ABSTRACT
Introduction
Generalized-Modules-for-Membrane-Antigens (GMMA) is a technology platform developed to design outer membrane vesicle (OMV)-based vaccines. GMMA are basically OMVs derived from a bacterial strain specifically engineered to obtain a fit-for-purpose and affordable vaccine by potentiating, or deleting, expression of specific genes. OMVs can be used as a carrier for antigens by inducing their expression on them, with the aim to improve antigen immunogenicity and design multivalent combination vaccines.
Areas Covered
We expanded this finding to show that the chemical conjugation of different proteic and/or polysaccharidic antigens, to GMMA, is a methodology complementary to the genetic manipulation to obtain highly effective combination vaccines. Here we discuss our findings with a specific focus on the impact that GMMA technology can have on global health, as this technology platform is particularly suited to support the development of affordable vaccines for low-income countries.
Expert Opinion
We believe that it is critical to elucidate the mode of action of GMMA immunogenicity and have provided a summarized description of the immunological questions to be addressed in the near future. The improved knowledge of GMMA might lead to designing more effective and safer GMMA-based vaccines to tackle the most serious vaccine-preventable diseases.
1. Introduction
Some bacterial cell types can spontaneously release membrane blebs [Citation1–3]. This phenomenon is particularly evident in several species of Gram-negative bacteria that generate vesicles of various size (roughly 20–200 nm) from the outer membrane, commonly indicated as Outer Membrane Vesicles (OMVs) [Citation1–6].
OMVs are supposed to have several different roles in the physiology of bacteria that release them, aimed at increasing the bacterial fitness in their growing environment [Citation1–3,Citation7]. For example, OMVs may help the biofilm formation mediating the attachment of the bacteria to epithelial cells or facilitate the nutrient acquisition in the bacterial population during growth, due to the enzymes packaged in the OMV structure [Citation1–3,Citation8,Citation9]. In addition, OMVs may work as decoy of bacterial cells to evade host antibody response, antibiotic activity against OMV proteins, anti-microbial agents or bacteriophage attack [Citation1–3,Citation10]. OMVs can also function as long-distance delivery system for toxins, virulence factors, or proteins critical for bacterial growth and survival [Citation1–3]. Since OMVs may contain nucleic acids, it has been also proposed that they play a role in gene transfer among bacterial cells, for example antibiotic resistance plasmids [Citation1–3]. Finally, OMVs may simply be the consequence of an imbalance between bacterial growth and outer membrane biosynthesis, which results in the release of the excess membrane material as OMVs [Citation1–3].
2. OMV and vaccines
Whatever their physiological function, for a long time OMVs have been considered very striking for vaccine design [Citation1–3,Citation11,Citation12]. OMVs, in fact, are naturally produced subunits of bacterial pathogens bearing multiple surface-exposed antigens in native conformation and orientation [Citation1–3,Citation12]. In addition, OMVs contain pathogen-associated molecular patterns (PAMPs), such as lipopolysaccharides (LPS), peptidoglycans, lipoproteins, flagella, that do not work only as antigens, but also are able to stimulate the innate immune system and ultimately induce an immune response [Citation1–3,Citation13–16]. In other words, OMVs can be basically considered ‘self-adjuvanted’ multiple vaccine antigens [Citation1–3,Citation12,Citation17,Citation18]. On top of that, the vesicle nature of OMVs and their size make them easily deliverable to immune cells, particularly to antigen presenting cells (APCs), that in turn trigger the T-cell response and the development of an effective immunity [Citation1–3,Citation18–21].
For all these reasons, OMVs have been extensively studied as vaccine components in preclinical and clinical research and firstly used in human vaccines against Neisseria menigitidis serogroup B (MenB) [Citation1–3,Citation18,Citation22–24]. In particular, three OMV-based vaccines against specific MenB strains were licensed and administered in humans to control epidemics of those particular MenB clones in Chile, Cuba, Norway, Normandy, Brazil and New Zealand [Citation22–24]. These vaccines were formulated through adsorption of OMVs to aluminum hydroxide adjuvant (Alum) and designed to be administered through intramuscular injection [Citation22–24]. The first manufactured broadly protective MenB vaccine was an OMV-based vaccine containing the OMVs of the previous licensed New Zealand MenB vaccine together with three recombinant MenB protein antigens described to generate cross-protection against several MenB unrelated strains [Citation22–24]. This four-component MenB vaccine (4CMenB) is formulated with Alum and it is administered intramuscularly [Citation22–27].
The landscape of the OMV-based vaccines has been reviewed elsewhere, focusing the attention on meningococcal vaccines, designed, and developed with OMV technology and non-meningococcal OMV-based vaccines [Citation12]. Regarding these latter, several preclinical studies were done with different types of natural OMVs released spontaneously by wild-type bacteria and not extracted with specific methods, confirming the strong immunogenicity and/or protection efficacy conferred by immunization with OMVs [Citation12]. Immunization with OMVs from Burkholderia pseudomallei induced protection against challenge in mice and promoted a strong antibody response against OMVs, LPS, and capsular polysaccharide [Citation28]. Immune sera from OMV immunized mice showed passive protection [Citation28]. OMVs from Salmonella typhimurium, administered intranasally in mice elicited a strong, cross-reactive mucosal immune response against Salmonella choleraesuis and Salmonella enteritidis, which also induced an effective protective response [Citation29]. An optimal protection efficacy after mucosal immunization in mice was also observed using Shigella flexneri OMVs [Citation30]. In another study, always in the murine model, a mixture of OMVs from different Shigella strains was able to elicit a good antibody response against these strains and passive protection to offspring [Citation31]. Consistently, the stomach of the offspring contained mother’s milk with high Shigella-specific antibody titers [Citation31]. Mouse intranasal immunization with Non-typeable Haemophilus influenzae (NTHi) OMVs induced a strong NTHi-specific humoral and mucosal immune response, this also increased protection against nasopharingeal colonization with both homologous and heterologous bacterial strains [Citation32]. Immunization of mice with Vibrio cholerae OMVs was highly immunogenic, independently of the immunization route and it was long lasting [Citation33–35]. OMVs from Vibrio cholerae were able to also show a mucosal immunity [Citation33–35]. Immunized mice were protected from Vibrio cholerae challenge and a mixture of different OMVs mucosally administered, showed a cross-protection [Citation33–35]. Active immunization with OMVs from Pseudomonas aeruginosa or passive immunization with sera of OMV immunized mice, conferred protection against a lethal challenge [Citation36,Citation37]. High levels of specific antibodies were produced and good opsonophagocytosis killing activity shown [Citation36,Citation37]. Immunization with Klebsiella pneumoniae OMVs promoted a strong humoral and cellular response and generated a protection against lethal challenge, in mice [Citation38,Citation39]. Also, the passive transfer of sera and splenocytes demonstrated that protection was shown by both cellular and humoral immunity [Citation38,Citation39]. OMVs from Bordetella pertussis induced a strong humoral and cellular immune response in mice, if compared to the standard vaccines [Citation40–42]. This potent immune response was able to show an optimal protection, even better than the standard vaccines [Citation40–42]. In the end, Francisella OMVs were able to promote a good protection in mice [Citation43].
