ABSTRACT
Introduction
Vaccines based on multiple antigens often induce an immune response, which is higher than that triggered by each single component, with antibodies acting cooperatively and synergistically in tackling the infection.
Areas covered
An interesting example is the antibody response induced by the 4CMenB vaccine, currently licensed for the prevention of Neisseria meningitidis serogroup B (MenB). It contains four antigenic components: Factor H binding protein (fHbp), Neisseria adhesin A (NadA), Neisserial Heparin Binding Antigen (NHBA), and Outer Membrane Vesicles (OMV). Monoclonal and polyclonal antibodies raised by vaccination with 4CMenB show synergistic activity in complement-dependent bacterial killing. This review summarizes published and unpublished data and provides evidence of the added value of multicomponent vaccines.
Expert opinion
The ability of 4CMenB vaccine to elicit antibodies targeting multiple surface-exposed antigens is corroborated by the recent data on real-world evidences. Bactericidal activity is generally mediated by antibodies that bind to antigens highly expressed on the bacterial surface and immunologically related. However, simultaneous binding of antibodies to various surface-exposed antigens can overcome the threshold density of antigen–antibody complexes needed for complement activation. The data discussed in this review highlight the interplay between antibodies targeting major and minor antigens and their effect on functionality.
Clinical trial registration
www.clinicaltrials.gov identifiers of studies with original data mentioned in the article: NCT00937521, NCT00433914, NCT02140762, and NCT02285777.
1. Introduction
Neisseria meningitidis is an encapsulated Gram-negative diplococcus and is the major cause of septicemia and meningitis in all age groups, with the highest disease incidence in young infants and a second incidence peak in adolescents. Six serogroups of Neisseria meningitidis (Men), A, C, W, Y, X and B, account together for more than 99% of invasive meningococcal disease (IMD) [Citation1]. Nowadays, MenA, MenC, and MenACWY capsular polysaccharide conjugated vaccines are available and their use has been successful in reducing the prevalence of IMD due to these serogroups worldwide [Citation2]. In contrast, the similarity of the group B polysaccharide capsule to the sialic acid found in human neural tissue makes it an unsuitable vaccine antigen. Indeed, concerns were mainly due to its poor immunogenicity and potential autoimmunity [Citation3]. As an alternative strategy for MenB vaccine development, Outer Membrane Vesicle (OMV) preparations have been developed, manufactured, and successfully used to fight outbreaks in Norway, Cuba, Chile, Brazil, Normandy, and New Zealand [Citation4–9]. Although these tailor-made vaccines showed good efficacy in humans and were able to control outbreaks, they did not induce cross-protection against heterologous strains [Citation4]. This was due to the high variability of the Porin A (PorA), which is one of the most abundant outer membrane protein (OMP) and the major protective antigen in OMV-based vaccines [Citation10,Citation11]. To overcome these limitations protein-based vaccines have hence been developed. To date, two protein-based vaccines against MenB have been licensed in many countries worldwide. The four-component MenB vaccine (4CMenB; Bexsero, GSK, Siena, Italy) is the only vaccine licensed for the active immunization in infants and older, starting from 2 months of age, while in the United States (US) it is licensed in 10–25 years-old only, as a two-dose schedule. The bivalent rLP2086 (Trumenba, Pfizer, Philadelphia, PA, USA) is licensed both in the US and in Europe as a two- or three-dose schedule only for 10–25-year-old individuals. The rLP2086 vaccine is composed of two lipidated forms of the fHbp antigen identified by a biochemical approach, which belong to subfamilies A and B or to variants 1.55 and 3.45 according to the classification used [Citation12]. 4CMenB contains three main antigens (fHbp variant 1.1, NadA variant 3, and NHBA peptide 2) identified by ‘reverse vaccinology’ in combination with the OMV from the New Zealand epidemic strain harboring the immunodominant PorA (P1.7–2,4) [Citation13–15].
Both rLP2086 and 4CMenB vaccines induce antibodies with functional activity able to recognize the protein targets on the bacterial surface and to activate complement-mediated bacterial killing. Nevertheless, the multicomponent nature of the 4CMenB vaccine enables the simultaneous binding of antibodies raised by multiple antigens to the different antigenic targets. Therefore, the bactericidal activity is mediated not only by antibodies directed against each major specific target antigen but also by the cooperativity and synergy of antibodies recognizing different antigens, including those present in the OMV component. In this review, we will present all data supporting the added value of 4CMenB multicomponent vaccine.
