR, Lepisto AJ, et al. Importance of MUC1 and spontaneous mouse tumor models for understanding the immunobiology of human adenocarcinomas. Immunol Res. 2011;50(2–3):261–268.
- Lee C-M, Lu S-N, Changchien C-S, et al. Age, gender, and local geographic variations of viral etiology of hepatocellular carcinoma in a hyperendemic area for hepatitis B virus infection. Cancer. 1999;86(7):1143–1150.
- Paavonen J, Naud P, Salmerón J, et al. Efficacy of human papillomavirus (HPV)-16/18 AS04-adjuvanted vaccine against cervical infection and precancer caused by oncogenic HPV types (PATRICIA): final analysis of a double-blind, randomised study in young women. Lancet. 2009;374(9686):301–314.
- Butts C, Socinski MA, Mitchell PL, et al. Tecemotide (L-BLP25) versus placebo after chemoradiotherapy for stage III non-small-cell lung cancer (START): a randomised, double-blind, phase 3 trial. Lancet Oncol. 2014;15(1):59–68.
- Ghanaat M, Goradel NH, Arashkia A, et al. Virus against virus: strategies for using adenovirus vectors in the treatment of HPV-induced cervical cancer. Acta Pharmacol Sin. 2021; DOI:10.1038/s41401-021-00616-5.
- Bräunlein E, Krackhardt AM. Identification and characterization of neoantigens as well as respective immune responses in cancer patients. Front Immunol. 2017;8:1702.
- Schumacher T, Bunse L, Pusch S, et al. A vaccine targeting mutant IDH1 induces antitumour immunity. Nature. 2014;512(7514):324–327.
- Wang R-F, Wang HY. Immune targets and neoantigens for cancer immunotherapy and precision medicine. Cell Res. 2017;27(1):11–37.
- Tang S, Madhavan S. neoantigen R: an annotation based pipeline for tumor neoantigen identification from sequencing data. bioRxiv. 2017;171843. DOI: 10.1101/171843.
- Tappeiner E, Finotello F, Charoentong P, et al. TIminer: NGS data mining pipeline for cancer immunology and immunotherapy. Bioinformatics. 2017;33(19):3140–3141.
- Hundal J, Carreno BM, Petti AA, et al. pVAC-Seq: a genome-guided in silico approach to identifying tumor neoantigens. Genome Med. 2016;8(1):1–11.
- Javidi MA, Ahmadi AH, Bakhshinejad B, et al. Cell-free microRNAs as cancer biomarkers: the odyssey of miRNAs through body fluids. Med Oncol. 2014;31(12):295.
- Bassani-Sternberg M, Bräunlein E, Klar R, et al. Direct identification of clinically relevant neoepitopes presented on native human melanoma tissue by mass spectrometry. Nat Commun. 2016;7(1):1–16.
- Bassani-Sternberg M. Mass Spectrometry Based Immunopeptidomics for the Discovery of Cancer Neoantigens. Methods Mol Biol. 2018;1719:209-221. DOI:10.1007/978-1-4939-7537-2_14
- Snyder A, Makarov V, Merghoub T, et al. Genetic basis for clinical response to CTLA-4 blockade in melanoma. N Engl J Med. 2014;371(23):2189–2199.
- Gros A, Garcia-Garijo A, Fajardo CA. Determinants for neoantigen identification. Front Immunol. 2019;10:1392.
- Finlay BB, McFadden G. Anti-immunology: evasion of the host immune system by bacterial and viral pathogens. Cell. 2006;124(4):767–782.
- Kuroki M, Ueno A, Matsumoto H, et al. Significance of tumor-associated antigens in the diagnosis and therapy of cancer: an overview. Anticancer Res. 2002;22(6C):4255–4264.
- Wolfel T, Hauer M, Schneider J, et al. A p16INK4a-insensitive CDK4 mutant targeted by cytolytic T lymphocytes in a human melanoma. Science. 1995;269(5228):1281–1284.
- Coulie PG, Lehmann F, Lethe B, et al. A mutated intron sequence codes for an antigenic peptide recognized by cytolytic T lymphocytes on a human melanoma. Proc Natl Acad Sci. 1995;92(17):7976–7980.
- Brändle D, Brasseur F, Weynants P, et al. A mutated HLA-A2 molecule recognized by autologous cytotoxic T lymphocytes on a human renal cell carcinoma. J Exp Med. 1996;183(6):2501–2508.