Despite the ability of MenB strains to naturally shed OMVs similarly to the other pathogens reported previously, their production for vaccine manufacturing has been carried out by detergent extraction [Citation1,Citation3,Citation22–24]. Essentially, bacteria were harvested from the cell culture discarding the supernatant containing naturally produced OMVs and then treated with a detergent to generate OMVs [Citation1,Citation3,Citation22–24]. This methodology allowed to significantly increase the OMV yield from bacterial culture, which was mandatory for vaccine manufacturing [Citation1,Citation3,Citation22–24]. Detergent extraction allows to largely remove LPS and most of the lipoproteins of the OMVs, improving consequently the tolerability of the vaccines [Citation1,Citation3,Citation22–24]. Lipoproteins and LPS are agonists of Toll-like Receptor 2 (TLR2) and TLR4 respectively, that are expressed in multiple immune cell types, including APCs or other cells of the innate immune system [Citation13–15]. Once stimulated via TLRs, immune cells release inflammatory/pyrogenic mediators such as cytokines and chemokines [Citation13–15]. The detergent extraction eliminates antigens that can be critical for vaccine efficacy and broad protection, such as the lipoprotein factor H binding protein (fHbp) [Citation1,Citation3,Citation22–24,Citation44,Citation45]. The 4CMenB vaccine was designed supplementing OMVs with recombinant surface MenB antigens, including fHbp [Citation1,Citation3,Citation22–24,Citation26]. This vaccine is conservatively estimated to provide 66–91% coverage against meningococcal serogroup B strains worldwide [Citation25]. The adsorption of Alum led to reduced pyrogenicity in preclinical studies [Citation22,Citation46]. Considering the mode of action of Alum adjuvant which, among other mechanisms, should be based on the entrapment of adsorbed antigen at injection site, the reduced pyrogenicity might be due to the limitation of systemic exposure of OMVs trapped at the injection site, though no critical difference in systemic reactogenicity was observed in humans between Alum adsorbed and free injected OMVs [Citation46,Citation47]. However, the adsorbtion of Alum is still considered important for the safety of the OMV-based vaccines. In the case of 4CMenB vaccine, the formulation with Alum also improved the stability of the recombinant antigens [Citation48].
The OMV production can be also carried out by detergent-free methods, such as sonication, vortexing or different chemical treatments that may improve the OMV yield without, or moderately, affecting their PAMP (lipoproteins/LPS) composition [Citation1,Citation3]. However, both detergent and detergent-free methodologies, aimed at increasing the OMV yield, need a non-trivial purification protocol as OMVs can be easily contaminated by cytoplasmic entities (detergent extracted OMVs should be also purified from detergent contamination) [Citation1,Citation3,Citation22]. To simplify the purification procedure, an elegant method has been implemented resulting in a technology platform for manufacturing of OMV based-vaccines: the Generalized Modules for Membrane Antigens (GMMA) technology [Citation17,Citation49,Citation50].
3. GMMA
GMMA are basically OMVs generated from bacterial strains genetically modified to design a ‘fit for purpose’ and affordable vaccine [Citation12,Citation17,Citation50,Citation51]. Some Gram-negative bacteria display a capacity of vesicle release, which is sufficiently effective to recover enough OMVs to implement a vaccine manufacturing process [Citation3,Citation49]. In many Gram-negative pathogens it is necessary to introduce genetic mutation to enhance the bleb release [Citation12,Citation17,Citation50,Citation51]. This genetic engineering can affect different molecular mechanisms depending on the bacterial species, but it is generally aimed at disrupting the anchorage of the outer membrane to the peptidoglycan layer in the periplasm [Citation17,Citation52–56]. This approach allows to obtain GMMA from the supernatant of bacterial cell culture through simple and economic methods of purification [Citation17,Citation51,Citation57,Citation58]. GMMA is not affected in their surface lipoprotein and LPS (or other PAMPs) composition and contain pathogen antigens in their native environment, differently to OMVs extracted with detergent or mechanical methods [Citation1,Citation3,Citation12]. This advantage may be problematic for vaccine design as GMMA contains the native form of LPS, which is reactogenic and known to induce a temporary temperature rise once injected, although the membrane bound LPS has been observed to be less pyrogenic than soluble LPS [Citation22,Citation59,Citation60]. To overcome this problem, in the bacterial strains used as a source of GMMA, additional mutations can be introduced to reduce the LPS activity without eliminating its immunostimulatory effect, which can be helpful for the ‘self-adjuvanticity’ of GMMA [Citation1,Citation3,Citation16–18]. This genetic modification, also called GMMA detoxification, is usually aimed at reducing the acylation and/or phosphorylation of the Lipid A of LPS, which results in a negative impact for the recognition/triggering of TLR4 [Citation16,Citation61,Citation62]. Detoxification may help to match the safety requirements for approval to market release of a GMMA-based vaccine.
Bacteria engineered to generate detoxified GMMA may be additionally modified to design a tailor-made vaccine [Citation1,Citation12]. For example, bacteria can be modified to: (a) overexpress desired homologous vaccine antigens or multiple variants of a homologous protective antigen; (b) retain and expose a secreted vaccine antigen on the surface; (c) delete any unwanted antigens that might negatively affect the generation of an effective immune response; (d) display heterologous antigens, either proteic or polysaccharidic, derived from different (even phylogenetically distant) pathogens (viral, bacterial, parasitic). Applying these options of manipulation, it is ultimately possible to obtain a ‘fit for purpose’ GMMA for the desired vaccine ().
Figure 1. The GMMA technology. The features of GMMA production are summarized in this diagram. Genetic manipulations are introduced in the bacterial strain used as source of GMMA with the aim to: (A) Improve the natural capacity of the Gram-negative bacterium to release outer membrane blebs. (B) Reduce the capacity of LPS to stimulate a reactogenic response upon GMMA injection in the body. (C) Remove antigens (Ags) that can be considered detrimental for the effectiveness/safety of the GMMA-based vaccine. (D) Overexpress antigen candidates that can improve the protective efficacy of the GMMA-based vaccine or are necessary for its design. Once GMMA are obtained, in addition to genetic manipulation of the bacterial cells, antigens can be chemically conjugated to GMMA with the same aim to generate GMMA carrying antigen candidates for the protective efficacy of the GMMA-based vaccine.