2. Measurement of vaccine immunogenicity and coverage for the 4CMenB vaccine
Multiple assays can be applied to measure strain coverage mediated by vaccination with the 4CMenB vaccine (). The serum bactericidal antibody assay (SBA) with human complement (hSBA) has been established as an in vitro surrogate of meningococcal vaccine protection and is now routinely used to support meningococcal vaccine licensure [Citation16]. Contrary to meningococcal capsular polysaccharides, which are highly conserved, protein antigens are variable. Due to the diversity of disease-causing isolates, the immunogenicity of protein-based vaccines should be ideally assessed in hSBA on multiple strains representative of the antigenic variability and levels of expression. Unfortunately, this approach is labor-intensive and is impractical due to the difficulty in finding seronegative exogenous human complement source and, especially for infants, due to the very limited amount of sera available for testing [Citation17]. To overcome these limitations, in the case of 4CMenB, vaccine immunogenicity and strain coverage are assessed by two independent assays. Immunogenicity is measured using four strains representative of the four main vaccine antigens, whose bacterial lysis is known to be mainly mediated by antibodies induced by each single vaccine component. These ‘indicator’ strains were isolated from cases of IMD and are used in hSBA assays to prove that each of the vaccine antigens is able to induce a functional immune response in clinical studies [Citation18]. Strain coverage is instead assessed by the Meningococcal Antigen Typing System (MATS) which combines conventional genotyping of PorA with a specialized sandwich ELISA that measures the levels of expression of fHbp, NadA, and NHBA proteins in a given meningococcal isolate and their immunological cross-reactivity with the corresponding vaccine antigen [Citation19]. A strain is predicted to be covered by the 4CMenB vaccine when the MATS value for one of the recombinant vaccine components is equal or higher than a positive bactericidal threshold (PBT) or when it matches the PorA peptide [Citation19]. MATS is a valuable tool currently used to predict 4CMenB coverage in individual countries using panels of epidemiologically representative strains [Citation20]. Nevertheless, many strains that are not predicted to be covered on the basis of MATS, are killed in the hSBA by infant and adolescent vaccinees sera suggesting that this method is conservative and underestimates strain coverage [Citation21–23]. This type of underestimation affects also new genetic methods like BAST (Bexsero Antigen Sequence Typing), gMATS (Genetic MATS), and more recently MenDeVAR (Meningococcal Deduced Vaccine Antigen Reactivity), which are all based on the genotyping of the target antigens included in 4CMenB (fHbp, NHBA, NadA and PorA). In the case of gMATS, the prediction of coverage for a given peptide is driven by the association of antigen genotyping with MATS results. All these methods represent a very powerful tool to monitor epidemiological changes and predict vaccine coverage also for non-cultivable isolates to which MATS cannot be applied [Citation24,Citation25]; however, similarly to MATS, they are not able to measure the contribution to bacterial killing mediated by the simultaneous binding of antibodies to multiple antigenic targets, which can lead to complement activation and bactericidal killing (). Due to the multiple combinations of synergistic mechanisms, this underestimation mainly affects multicomponent vaccines, as 4CMenB.
Figure 1. The differences between the three methods of vaccine coverage estimation are illustrated. When an antigen is highly expressed on the bacterial strain and it is antigenically related to the vaccine antigen, the strain is predicted to be covered by MATS, gMATS, or MenDeVAR and killed in the bactericidal assay (Strain A). When an antigen is present at low amounts on the bacterial strain or is antigenically diverse to the vaccine ones, the strain is not predicted covered and the number of antibodies bound on bacterial surface are not sufficient to induce bactericidal killing (Strain B). However, when multiple antigens are present on a bacterial strain even if their diversity and expression levels are suboptimal and the strain is not considered covered by MATS, gMATS/MenDeVAR, the antibodies targeting multiple antigens can bind simultaneously and contribute synergistically to complement activation and bacterial killing (Strain C).