- Hogan KT, Eisinger DP, Cupp SB, et al. The peptide recognized by HLA-A68. 2-restricted, squamous cell carcinoma of the lung-specific cytotoxic T lymphocytes is derived from a mutated elongation factor 2 gene. Cancer Res. 1998;58(22):5144–5150.
- Wu J, Zhao W, Zhou B, et al. TSNAdb: a database for tumor-specific neoantigens from immunogenomics data analysis. Genomics Proteomics Bioinformatics. 2018;16(4):276–282.
- Arnaud M, Duchamp M, Bobisse S, et al. Biotechnologies to tackle the challenge of neoantigen identification. Curr Opin Biotechnol. 2020;65:52–59.
- Matsushita H, Vesely MD, Koboldt DC, et al. Cancer exome analysis reveals a T-cell-dependent mechanism of cancer immunoediting. Nature. 2012;482(7385):400–404.
- Roudko V, Greenbaum B, Bhardwaj N. Computational prediction and validation of tumor-associated neoantigens. Front Immunol. 2020;11:27.
- Saini SK, Rekers N, Hadrup SR. Novel tools to assist neoepitope targeting in personalized cancer immunotherapy. Ann Oncol. 2017;28:xii3–xii10.
- Orentas RJ, Nordlund J, He J, et al. Bioinformatic description of immunotherapy targets for pediatric T-cell leukemia and the impact of normal gene sets used for comparison. Front Oncol. 2014;4:134.
- Hammerbacher J, Snyder A. Informatics for cancer immunotherapy. Ann Oncol. 2017;28:xii56–xii73.
- Linnemann C, Van Buuren MM, Bies L, et al. High-throughput epitope discovery reveals frequent recognition of neo-antigens by CD4+ T cells in human melanoma. Nature Med. 2015;21(1):81–85.
- Amirmahani F, Ebrahimi N, Molaei F, et al. Approaches for the integration of big data in translational medicine: single-cell and computational methods. Ann N Y Acad Sci.
- Li K, Vaudel M, Zhang B, et al. PDV: an integrative proteomics data viewer. Bioinformatics. 2019;35(7):1249–1251.
- Wen B, Li K, Zhang Y, et al. Cancer neoantigen prioritization through sensitive and reliable proteogenomics analysis. Nat Commun. 2020;11(1):1–14.
- Lancaster EM, Jablons D, Kratz JR. Applications of next-generation sequencing in neoantigen prediction and cancer vaccine development. Genet. Test. Mol. Biomark. 2020;24(2):59–66.
- McKenna A, Hanna M, Banks E, et al. The Genome Analysis Toolkit: a MapReduce framework for analyzing next-generation DNA sequencing data. Genome Res. 2010;20(9):1297–1303.
- Koboldt DC, Zhang Q, Larson DE, et al. VarScan 2: somatic mutation and copy number alteration discovery in cancer by exome sequencing. Genome Res. 2012;22(3):568–576.
- Hackl H, Charoentong P, Finotello F, et al. Computational genomics tools for dissecting tumour–immune cell interactions. Nat Rev Genet. 2016;17(8):441.
- Szolek A, Schubert B, Mohr C, et al. OptiType: precision HLA typing from next-generation sequencing data. Bioinformatics. 2014;30(23):3310–3316.
- Shukla SA, Rooney MS, Rajasagi M, et al. Comprehensive analysis of cancer-associated somatic mutations in class I HLA genes. Nat Biotechnol. 2015;33(11):1152–1158.
- Boegel S, Löwer M, Schäfer M, et al. HLA typing from RNA-Seq sequence reads. Genome Med. 2013;4(12):1–12.
- Warren RL, Choe G, Freeman DJ, et al. Derivation of HLA types from shotgun sequence datasets. Genome Med. 2012;4(12):1–8.
- Lundegaard C, Lamberth K, Harndahl M, et al. NetMHC-3.0: accurate web accessible predictions of human, mouse and monkey MHC class I affinities for peptides of length 8–11. Nucleic Acids Res. 2008;36(suppl_2):W509–W512.
- Nielsen M, Andreatta M. NetMHCpan-3.0; improved prediction of binding to MHC class I molecules integrating information from multiple receptor and peptide length datasets. Genome Med. 2016;8(1):1–9.