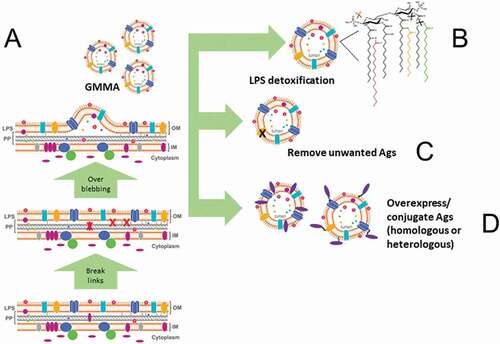
4. GMMA for global health
The vaccine market is particularly relevant for low and middle-income countries, where a major part of the world’s population resides, and the infectious diseases determine the most dramatic health and socio-economic consequences [Citation63] (http://ghdx.healthdata.org/gbd-results-tool). In fact, Malaria, Tuberculosis, and Acquired Immune Deficiency Syndrome (AIDS), which are considered the major high-burden infectious diseases globally, are endemic in several countries of the low- and middle-income regions of the world [Citation64,Citation65]. Tuberculosis, in particular, is a real poverty-driven disease that affects poor countries only [Citation66]. Diarrheal diseases, which are the second leading cause of deaths in children under 5 years of age, are prevalent in low-income countries, despite the fact they may be easily prevented through safe drinking-water, adequate sanitation, and hygiene, measures that are considered normal in high-income countries, but very challenging to be implemented in poor countries (https://www.who.int/news-room/fact-sheets/detail/diarrheal-disease). Diarrheal diseases can be caused by food and water contamination with several pathogens, including the Gram-negative bacteria Salmonella, Shigella, and Enterotoxigenic Escherichia coli (ETEC) [Citation67,Citation68] (https://www.who.int/news-room/fact-sheets/detail/diarrheal-disease).
Immunization is the main weapon against microbial infection worldwide, particularly in poorest countries [Citation64,Citation65].
Unfortunately, vaccines are not available against several pathogens responsible for serious diseases in low and middle-income countries, such as AIDS, Shigellosis, invasive non-typhoidal Salmonella (iNTS), ETEC [Citation67,Citation69–73]. In the case of Malaria, a vaccine that is able to reduce the disease in young children has been developed [Citation74]. This vaccine demonstrated a 30% efficacy in Phase III trial and a Malaria Vaccine Implementation Programme was established to support the introduction of the vaccine through the routine immunization programs in selected areas of Africa, with the aim to evaluate the vaccine’s public health impact on a broader scale [Citation74] (https://www.who.int/news-room/fact-sheets/detail/malaria). Tuberculosis has a vaccine (BCG; first used in 1920) currently available, but does not prevent primary infection and reactivation of latent pulmonary infection, thus its impact on the decline of disease incidence is limited [Citation66,Citation75]. Recently, a Tuberculosis vaccine candidate showed 54% of protective efficacy against the disease over 2 years of follow-up in a Phase IIb trial among Human Immunodeficiency Virus (HIV)-negative adults with evidence of latent Tuberculosis infection [Citation75].
The public health concern represented by serious vaccine-preventable diseases in low-income countries is further increased by the development of the antimicrobial resistance (AMR) [Citation76–80] (https://www.who.int/health-topics/antimicrobial-resistance). The emergence of circulating AMR strains poses the dramatic risk that diseases may become untreatable, with the potential to generate a global health crisis [Citation76–79] (https://www.who.int/health-topics/antimicrobial-resistance).
Immunization is one of the major countermeasures against AMR because it prevents the spread of pathogens and helps reduce the generation of vaccine-resistant strains [Citation76–79] (https://www.who.int/health-topics/antimicrobial-resistance). This occurs because vaccines are administered once, or few times in a lifetime, when circulating microorganisms are relatively low or absent in vaccinated population [Citation76–79] (https://www.who.int/health-topics/antimicrobial-resistance). Vaccines usually target several pathogenic mechanisms, requiring multiple mutations at the same time to confer resistance [Citation76–79] (https://www.who.int/health-topics/antimicrobial-resistance).
Despite the progress in vaccine development and the existence of effective vaccines, the research and development of novel effective vaccines is still critical. This is particularly important considering the opportunity to develop combination vaccines that offer protection from more than one disease at the same time, which can make the difference in low and middle-income countries.
However, the cost of vaccine development, certainly in the later stages, is so high that it cannot even be covered by the sales cost of the vaccine doses for immunization campaigns in poorer countries [Citation63]. The investment for vaccine development can be highly superior to the budget available for the delivery in low and middle-income countries [Citation63]. If a vaccine does not have the potential to be produced for a dual market, such as in developed and developing countries, the development of the vaccine is much more difficult for pharma companies [Citation63]. The dual market, in fact, makes sure that the financial profit derived from the high-income market, justifies the commitment to sell the vaccine in low-income market [Citation63]. When this dual-market strategy cannot be put in place, it is necessary to create a global partnership among different subjects, public entities, countries, private foundations, and even pharma companies, when possible, to attract financial investments, and transform these funds into a vaccine that can be administered to the human target population [Citation63]. The Meningococcus A (MenA) glycoconjugate vaccine for African countries of the so-called ‘meningitis belt’ (where the MenA disease burden was very high), the development path of the Malaria vaccine (which is in a pilot administration stage in target countries), and the Tuberculosis vaccine (in Phase II clinical development) are noteworthy examples of this kind of global effort to combat serious vaccine-preventable diseases by producing nonprofitable vaccines that are extremely critical for the health of millions of people [Citation63,Citation74,Citation75].
All technologies that allow the development of an effective vaccine, as quickly as possible and at low cost, have become decisive for implementation in immunization campaigns [Citation63].
As the manufacturing process of GMMA is straightforward and simple, because it's based on growing bacteria and separating GMMA released in the culture supernatant through filtration steps, the GMMA technology seems particularly attractive to design affordable vaccines, which is a critical aspect for immunization campaigns, especially in low and middle-income countries [Citation1,Citation3,Citation17,Citation49,Citation63].
From this perspective, GMMA is a technology platform with the potential to make a difference for global health.
5. The experience of Shigella sonnei GMMA-based vaccine
The first GMMA-based vaccine entered in clinical development has been the Shigella sonnei vaccine 1790GAHB [Citation51,Citation81–84]. This vaccine has been developed by the GSK Vaccine Institute for Global Health, and it is a good example of the commitment of different stakeholders in the development of a vaccine for an unmet medical need in low-income countries [Citation51,Citation81–83].
The Shigella vaccine 1790GAHB is composed of GMMA derived from a Shigella sonnei strain genetically manipulated to: (a) eliminate a protein participating in linking inner and outer membranes, thus inducing OMV overblebbing; (b) generate a pentacylated LPS, in order to reduce the immunostimulatory activity of lipid A and then improve the reactogenic profile of GMMA once injected [Citation51,Citation62]. In addition, these GMMA were adsorbed to Alhydrogel, which is basically Alum, to further improve the tolerability based on the pyrogenicity results in rabbits [Citation51].
The Shigella sonnei vaccine 1790GAHB contains 200 μg/ml of GMMA (based on protein dosage) and 0.7 mg/ml Alum (based on Al3+ ion dosage) [Citation51]. Different vaccine doses were obtained by bed-side mixing using placebo as diluent [Citation81,Citation83].
The GMMA for the vaccine was obtained by growing the bacterial strain as a source of GMMA and simply purifying GMMA from the culture supernatant through two consecutives steps of tangential flow filtration [Citation51].