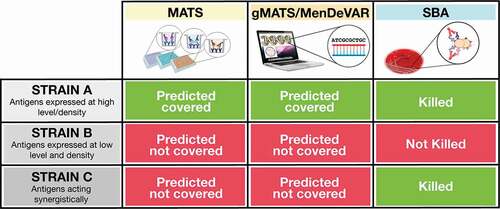
3. OMV contribution to 4CMenB coverage
Early clinical 4CMenB trials in infants were performed comparing two formulations: one containing only the recombinant meningococcal antigens NadA, NHBA, and fHbp (rMenB), the other containing the same antigens in combination with the New Zealand OMV (rMenB + OMV). The deriving sera were tested for bactericidal activity against seven genetically diverse strains (). Results showed that only infants immunized with the vaccine containing OMV had antibodies inducing complement-mediated killing of the seven strains, including the two isolates carrying non-homologous 4CMenB antigens (M01 240101 and M01 240355), suggesting that antibodies raised by the OMV component contribute to broaden the strain coverage () [Citation26,Citation27].
Figure 2. hSBA data of 4CMenB vaccinee sera on a panel of 7 MenB strains. Histograms show the percentage of participants achieving hSBA titer ≥4 after the final dose of rMenB (NadA, NHBA and fHbp – dark gray) or rMenB + OMV (light gray) vaccine.
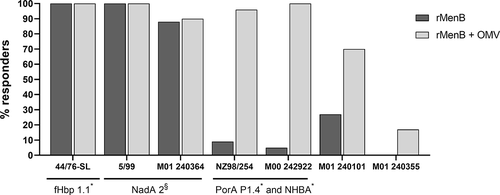
Further insights on the OMV contribution to 4CMenB strain coverage are derived from a dose-ranging and formulation-finding clinical study in which formulations containing recombinant meningococcal antigens alone (rMenB) or in combination with 25 µg or 6.25 µg of OMV were evaluated in 2-month-old infants (NCT00937521). All antigens in the different formulations elicited complement-mediated bacterial killing against the four indicator strains tested [Citation28]. To further measure differences in the cross-protective ability of antibodies induced by the different formulations, a panel of 61 strains was tested in hSBA using the pooled sera of 25 infants that had received four doses of the three formulations containing no OMV, 6.25 or 25 µg of OMV. As shown in , the percentages of strains killed in the bactericidal assay with titers ≥4 were 51% (31/61), 80% (49/61), and 89% (54/61), respectively. It is noteworthy that the hSBA titers achieved were higher for the formulation containing OMV with respect to formulation containing MenB antigens only. Considering that 20 out of the 54 strains, mismatched for the PorA antigen, were killed only by formulations containing OMV, it is clear that antibodies against the minor OMV component, alone or in combination with antibodies targeting the recombinant antigens act cooperatively in inducing bacterial lysis. Overall, vaccine formulations with or without one-quarter amount of OMV performed reasonably well and by far had lower rates of local and systemic reactogenicity [Citation28]. Nevertheless, the lack or reduced amount of OMV had a significant impact of immunogenicity on heterologous strains (). The reactogenicity of 4CMenB in infants can be tempered by the prophylactic use of paracetamol at the time of immunization [Citation29].
Figure 3. hSBA titers obtained over a panel of 61 strains with pooled sera from infants vaccinated with different vaccine formulations. Infant sera were collected before and after the fourth vaccination dose and the sera were assayed as pool (25 subjects each group) in hSBA. Dots represent the SBA titer obtained for each of the 61 genetically different strains tested. Histograms show the geometric mean with 95% CI of SBA titers obtained using rMenB (cyan), rMenB + 6.25 µg OMV (light blue), rMenB + 25 µg OMV (dark blue) pooled antisera or the corresponding preimmune sera of each group (gray).