- Bjerregaard A-M, Nielsen M, Hadrup SR, et al. MuPeXI: prediction of neo-epitopes from tumor sequencing data. Cancer Immunol Immunother. 2017;66(9):1123–1130.
- Wick DA, Webb JR, Nielsen JS, et al. Surveillance of the tumor mutanome by T cells during progression from primary to recurrent ovarian cancer. Clin Cancer Res. 2014;20(5):1125–1134.
- Melero I, Gaudernack G, Gerritsen W, et al. Therapeutic vaccines for cancer: an overview of clinical trials. Nat Rev Clin Oncol. 2014;11(9):509.
- Li L, Goedegebuure S, Gillanders WE. Preclinical and clinical development of neoantigen vaccines. Ann Oncol. 2017;28:xii11–xii17.
- Schumacher TN, Schreiber RD. Neoantigens in cancer immunotherapy. Science. 2015;348(6230):69–74.
- Kuai R, Ochyl LJ, Bahjat KS, et al. Designer vaccine nanodiscs for personalized cancer immunotherapy. Nat Mater. 2017;16(4):489–496.
- Fang Y, Mo F, Shou J, et al. A pan-cancer clinical study of personalized neoantigen vaccine monotherapy in treating patients with various types of advanced solid tumors. Clin Cancer Res. 2020;26(17):4511–4520.
- Yadav M, Jhunjhunwala S, Phung QT, et al. Predicting immunogenic tumour mutations by combining mass spectrometry and exome sequencing. Nature. 2014;515(7528):572–576.
- Beatty GL, Gladney WL. Immune escape mechanisms as a guide for cancer immunotherapy. Clin Cancer Res. 2015;21(4):687–692.
- Hu Z, Ott PA, Wu CJ. Towards personalized, tumour-specific, therapeutic vaccines for cancer. Nat Rev Immunol. 2018;18(3):168.
- Moy JD, Moskovitz JM, Ferris RL. Biological mechanisms of immune escape and implications for immunotherapy in head and neck squamous cell carcinoma. Eur J Cancer. 2017;76:152–166.
- Ott PA, Hu Z, Keskin DB, et al. An immunogenic personal neoantigen vaccine for patients with melanoma. Nature. 2017;547(7662):217–221.
- Sahin U, Derhovanessian E, Miller M, et al. Personalized RNA mutanome vaccines mobilize poly-specific therapeutic immunity against cancer. Nature. 2017;547(7662):222–226.
- Keskin DB, Anandappa AJ, Sun J, et al. Neoantigen vaccine generates intratumoral T cell responses in phase Ib glioblastoma trial. Nature. 2019;565(7738):234–239.
- Hilf N, Kuttruff-Coqui S, Frenzel K, et al. Actively personalized vaccination trial for newly diagnosed glioblastoma. Nature. 2019;565(7738):240–245.
- Bhatt D, Daemen T, Vaccines T, et al., 2020, Multidisciplinary digital publishing institute.
- Garg AD, Galluzzi L, Apetoh L, et al. Molecular and translational classifications of DAMPs in immunogenic cell death. Front Immunol. 2015;6:588.
- Jiang T, Shi T, Zhang H, et al. Tumor neoantigens: from basic research to clinical applications. J Hematol Oncol. 2019;12(1):1–13.
- Mondal M, Guo J, He P, et al. Recent advances of oncolytic virus in cancer therapy. Hum Vaccines Immunother. 2020;16(10):2389–2402.
- Guo C, Manjili MH, Subjeck JR, et al. Therapeutic cancer vaccines: past, present, and future. Adv Cancer Res. 2013;119:421–475.
- Stratford R, Douce G, Zhang-Barber L, et al. Influence of codon usage on the immunogenicity of a DNA vaccine against tetanus. Vaccine. 2000;19(7–8):810–815.
- Lin EY, Li J-F, Gnatovskiy L, et al. Macrophages regulate the angiogenic switch in a mouse model of breast cancer. Cancer Res. 2006;66(23):11238–11246.
- Ribas A, Weber JS, Chmielowski B, et al. Intra–lymph node prime-boost vaccination against melan A and tyrosinase for the treatment of metastatic melanoma: results of a phase 1 clinical trial. Clin Cancer Res. 2011;17(9):2987–2996.