On top of all the physico-chemical analyses performed to check the quality of the drug substance and the drug product, the 1790GAHB Shigella sonnei vaccine was analyzed for its biological activity [Citation51,Citation62].
The monocyte activation test confirmed that 1790-GMMA contained in the vaccine were 10-fold less active at inducing IL-6 release, compared to non-detoxified exacylated GMMA [Citation51]. The toxicology study in rabbits showed a good tolerability profile, with only a slight and transient increase of temperature during the first 6 hours after the administration [Citation51]. Also the Irwin study, aimed at evaluating the effect of vaccine to the nervous system after intranasal administration in rats, did not reveal pathological signs [Citation51]. The vaccine was demonstrated immunogenic both in rabbits and in mice, that were used to establish a potency assay for the vaccine lot release [Citation51]. The antigen selected to measure the antibody response was the LPS, which is considered the major protective antigen for Shigella sonnei [Citation51,Citation85].
The Sigella sonnei 1790GAHB vaccine was then tested in clinical trials [Citation81–83].
The first Phase I studies, conducted in healthy European adults, showed that the vaccine was well tolerated and immunogenic, when measuring the LPS-specific antibody response [Citation81,Citation82]. The intramuscular route of administration was observed as the preferred one for immunogenicity [Citation81,Citation82]. In particular, ≤6/100 μg (OAg/protein amount) of 1790GAHB per dose was well tolerated by the intramuscular route in healthy European adult, and ≥1.5/25 μg (OAg/protein amount) dose of 1790GAHB elicited serum IgG anti-Shigella sonnei LPS levels ≥121 ELISA units, which is the median antibody level in a panel of 87 convalescent subjects after natural infection by Shigella sonnei. Immunized subjects, via intramuscular administration, who had undetectable antibodies at the baseline in the parent study, received a booster dose 2–3 years later and displayed an enhanced LPS-specific antibody response [Citation81,Citation82]. Thus, the vaccine is capable to promote a good immunological memory. The functionality of the antibody response was measured by Serum Bactericidal Assay (SBA) and it has been shown that SBA titers increased with OAg dose, demonstrating a correlation between anti-Shigella sonnei SBA and LPS serum IgG titers [Citation84]. A Phase 2a study conducted in healthy Kenyan adults, a possible target population where shigellosis is endemic, confirmed that the vaccine, administered intramuscularly, was well tolerated and immunogenic [Citation83]. However, more recently 1.5 µg OAg of 1790GAHB failed to protect against bacterial challenge in a Shigella sonnei controlled human infection model for assessing vaccine efficacy [Citation86]. The amount of Shigella OAg on GMMA seems to be critical to induce a level of IgG antibodies able to protect from Shigella sonnei infection and a 4-component Shigella GMMA-based vaccine containing a 10-fold higher OAg dose of each of the components is currently entering clinical trials.
6. GMMA technology as a ‘plug and play’ tool to design combination vaccines and fulfill global health needs
From the perspective of using GMMA to design effective vaccines for global health, we believe that our recent work published in Vaccines offers a novel insight on how to better exploit this technology [Citation87].
OMVs and GMMA have been also proposed as innovative delivery system of heterologous proteins and carbohydrates, shown to generate an optimal immunization in preclinical studies [Citation1,Citation12,Citation88].
We have extended this finding by using chemical conjugation to display on GMMA-specific proteic or saccharidic antigens [Citation87]. Chemical conjugation is a rapid method to exploit GMMA as antigen carrier and it allows, within a certain range, to control amount and density of antigen displayed on GMMA vesicles [Citation87]. As chemical conjugation is feasible once the purified antigen is available, it is a complementary tool to the expression of antigens on GMMA [Citation87].
Such approaches can be used to design multivalent combination GMMA-based vaccines [Citation87]. The expression of desired antigens is more economical and straightforward, but not all bacterial strains can be easily modified to express additional antigens on their surface, especially if they are antigens derived from unrelated phylogenetically distant pathogens. Indeed, to maintain the essential bacterial functions required for high-yield fermentation, only a discrete amount of genetic modifications can be introduced in certain bacterial strains [Citation12]. These limitations reduce the possibility to redirect on the bacterial outer membrane the expression of as many antigens we want. Thus, the chemical conjugation represents a complementary technology from the perspective of designing a GMMA-based combination vaccine.
In our study, we showed, using the mouse model, that the effectiveness of the antigen-specific humoral immune response is significantly enhanced, when immunizing with GMMA bearing protein or saccharide antigens chemically conjugated [Citation87]. We made this observation using different GMMA-antigen heterologous models, such as Malaria protein antigens conjugated to Salmonella typhimurium GMMA, MenB fHbp to Salmonella typhimurium GMMA, ETEC proteins to Shigella sonnei GMMA, MenA or MenC oligosaccharide to MenB or Salmonella typhimurium GMMA, type b Hemophilus influenzae (Hib) oligosaccharide to MenB GMMA [Citation87]. All of these GMMA-antigen combinations, even though were models to prove the concept of our study, have a rationale for the design of specific vaccines: (1) Salmonella and Malaria diseases are endemic in the same area and clinical Malaria is considered a risk factor for iNTS disease [Citation69,Citation71]; (2) Salmonella and meningococcal diseases are both common in several countries of sub-Saharan Africa [Citation71,Citation73]; (3) ETEC and Shigella are the two Gram-negative bacteria prevalently associated with diarrhea (although the preference of a combination vaccine compared the two stand-alone vaccines is still debated) [Citation67]; (4) Hib and MenB are two critical etiological agents of meningitis (https://www.who.int/health-topics/meningitis#tab=tab_1); (5) A unique pan-meningococcal vaccine might be an optimal advancement to combat the meningococcal meningitis worldwide (https://www.who.int/initiatives/defeating-meningitis-by-2030).
GMMA-saccharide glycoconjugate showed a carrier effect, improving saccharide immunogenicity, compared to unconjugated carbohydrates, that were not or poorly immunogenic, as expected [Citation87]. Also, we observed that the carrier effect was superior to those obtained by conjugation to CRM197, a carrier protein extensively used in licensed vaccines [Citation87,Citation89,Citation90]. Interestingly, the carrier effect of GMMA was observed whether saccharides were attached to proteins or LPS molecules [Citation87]. The conjugation of Malaria protein antigens to GMMA elicited an antigen-specific antibody response that was superior to those induced by the physical mixture of antigens and GMMA at the same doses [Citation87]. By using MenB fHbp as protein antigen, despite the antibody response was similar when immunized with GMMA and fHbp conjugated or physically mixed at the same doses, the functionality of antibody response was significantly different [Citation87]. The conjugation to GMMA induced an antigen-driven bactericidal activity, which was notably superior to those elicited by the physical mixture [Citation87]. The bactericidal activity is a correlate of protection for meningococcus diseases in humans, and then it is an optimal functional assay to generate a proof of concept in preclinical studies [Citation23,Citation24]. In addition, the conjugation of MenB fHbp to GMMA promoted a functional antibody response also against MenA and MenW strains bearing heterologous variants of fHbp, confirming that the superior quality of antibody response stimulated by GMMA as an antigen carrier can promote a broad protection among meningococcal strains [Citation87].