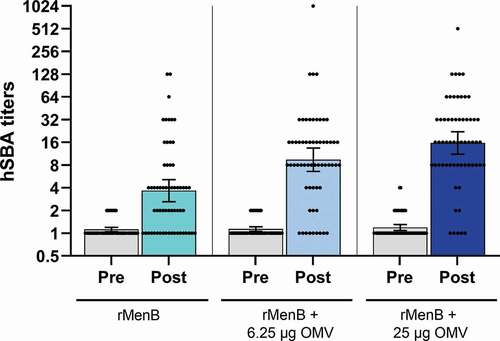
Multiple hypotheses could explain the enhanced cross-protection in sera from subjects receiving rMenB + OMV. Indeed, this effect could be due to (i) the synergy between antibodies targeting the main rMenB antigens and the minor-OMV antigens, (ii) the synergy between antibodies targeting the minor OMV antigens, (iii) the intrinsic immunostimulatory effect of OMV or by a combination of these factors.
4. Insights on the synergy between antibodies targeting rMenB antigens and OMV
As described above, the ability of each 4CMenB antigen to induce functional antibodies is assessed on four indicator strains, each of them killed by antibodies directed against one of the four vaccine antigens. These strains are used as immunogenicity indicator in clinical trials and in all age groups. In their work, Giuliani and coworkers measured the specificity of bactericidal activity against the four indicator strains on sera of subjects immunized with 4CMenB, which were pre-incubated with each of the major vaccine antigens to deplete the specific antibodies (competitive-SBA experiments) [Citation18]. Surprisingly, when tested against reference strains for the recombinant antigens (5/99 for NadA, H44/76 for fHbp, and M4407 for NHBA), four of the sera analyzed showed negative SBA only following depletion with recombinant antigens plus OMV (). Indeed, although for each strain the addition of the recombinant antigen depleted most of the functional antibodies resulting in a significant reduction in bactericidal killing, a complete inhibition was observed only when the relevant antigen was added in combination with OMV. The fact that OMV contains multiple outer membrane proteins in addition to PorA suggests that antibodies induced by non-PorA antigens may cooperate with antibodies directed against the recombinant antigens and contribute to bactericidal killing. Notably, the cooperative effect was more relevant on the NHBA-specific indicator strain, M4407. However, when the 5/99 strain was engineered to express higher amounts of NHBA compared to the M4407 isolate, the contribution to killing of anti-NHBA antibodies increased and became comparable to the effect of anti-NadA or anti-fHbp antibody depletion on the 5/99 and H44/76 strain, respectively. This result suggests that in case of highly expressed antigens, as for NadA in the 5/99 and fHbp in the H44/76 strains, the contribution to killing of antibodies targeting additional antigens is less measurable. In contrast, in case of strains in which antigens are expressed at lower levels, or are more antigenically diverse, the effect of antibodies targeting multiple antigens simultaneously is key to reach a threshold for complement mediated killing [Citation18].
Figure 4. Serum bactericidal titers using human complement against indicator strains with or without the addition of the specific competitor. All sera tested derived from four subjects vaccinated with 4CMenB. 5/99, H44/76 and M4407 strains were selected to assess the contribution of antibodies directed against NadA, fHbp, and NHBA, respectively. The 5/99 strain overexpressing NHBA was a deletion mutant for nadA and NHBA complemented with the NHBA gene under the control of an IPTG-inducible promoter. Green dots denote significant inhibition (≥ fourfold) of SBA by the addition of exogenous antigens compared to the untreated sera (initial titer). Orange dots describe mild inhibitions characterized by a twofold decrease of SBA titers compared to the initial titer. Red dots indicate no inhibition at all. Figure generated based on data discussed in Giuliani et al. [Citation18].