- Weber JS, Vogelzang NJ, Ernstoff MS, et al. A phase 1 study of a vaccine targeting preferentially expressed antigen in melanoma and prostate-specific membrane antigen in patients with advanced solid tumors. J Immunother. 2011;34(7):556. Hagerstown, Md: 1997.
- Duperret EK, Perales-Puchalt A, Stoltz R, et al. A synthetic DNA, multi-neoantigen vaccine drives predominately MHC class I CD8+ T-cell responses, impacting tumor challenge. Cancer Immunol Res. 2019;7(2):174–182.
- Scheel B, Teufel R, Probst J, et al. Toll‐like receptor‐dependent activation of several human blood cell types by protamine‐condensed mRNA. Eur J Immunol. 2005;35(5):1557–1566.
- Carralot J-P, Probst J, Hoerr I, et al. Polarization of immunity induced by direct injection of naked sequence-stabilized mRNA vaccines. Cell Mol Life Sci. 2004;61(18):2418–2424.
- Pardoll DM. The blockade of immune checkpoints in cancer immunotherapy. Nat Rev Cancer. 2012;12(4):252–264.
- Brest P, Refae S, Mograbi B, et al. Host polymorphisms may impact SARS-CoV-2 infectivity. Trends Genet. 2020;36(11):813–815.
- Gosain R, Abdou Y, Singh A, et al. COVID-19 and cancer: a comprehensive review. Curr Oncol Rep. 2020;22(5):1–15.
- Fanciullino R, Ciccolini J, Milano G. COVID-19 vaccine race: watch your step for cancer patients. Br J Cancer. 2021;124(5):860–861.
- Bonehill A, Van Nuffel AM, Corthals J, et al. Single-step antigen loading and activation of dendritic cells by mRNA electroporation for the purpose of therapeutic vaccination in melanoma patients. Clin Cancer Res. 2009;15(10):3366–3375.
- Jäger E, Gnjatic S, Nagata Y, et al. Induction of primary NY-ESO-1 immunity: CD8+ T lymphocyte and antibody responses in peptide-vaccinated patients with NY-ESO-1+ cancers. Proc Natl Acad Sci. 2000;97(22):12198–12203.
- Ménager J, Ebstein F, Oger R, et al. Cross-presentation of synthetic long peptides by human dendritic cells: a process dependent on ERAD component p97/VCP but Not sec61 and/or Derlin-1. PLoS One. 2014;9(2):e89897.
- Schuette V, Burgdorf S. The ins-and-outs of endosomal antigens for cross-presentation. Curr Opin Immunol. 2014;26:63–68.
- Ho NI, Huis in ‘t Veld LGM, Raaijmakers TK, et al. Adjuvants enhancing cross-presentation by dendritic cells: the key to more effective vaccines? Front Immunol. 2018;9:2874.
- Cai A, Keskin DB, DeLuca DS, et al. Mutated BCR-ABL generates immunogenic T-cell epitopes in CML patients. Clin Cancer Res. 2012;18(20):5761–5772.
- Chen F, Zou Z, Du J, et al. Neoantigen identification strategies enable personalized immunotherapy in refractory solid tumors. J Clin Investig. 2019;129(5):2056–2070.
- Hutchison S, Pritchard AL. Identifying neoantigens for use in immunotherapy. Mamm. Genome. 2018;29(11–12):714–730.
- Saxena M, Bhardwaj N. Re-emergence of dendritic cell vaccines for cancer treatment. Trends Cancer. 2018;4(2):119–137.
- Garg AD, Vara Perez M, Schaaf M, et al. Trial watch: dendritic cell-based anticancer immunotherapy. Oncoimmunology. 2017;6(7):e1328341.
- García-Vallejo JJ, Unger WW, Kalay H, et al. Glycan-based DC-SIGN targeting to enhance antigen cross-presentation in anticancer vaccines. Oncoimmunology. 2013;2(2):e23040.
- Carreno BM, Magrini V, Becker-Hapak M, et al. A dendritic cell vaccine increases the breadth and diversity of melanoma neoantigen-specific T cells. Science. 2015;348(6236):803–808.
- Zhang X, Sharma PK, Goedegebuure SP, et al. Personalized cancer vaccines: targeting the cancer mutanome. Vaccine. 2017;35(7):1094–1100.