Very interestingly, the chemical conjugation did not negatively affect the GMMA-specific humoral immune response both in terms of antibody production (e.g. analyzed via measuring anti-LPS response) and functionality (bactericidal activity) [Citation87].
In addition to these findings, we showed that the conjugation of two proteins or two saccharides on the same GMMA (bi-valent conjugates, such as ETEC antigens on the same Shigella GMMA or MenA and MenC saccharides on Salmonella GMMA) promoted an antigen-specific antibody response which was comparable to that induced by the monovalent conjugates, without affecting the GMMA-specific antibody response [Citation87]. Thus, the generation of bi-valent conjugates was not detrimental for both antigen and GMMA-specific humoral immune response, without leading to any immune-interference [Citation87].
In conclusion, our preclinical study in animal models generated the hypothesis that GMMA exploited as carrier for antigens, both proteic and saccharidic, can be used to induce effective immunization against multiple pathogens at the same time [Citation87]. In addition, our study showed that the chemical conjugation can be useful to this aim, thus complementing the already known approach to express antigens on GMMA [Citation87]. Ultimately, our results offer the proof of concept that GMMA as carrier for antigens can be a ‘plug and play’ tool to design highly effective multivalent combination vaccines, extending the value of GMMA as useful technology platform to combat a variety of infectious diseases that are still a threat for mankind [Citation87].
In the landscape of the most serious infectious diseases, mainly affecting low and middle-income countries, vaccine development is at clinical stage. Despite the advancement in vaccine development, to continue the research to find out broadly protective and affordable vaccines targeting multiple diseases at the same time, is vital. From this perspective, we believe that exploiting GMMA as antigen carrier to design tailored combination vaccines for the more at risk populations, offers novel insights to be further investigated, that might help the effort to improve global health.
7. Conclusion
GMMA is a technology platform particularly suited for designing and manufacturing of highly effective and affordable vaccines. For this reason, GMMA technology has the potential to make the difference in low-income countries and to significantly improve the effort to combat serious infectious diseases that are endemic in poor countries and are still a threat for the mankind. A vaccine against Shigella sonnei, which causes a serious diarrheal disease endemic in several low-income countries, is the first GMMA-based vaccine that entered clinical development. According to preclinical studies, GMMA are highly immunogenic also when used as carrier for antigens, enhancing significantly their immunogenicity. This opportunity further extends the potential of GMMA technology, which can be applied to design vaccines targeting multiple pathogens at the same time. The chemical conjugation of proteic and polysaccharidic antigens on GMMA expands this potential of GMMA technology in order to use it as a ‘plug and play’ tool to produce multivalent combination vaccines, which is a key aspect in case of several infectious diseases endemic in the same area of the world.
8. Expert opinion
To completely understand the mode of action of the potent carrier effect of GMMA is certainly critical in order to expand our knowledge of GMMA immunogenicity with the aim to better exploit it and consequently design ‘fit for purpose’ highly effective GMMA-based combination vaccines.
We believe that several key scientific points should be addressed in the future, to better understand the mode of action of GMMA immunogenicity and their carrier effect. Among all the possibilities, here we provide a list of mechanisms that we consider more relevant to this aim, from an immunological perspective and that deserve a high priority in future investigations ():
Figure 2. Immunological mechanisms of GMMA immunogenicity to be investigated with high priority. Diagram of the immunological mechanisms that should be carefully investigated to unveil the mode of action of GMMA immunogenicity. Innate immunity (left hand side): (1) Upon injection of GMMA, innate immune responses are triggered by direct or indirect activation of different immune cell types, which in turn promote the production of different factors (mainly cytokines) affecting the type and extent of the innate immune response. (2) TLRs are critical receptors for the triggering of innate immune responses and GMMA can have an active role on activating cells via these receptors due to their content of lipoproteins/peptidoglycan and LPS, that are TLR2 and TLR4 agonists respectively. Adaptive immunity (right hand side): (3) Antigen presentation by DCs induces activation of antigen-specific T cells, that in turn activate cognate B cells while presenting antigen to activated T cells. (4) Inside the germinal center, activated antigen-specific B cells encounter the antigen again presented by FDCs, undergoing to an additional and critical stimulation. (5) FDC-stimulated antigen-specific B cells interact with cognate Tfh cells to receive the stimulation which leads to complete the differentiation in antibody-secreting plasma cells or memory B cells. (6) This journey results in the production of antibodies that are functional and persistent inside the body, and in the establishment of the memory B cell compartment. (7) GMMA that arrive to the lymphoid organs via afferent lymphatics can be captured by SCSMs and delivered to antigen-specific B cells. (8) In addition, Mo-DCs, that can develop upon triggering of innate immune responses, may promote Tfh differentiation.
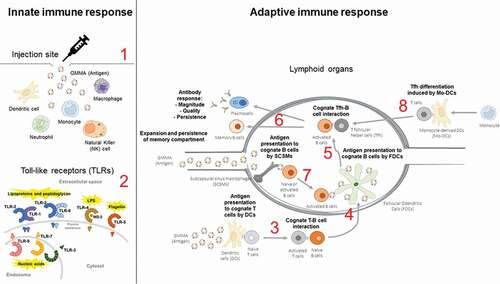
Innate immune response:
The innate immune response is a driver of the adaptive immune response.
1. The type of innate immune cells that undergo activation upon immunization with GMMA: GMMA can stimulate different cell types directly or indirectly due to bystander effects upon injection. In turn, the type of cells activated and the factors (mainly cytokines) produced upon their stimulation affect the type and extent of innate immune responses elicited.
2. Role of TLR engagement: TLRs play a central role in the stimulation of innate immune cells and GMMA contains TLR2 and TLR4 agonists.
Adaptive Immune response:
3. Improvement in antigen uptake and presentation capacity by APCs when an antigen is localized on GMMA and the intracellular trafficking of GMMA or antigen-GMMA conjugates upon their engulfment by APCs: antigen presentation by APCs to cognate T cells represents the central step of the induction of adaptive immune response and it is dependent on the intracellular fate of the antigen when it is engulfed by APCs and on its persistence inside their endosomal compartment.
4. Antigen presentation of activated cognate B cells by Follicular Dendritic Cells (FDCs): this mechanism facilitates a further encounter of antigen-experienced B cells (already activated by cognate T cells) with the cognate antigen inside the B cell follicle and represents a key step in the generation of germinal centers, which lead to memory B cell and/or long-lived plasma cell formation.
5. T follicular helper (Tfh) cell response: Tfh cells play a decisive role in the induction of an effective and durable humoral immunity and it takes place inside the B cell follicle upon the interaction between Tfh cells and cognate B cells, activated after the antigen presentation by FDCs.