![Figure 4. Serum bactericidal titers using human complement against indicator strains with or without the addition of the specific competitor. All sera tested derived from four subjects vaccinated with 4CMenB. 5/99, H44/76 and M4407 strains were selected to assess the contribution of antibodies directed against NadA, fHbp, and NHBA, respectively. The 5/99 strain overexpressing NHBA was a deletion mutant for nadA and NHBA complemented with the NHBA gene under the control of an IPTG-inducible promoter. Green dots denote significant inhibition (≥ fourfold) of SBA by the addition of exogenous antigens compared to the untreated sera (initial titer). Orange dots describe mild inhibitions characterized by a twofold decrease of SBA titers compared to the initial titer. Red dots indicate no inhibition at all. Figure generated based on data discussed in Giuliani et al. [Citation18].](/cms/asset/833f9f69-48cc-4ccb-9795-31dbd1c22efc/ierv_a_2050697_f0004_oc.jpg)
In a similar work, Vu et al. assayed complement-mediated bactericidal activity in human subjects vaccinated with the three recombinant meningococcal antigens (no OMV) after depletion of antibodies directed to fHbp and/or NHBA [Citation30]. In four out of the six subjects analyzed, the removal of both anti-fHbp and anti-NHBA antibodies reduced bactericidal activity more than the depletion of either antibody individually. Moreover, mixing a mouse non-bactericidal anti-fHbp variant 1 antiserum with a mouse anti-NHBA antiserum augmented the anti-NHBA SBA titer against the tested strain. In another study, Partridge and colleagues measured the effect of depletion of anti-NHBA and/or anti-fHbp antibodies in sera of adults vaccinated with 4CMenB on the bactericidal activity against three meningococcal strains [Citation31]. Sera depleted of NHBA or fHbp antibodies had a reduction of bactericidal activity ranging between 43–79% and 45–64%, respectively. However, bactericidal activity decreased to 84–100% only when sera were simultaneously depleted of anti-NHBA and -fHbp antibodies, demonstrating that those specific antibodies may act cooperatively. Overall, these studies suggest that when antigens are relatively sparsely expressed on vaccine target strains, such as fHbp or NHBA, non-bactericidal antibodies against individual antigens may work in concert and cooperate triggering complement-mediated killing. To further test this hypothesis, we have analyzed the bactericidal activity of infant sera against 451 strains characterized for MATS. Among the 451 strains analyzed, 138 were not predicted to be covered by MATS and 64 strains of them were killed in bactericidal assay by 4CMenB infant sera. The negative MATS results suggest that antibody binding to a single antigen on the bacterial surface may not be effective to trigger complement activation and bacterial lysis, while the simultaneous binding can be. The killing of the 64 strains in SBA may be mediated by antibodies induced by the recombinant vaccine antigens and OMV minor proteins acting in synergy. Indeed, on the basis of the antigenic repertoire of these strains, we could envisage cooperativity between antibodies binding to OMV minor antigens and/or to the recombinant antigens, which, being expressed at low levels or too diverse in sequence, results to be MATS negative. In addition, clues about the cooperativity effect of antibodies derive from a study in which the Breadth of Coverage (BoC) was assessed in sera of adolescents vaccinated with an investigational MenABCWY vaccine (containing MenACWY conjugated to CRM197 combined with 4CMenB) using the endogenous complement as source (enc-hSBA), over a panel of 110 randomly selected serogroup B strains causing invasive disease in the US [Citation32]. The aim of this study was to estimate the vaccine ability to protect against meningococcal strains in a real-world setting, in an approximation of the vaccine’s effectiveness. Among the 110 strains, 25 of them not predicted to be covered by gMATS showed a BoC ranging between 4% and 92% (). These results further support the hypothesis of a cooperative antibody effect and suggest that predictors of MenB strain coverage based only on one antigen at time underestimate the full potential that the vaccine may have in real-world settings.
Figure 5. Strain-specific Breadth of Coverage obtained with the MenABCWY vaccine. Strain-specific BoC, calculated as (1 – relative risk) × 100 (relative risk = ratio between the percentage of samples seronegative at 1:4 dilution against the selected strains in the MenABCWY vs Control group), was determined for each of the 25 strains (not predicted to be covered by gMATS) out of 110 as measured by serum bactericidal activity. Figure realized from data described in Welsch et al. [Citation32].
![Figure 5. Strain-specific Breadth of Coverage obtained with the MenABCWY vaccine. Strain-specific BoC, calculated as (1 – relative risk) × 100 (relative risk = ratio between the percentage of samples seronegative at 1:4 dilution against the selected strains in the MenABCWY vs Control group), was determined for each of the 25 strains (not predicted to be covered by gMATS) out of 110 as measured by serum bactericidal activity. Figure realized from data described in Welsch et al. [Citation32].](/cms/asset/e5db418b-8480-43d2-81d8-92b92a3dec86/ierv_a_2050697_f0005_oc.jpg)
Finally, the contribution of 4CMenB antibodies targeting different antigens alone or simultaneously has also been shown on a panel of 147 MenC, MenY, and MenW isolates, of which 109 were killed in hSBA, resulting in an overall coverage of 74% [Citation33].