- Walsh SR, Dolin R. Vaccinia viruses: vaccines against smallpox and vectors against infectious diseases and tumors. Expert Rev Vaccines. 2011;10(8):1221–1240.
- Madan RA, Bilusic M, Heery C, et al. Clinical evaluation of TRICOM vector therapeutic cancer vaccines. In: Seminars in oncology.
- Woller N, Gürlevik E, Ureche C-I, et al. Oncolytic viruses as anticancer vaccines. Front Oncol. 2014;4:188.
- Aaes TL, Kaczmarek A, Delvaeye T, et al. Vaccination with necroptotic cancer cells induces efficient anti-tumor immunity. Cell Rep. 2016;15(2):274–287.
- Turubanova VD, Balalaeva IV, Mishchenko TA, et al. Immunogenic cell death induced by a new photodynamic therapy based on photosens and photodithazine. J Immunother Cancer. 2019;7(1):350.
- Yatim N, Jusforgues-Saklani H, Orozco S, et al. RIPK1 and NF-κB signaling in dying cells determines cross-priming of CD8⁺ T cells. Science. 2015;350(6258):328–334.
- Chen DS, Mellman I. Oncology meets immunology: the cancer-immunity cycle. immunity. 2013;39(1):1–10.
- Rezaei N, Keshavarz-Fathi M, Vaccines for cancer immunotherapy: an evidence-based review on current status and future perspectives. 2018.
- Song Q, Zhang C-D, Wu X-H. Therapeutic cancer vaccines: from initial findings to prospects. Immunol Lett. 2018;196:11–21.
- Ali OA, Lewin SA, Dranoff G, et al. Vaccines combined with immune checkpoint antibodies promote cytotoxic T-cell activity and tumor eradication. Cancer Immunol Res. 2016;4(2):95–100.
- Taube JM, Anders RA, Young GD, et al. Colocalization of inflammatory response with B7-h1 expression in human melanocytic lesions supports an adaptive resistance mechanism of immune escape. Sci Transl Med. 2012;4(127):127ra37–127ra37.
- Chen L, Han X. Anti–PD-1/PD-L1 therapy of human cancer: past, present, and future. J Clin Invest. 2015;125(9):3384–3391.
- Spranger S, Spaapen RM, Zha Y, et al. Up-regulation of PD-L1, IDO, and Tregs in the melanoma tumor microenvironment is driven by CD8+ T cells. Sci Transl Med. 2013;5(200):200ra116–200ra116.
- Liu Y. Neoantigen: a long march toward cancer immunotherapy. Clin Cancer Res. 2016;22(11):2602–2604.
- Eil R, Vodnala SK, Clever D, et al. Ionic immune suppression within the tumour microenvironment limits T cell effector function. Nature. 2016;537(7621):539–543.
- Mroz P, Szokalska A, Wu MX, et al. Photodynamic therapy of tumors can lead to development of systemic antigen-specific immune response. PloS One. 2010;5(12):e15194.
- Gameiro SR, Jammed ML, Wattenberg MM, et al. Radiation-induced immunogenic modulation of tumor enhances antigen processing and calreticulin exposure, resulting in enhanced T-cell killing. Oncotarget. 2014;5(2):403.
- Lugade AA, Moran JP, Gerber SA, et al. Local radiation therapy of B16 melanoma tumors increases the generation of tumor antigen-specific effector cells that traffic to the tumor. J Immunol. 2005;174(12):7516–7523.
- Machiels J-PH, Reillym RT, Emensm LA, et al. Cyclophosphamide, doxorubicin, and paclitaxel enhance the antitumor immune response of granulocyte/macrophage-colony stimulating factor-secreting whole-cell vaccines in HER-2/neu tolerized mice. Cancer Res. 2001;61(9):3689–3697.
- Peng M, Mo Y, Wang Y, et al. Neoantigen vaccine: an emerging tumor immunotherapy. Mol Cancer. 2019;18(1):1–14.
- Aaes TL, Verschuere H, Kaczmarek A, et al. Immunodominant AH1 antigen-deficient necroptotic, but not apoptotic, murine cancer cells induce antitumor protection. J Immunol. 2020;204(4):775–787.
- Goradel NH, Baker AT, Arashkia A, et al. Oncolytic virotherapy: challenges and solutions. Curr Probl Cancer. 2021;45(1):100639.