6. Magnitude, quality, and persistence of antibody response, and the expansion of the memory B cell compartment: affinity of antigen-specific antibodies, isotype switching (among IgM, IgA, and IgG and among IgG subclasses), and somatic hypermutation in the Ig idiotype, together with the magnitude and the persistence of the antibody response in sera or mucosae, are all mechanisms critical for the development of an effective humoral immune response. In addition, the expansion of the memory B-cell compartment plays a primary role for the generation of a long-term humoral immunity.
7. The role of Subcapsular Sinus Macrophages (SCSMs): SCSMs are a peculiar subset of macrophages specialized in antigen transport inside the B cell follicle when the antigen is drained to the lymph node via the afferent lymphatic vessels and can exert an important role in the early activation of antigen-specific B cells by delivering the cognate antigen to B cells.
8. The role of monocyte-derived DCs (Mo-DCs): Mo-DCs has been described to have a prominent role at inducing Tfh response.
The expansion and deepening of our knowledge on the valuable ‘GMMA technology’ to design and produce effective vaccines, might probably make the difference in the long and challenging path to combat the most dangerous infectious diseases for the mankind and have a safer world for all people, ‘regardless of where they are born, who they are, or where they live’ (cit [Citation65]).
Article highlights
GMMA is a technology platform that can be used to design vaccines based on OMVs of Gram-negative bacteria.
GMMA is OMVs derived from bacteria that have been through several genetic manipulations, with the aim to obtain a fit-for-purpose OMV-based vaccine. For instance, the bacterium source of GMMA can be genetically engineered to: (A) enhance the vesicle release; (B) reduce the capacity of LPS to induce reactogenicity upon OMV injection; (C) delete antigens that can be detrimental for vaccine design; (D) up-regulate antigens considered optimal candidates to have a protective efficacy of the vaccine; (E) express heterologous antigens to design combination vaccines. GMMA can be obtained for vaccine manufacturing by applying a simple method for vesicle purification.
GMMA technology is particularly suited to the manufacturing of affordable vaccines, which makes this technology very useful to produce vaccines for low-income countries
The first GMMA-based vaccine in clinical development is against Shigella sonnei; it demonstrated a good immunogenic potential and safety profile. Moreover, the possibility to use GMMA to design combination vaccine can make a difference, as several infections considered a serious threat in poor countries overlap the area of endemicity.
The application of the chemical conjugation of proteic or polysaccharidic antigens to GMMA expands the potential to use this technology as a ‘plug and play’ tool to design multivalent combination vaccines.
In the coming future, it will be particularly important to elucidate the immunological mechanisms driving GMMA to promote an optimal immune response, because they can be instrumental to optimize the design of highly effective GMMA-based vaccines. An overview of these mechanisms is presented in the Expert Opinion.
Reviewer disclosures
Peer reviewers on this manuscript have no relevant financial or other relationships to disclose.
Declaration of Interests
D Piccioli, E Bartolini, and F Micoli are employees of the GSK group of companies. The authors have no other relevant affiliations or financial involvement with any organization or entity with a financial interest in or financial conflict with the subject matter or materials discussed in the manuscript apart from those disclosed.
Authorship
D Piccioli: conceived and wrote the manuscript interpreting the relevant literature, prepared the figures, and critically reviewed the manuscript.
E Bartolini: substantially contributed to the conception and design of the manuscript interpreting the relevant literature, prepared the figures and has been involved in the critical revision of the manuscript.
F Micoli: substantially contributed to the conception and design of the manuscript interpreting the relevant literature, prepared the figures and has been involved in the critical revision of the manuscript.
All authors approved the final version of the manuscript.
Acknowledgments
The authors thank Benn Reeve for the English revision of the manuscript.
Correction Statement
This article has been republished with minor changes. These changes do not impact the academic content of the article.
Additional information
Funding
References
- Gnopo YMD, Watkins HC, Stevenson TC, et al. Designer outer membrane vesicles as immunomodulatory systems - Reprogramming bacteria for vaccine delivery. Adv Drug Deliv Rev. 2017;114:132–142.
- Jan AT. Outer Membrane Vesicles (OMVs) of gram-negative bacteria: a perspective update. Front Microbiol. 2017;8:1053.
- van der Pol L, Stork M, van der Ley P. Outer membrane vesicles as platform vaccine technology. Biotechnol J. 2015;10(11):1689–1706.
- Chatterjee SN, Das J. Electron microscopic observations on the excretion of cell-wall material by Vibrio cholerae. J Gen Microbiol. 1967;49(1):1–11.
- McBroom AJ, et al. Outer membrane vesicle production by Escherichia coli is independent of membrane instability. J Bacteriol. 2006;188(15):5385–5392.
- Toyofuku M, Nomura N, Eberl L. Types and origins of bacterial membrane vesicles. Nat Rev Microbiol. 2019;17(1):13–24.
- Ellis TN, Kuehn MJ. Virulence and immunomodulatory roles of bacterial outer membrane vesicles. Microbiol Mol Biol Rev. 2010;74(1):81–94.
- Schooling SR, Beveridge TJ. Membrane vesicles: an overlooked component of the matrices of biofilms. J Bacteriol. 2006;188(16):5945–5957.
- Rumbo C, et al. Horizontal transfer of the OXA-24 carbapenemase gene via outer membrane vesicles: a new mechanism of dissemination of carbapenem resistance genes in Acinetobacter baumannii. Antimicrob Agents Chemother. 2011;55(7):3084–3090.
- Kadurugamuwa JL, Beveridge TJ. Virulence factors are released from Pseudomonas aeruginosa in association with membrane vesicles during normal growth and exposure to gentamicin: a novel mechanism of enzyme secretion. J Bacteriol. 1995;177(14):3998–4008.
- Li M, Zhou H, Yang C, et al. Bacterial outer membrane vesicles as a platform for biomedical applications: an update. J Control Release. 2020;323:253–268.
- Micoli F, MacLennan CA. Outer membrane vesicle vaccines. Semin Immunol. 2020;50:101433.
- Akira S, Uematsu S, Takeuchi O. Pathogen recognition and innate immunity. Cell. 2006;124(4):783–801.
- Iwasaki A, Medzhitov R. Regulation of adaptive immunity by the innate immune system. Science. 2010;327(5963):291–295.
- Kawai T, Akira S. The role of pattern-recognition receptors in innate immunity: update on Toll-like receptors. Nat Immunol. 2010;11(5):373–384.
- Mancini F, et al. OMV vaccines and the role of TLR Agonists in immune response. Int J Mol Sci. 2020;21(12).
- MacLennan CA. Vaccines for low-income countries. Semin Immunol. 2013;25(2):114–123.
- Tan K, Li R, Huang X, et al. Outer membrane vesicles: current status and future direction of these novel vaccine adjuvants. Front Microbiol. 2018;9:783.
- Bachmann MF, Jennings GT. Vaccine delivery: a matter of size, geometry, kinetics and molecular patterns. Nat Rev Immunol. 2010;10(11):787–796.
- Benne N, van Duijn J, Kuiper J, et al. Orchestrating immune responses: how size, shape and rigidity affect the immunogenicity of particulate vaccines. J Control Release. 2016;234:124–134.
- Moyer TJ, Zmolek AC, Irvine DJ. Beyond antigens and adjuvants: formulating future vaccines. J Clin Invest. 2016;126(3):799–808.
- Holst J, Martin D, Arnold R, et al. Properties and clinical performance of vaccines containing outer membrane vesicles from Neisseria meningitidis. Vaccine. 2009;27(Suppl 2):B3–12.
- Rappuoli R, Pizza M, Masignani V, et al. Meningococcal B vaccine (4CMenB): the journey from research to real world experience. Expert Rev Vaccines. 2018;17(12):1111–1121.
- Toneatto D, Pizza M, Masignani V, et al. Emerging experience with meningococcal serogroup B protein vaccines. Expert Rev Vaccines. 2017;16(5):433–451.
- O’Ryan M, Stoddard J, Toneatto D, et al. A multi-component meningococcal serogroup B vaccine (4CMenB): the clinical development program. Drugs. 2014;74(1):15–30.
- Pizza M, Scarlato V, Masignani V, et al. Identification of vaccine candidates against serogroup B meningococcus by whole-genome sequencing. Science. 2000;287(5459):1816–1820.
- Serruto D, Bottomley MJ, Ram S, et al. The new multicomponent vaccine against meningococcal serogroup B, 4CMenB: immunological, functional and structural characterization of the antigens. Vaccine. 2012;30(Suppl 2):B87–97.
- Nieves W, Petersen H, Judy BM, et al. A Burkholderia pseudomallei outer membrane vesicle vaccine provides protection against lethal sepsis. Clin Vaccine Immunol. 2014;21(5):747–754.
- Liu Q, et al. Outer membrane vesicles from flagellin-deficient Salmonella enterica serovar Typhimurium induce cross-reactive immunity and provide cross-protection against heterologous Salmonella challenge. Sci Rep. 2016;6(1):34776.
- Camacho AI, de Souza J, Sánchez-Gómez S, et al. Mucosal immunization with Shigella flexneri outer membrane vesicles induced protection in mice. Vaccine. 2011;29(46):8222–8229.
- Mitra S, Chakrabarti MK, Koley H. Multi-serotype outer membrane vesicles of Shigellae confer passive protection to the neonatal mice against shigellosis. Vaccine. 2013;31(31):3163–3173.
- Roier S, Leitner DR, Iwashkiw J, et al. Intranasal immunization with nontypeable Haemophilus influenzae outer membrane vesicles induces cross-protective immunity in mice. PLoS One. 2012;7(8):e42664.
- Bishop AL, Schild S, Patimalla B, et al. Mucosal immunization with vibrio cholerae outer membrane vesicles provides maternal protection mediated by antilipopolysaccharide antibodies that inhibit bacterial motility. Infect Immun. 2010;78(10):4402–4420.
- Schild S, Nelson EJ, Camilli A. Immunization with Vibrio cholerae outer membrane vesicles induces protective immunity in mice. Infect Immun. 2008;76(10):4554–4563.
- Sedaghat M, Siadat SD, Mirabzadeh E, et al. Evaluation of antibody responses to outer membrane vesicles (OMVs) and killed whole cell of vibrio cholerae O1 El Tor in immunized mice. Iran J Microbiol. 2019;11(3):212–219.
- Zhang X, Yang F, Zou J, et al. Immunization with Pseudomonas aeruginosa outer membrane vesicles stimulates protective immunity in mice. Vaccine. 2018;36(8):1047–1054.
- Zhao K, Deng X, He C, et al. Pseudomonas aeruginosa outer membrane vesicles modulate host immune responses by targeting the Toll-like receptor 4 signaling pathway. Infect Immun. 2013;81(12):4509–4518.
- Lee WH, Choi H-I, Hong S-W, et al. Vaccination with Klebsiella pneumoniae-derived extracellular vesicles protects against bacteria-induced lethality via both humoral and cellular immunity. Exp Mol Med. 2015;47(9):e183.
- Wu G, Ji H, Guo X, et al. Nanoparticle reinforced bacterial outer-membrane vesicles effectively prevent fatal infection of carbapenem-resistant Klebsiella pneumoniae. Nanomedicine. 2020;24:102148.
- Bottero D, Gaillard ME, Zurita E, et al. Characterization of the immune response induced by pertussis OMVs-based vaccine. Vaccine. 2016;34(28):3303–3309.
- Gaillard ME, Bottero D, Errea A, et al. Acellular pertussis vaccine based on outer membrane vesicles capable of conferring both long-lasting immunity and protection against different strain genotypes. Vaccine. 2014;32(8):931–937.
- Raeven RH, et al. Immunoproteomic profiling of bordetella pertussis outer membrane vesicle vaccine reveals broad and balanced humoral immunogenicity. J Proteome Res. 2015;14(7):2929–2942.
- Pierson T, et al. Proteomic characterization and functional analysis of outer membrane vesicles of Francisella novicida suggests possible role in virulence and use as a vaccine. J Proteome Res. 2011;10(3):954–967.
- Ferrari G, et al. Outer membrane vesicles from group B Neisseria meningitidis delta gna33 mutant: proteomic and immunological comparison with detergent-derived outer membrane vesicles. Proteomics. 2006;6(6):1856–1866.
- van de Waterbeemd B, Mommen GPM, Pennings JLA, et al. Quantitative proteomics reveals distinct differences in the protein content of outer membrane vesicle vaccines. J Proteome Res. 2013;12(4):1898–1908.
- Rosenqvist E, et al. Effect of aluminium hydroxide and meningococcal serogroup C capsular polysaccharide on the immunogenicity and reactogenicity of a group B Neisseria meningitidis outer membrane vesicle vaccine. Dev Biol Stand. 1998;92:323–333.
- Shah RR, Hassett KJ, Brito LA. Overview of vaccine adjuvants: introduction, history, and current status. Methods Mol Biol. 2017;1494:1–13.
- Colaprico A, Senesi S, Ferlicca F, et al. Adsorption onto aluminum hydroxide adjuvant protects antigens from degradation. Vaccine. 2020;38(19):3600–3609.
- Kis Z, Shattock R, Shah N, et al. Emerging technologies for low-cost, rapid vaccine manufacture. Biotechnol J. 2019;14(1):e1800376.
- Berlanda Scorza F, Colucci AM, Maggiore L, et al. High yield production process for Shigella outer membrane particles. PLoS One. 2012;7(6):e35616.
- Gerke C, Colucci AM, Giannelli C, et al. Production of a Shigella sonnei vaccine based on Generalized Modules for Membrane Antigens (GMMA), 1790GAHB. PLoS One. 2015;10(8):e0134478.
- Bernadac A, Gavioli M, Lazzaroni J-C, et al. Escherichia coli tol-pal mutants form outer membrane vesicles. J Bacteriol. 1998;180(18):4872–4878.
- Kulp AJ, Sun B, Ai T, et al. Genome-wide assessment of outer membrane vesicle production in Escherichia coli. PLoS One. 2015;10(9):e0139200.
- Mitra S, Sinha R, Mitobe J, et al. Development of a cost-effective vaccine candidate with outer membrane vesicles of a tolA-disrupted Shigella boydii strain. Vaccine. 2016;34(15):1839–1846.
- Roier S, Zingl FG, Cakar F, et al. A novel mechanism for the biogenesis of outer membrane vesicles in Gram-negative bacteria. Nat Commun. 2016;7(1):10515.
- Turner L, Praszkier J, Hutton ML, et al. Increased outer membrane vesicle formation in a helicobacter pylori tolB mutant. Helicobacter. 2015;20(4):269–283.
- Gerritzen MJH, Salverda MLM, Martens DE, et al. Spontaneously released Neisseria meningitidis outer membrane vesicles as vaccine platform: production and purification. Vaccine. 2019;37(47):6978–6986.
- Gerritzen MJH, Stangowez L, van de Waterbeemd B, et al. Continuous production of Neisseria meningitidis outer membrane vesicles. Appl Microbiol Biotechnol. 2019;103(23–24):9401–9410.
- Park BS, Song DH, Kim HM, et al. The structural basis of lipopolysaccharide recognition by the TLR4-MD-2 complex. Nature. 2009;458(7242):1191–1195.
- Schromm AB, Brandenburg K, Loppnow H, et al. Biological activities of lipopolysaccharides are determined by the shape of their lipid A portion. Eur J Biochem. 2000;267(7):2008–2013.
- Rossi O, Caboni M, Negrea A, et al. Toll-like receptor activation by generalized modules for membrane antigens from lipid a mutants of Salmonella enterica serovars typhimurium and enteritidis. Clin Vaccine Immunol. 2016;23(4):304–314.
- Rossi O, Pesce I, Giannelli C, et al. Modulation of endotoxicity of Shigella generalized modules for membrane antigens (GMMA) by genetic lipid A modifications: relative activation of TLR4 and TLR2 pathways in different mutants. J Biol Chem. 2014;289(36):24922–24935.
- Rappuoli R, Black S, Bloom DE. Vaccines and global health: in search of a sustainable model for vaccine development and delivery. Sci Transl Med. 2019;11(497).
- WHO. 2009. State of the world’s vaccines and immunization. 3rd ed.
- WHO, Global vaccine action plan 2011-2020. 2013.
- WHO. Weekly epidemiological record. WHO position papers; 2004.
- Hosangadi D, Smith PG, Kaslow DC, et al. WHO consultation on ETEC and Shigella burden of disease, Geneva, 6-7th April 2017: meeting report. Vaccine. 2019;37(50):7381–7390.
- Liu J, Platts-Mills JA, Juma J, et al. Use of quantitative molecular diagnostic methods to identify causes of diarrhoea in children: a reanalysis of the GEMS case-control study. Lancet. 2016;388(10051):1291–1301.
- Balasubramanian R, Im J, Lee J-S, et al. The global burden and epidemiology of invasive non-typhoidal Salmonella infections. Hum Vaccin Immunother. 2019;15(6):1421–1426.
- Bekker LG, Tatoud R, Dabis F, et al. The complex challenges of HIV vaccine development require renewed and expanded global commitment. Lancet. 2020;395(10221):384–388.
- Haselbeck AH, Panzner U, Im J, et al. Current perspectives on invasive nontyphoidal Salmonella disease. Curr Opin Infect Dis. 2017;30(5):498–503.
- Jones LD, Moody MA, Thompson AB. Innovations in HIV-1 Vaccine Design. Clin Ther. 2020;42(3):499–514.
- Tennant SM, MacLennan CA, Simon R, et al. Nontyphoidal salmonella disease: current status of vaccine research and development. Vaccine. 2016;34(26):2907–2910.
- WHO, First malaria vaccine in Africa. 2019.
- WHO, Global tuberculosis report 2019. 2019.
- Bloom DE, et al. Antimicrobial resistance and the role of vaccines. Proc Natl Acad Sci U S A. 2018;115(51):12868–12871.
- CDC, Antibiotic resistance threats in the United States, 2019. 2019.
- ECDC, Surveillance of antimicrobial resistance in Europe 2018. 2019.
- WHO, Global action plan on antimicrobial resistance. 2015.
- Micoli F, Bagnoli F, Rappuoli R, et al. The role of vaccines in combatting antimicrobial resistance. Nat Rev Microbiol. 2021;19(5):287–302.
- Launay O, Lewis DJM, Anemona A, et al. Safety profile and immunologic responses of a novel vaccine against Shigella sonnei administered intramuscularly, intradermally and intranasally: results from two parallel randomized phase 1 clinical studies in healthy adult volunteers in Europe. EBioMedicine. 2017;22:164–172.
- Launay O, Ndiaye AGW, Conti V, et al. Booster vaccination with GVGH Shigella sonnei 1790GAHB GMMA vaccine compared to single vaccination in unvaccinated healthy European adults: results FROM A PHASE 1 CLINICAL TRIAL. Front Immunol. 2019;10:335.
- Obiero CW, Ndiaye AGW, Sciré AS, et al. A phase 2a randomized study to evaluate the safety and immunogenicity of the 1790GAHB generalized modules for membrane antigen vaccine against Shigella sonnei administered intramuscularly to adults from a Shigellosis-endemic country. Front Immunol. 2017;8:1884.
- Micoli F, Rossi O, Conti V, et al. Antibodies elicited by the Shigella sonnei GMMA vaccine in adults trigger complement-mediated serum bactericidal activity: results from a phase 1 dose escalation trial followed by a booster extension. Front Immunol. 2021;12:671325.
- Mani S, Wierzba T, Walker RI. Status of vaccine research and development for Shigella. Vaccine. 2016;34(26):2887–2894.
- Frenck RW Jr., et al. Efficacy, safety, and immunogenicity of the Shigella sonnei 1790GAHB GMMA candidate vaccine: results from a phase 2b randomized, placebo-controlled challenge study in adults. EClinicalMedicine. 2021;39:101076.
- Micoli F, et al. GMMA is a versatile platform to design effective multivalent combination vaccines. Vaccines (Basel). 2020;8(3.
- Berti F, Micoli F. Improving efficacy of glycoconjugate vaccines: from chemical conjugates to next generation constructs. Curr Opin Immunol. 2020;65:42–49.
- Broker M, Costantino P, DeTora L, et al. Biochemical and biological characteristics of cross-reacting material 197 CRM197, a non-toxic mutant of diphtheria toxin: use as a conjugation protein in vaccines and other potential clinical applications. Biologicals. 2011;39(4):195–204.
- Micoli F, Adamo R, Costantino P. Protein carriers for glycoconjugate vaccines: history, selection criteria, characterization and new trends. Molecules. 2018;23(6).