5. Insights on the role of OMV-minor antigens in protection
The protective effect of meningococcus B OMV-based vaccines is thought to be uniquely mediated by the immunodominant PorA protein, which is highly immunogenic, but also extremely antigenic variable. For this reason, these vaccines have been mainly used to fight outbreaks caused by a clone of a given PorA serosubtype and therefore they are generally defined as ‘tailor-made.’ However, looking retrospectively in the literature, several are the reports describing a moderate cross-protection of OMV-based vaccines. Almost 30 years ago, the OMV Cuban vaccine (VA-MENGOC-BC, CU385, B:4:P1.15 + MenC capsular polysaccharide) was shown to elicit bactericidal antibodies against a broader spectrum of MenB isolates than the solely vaccine-strain [Citation6]. These data were also corroborated by a case-control study performed in Sao Paolo, Brazil, where the OMV Cuban vaccine was administered to 2.4 million children (3 months to 6 years) in order to tackle a MenB outbreak dominated by multiple strains (only 44% of MenB isolates detected matched the vaccine-PorA-serosubtype) [Citation34]. In this study, similar protective efficacy was estimated against homologous and heterologous strains, although the number of cases was small and this inevitability limited the ability to draw significant conclusions [Citation34]. Recently, other data on support of cross-protection induced by VA-MENGOC-BC vaccine have been described in several clinical trials [Citation35]. Similar results were observed also for the Norwegian vaccine (MenBVac, H44/76, B:15:P1.7,16). Indeed, in a comparative immunogenicity study, Tappero and coworkers, reported the difference in the immune response among various age-groups (infants, children and adults) immunized with a two- or three-dose regimen of the Cuban or of the Norwegian OMV-vaccines ( A and B, respectively) [Citation4]. The percentage of responders was similar across the three age groups when the bactericidal activity was measured against the homologous vaccine strain, particularly in the case of MenBVac immunized subjects. In the case of the heterologous strains, a dramatic decrease in protection was observed for the infants with response rate in bactericidal activity decreasing to 10–31% and 2–12% for VA-MENGOC-BC- or MenBVac-immunized subjects, respectively. Interestingly, the seroresponse rate to heterologous strains was higher in children and adults suggesting a contribution of OMV antigens other than PorA in cross-protection, particularly in these age groups (). Nevertheless, results of these clinical studies have been evaluated considering the immunodominance of PorA especially in infants and young children, supporting the concept of the OMV-vaccine strain specificity. The evidence of a moderate cross-protection in the older age groups, which could be ascribed to the minor OMV antigen(s) that alone or in concert may increase the vaccine breadth of coverage, has been hence overlooked [Citation36,Citation37]. More recently, the OMV-MeNZB vaccine (MeNZB, NZ98/254, B:4:P1.7–2,4) was produced to fight a prolonged epidemic of serogroup B in New Zealand, causing from 1991 to 2006 around 6,000 IMD cases and 250 [Citation38]. The vaccine was used in a mass-immunization campaign (2004–2008) conducted on one million people. According to a study performed in the years 2001–2008 the MeNZB effectiveness on meningococcal B strains different from the PorA serosubtype of the clone-outbreak was estimated to be 54% (or 41% corrected for residual confounding), demonstrating protection beyond the PorA serosubtype [Citation38,Citation39]. These data were in line with the results of Wedege and colleagues which demonstrated that adults immunized with MeNZB or MenBVac could mount low titers of cross-reactive SBA responses against PorA-mismatched strains [Citation40].
Figure 6. hSBA data on homologous and heterologous vaccine-strains. Histograms represent the percentage of responders in the SBA (≥fourfold rise) in all the three age-groups with various target strains, after three doses of VA-MENGOC-BC (A) or MenBVac (B). The vaccine homologous strain is represented by dotted histograms. The degree of strain-specific response varies with ages. Data represented in this figure are derived from [Citation4,Citation36].
![Figure 6. hSBA data on homologous and heterologous vaccine-strains. Histograms represent the percentage of responders in the SBA (≥fourfold rise) in all the three age-groups with various target strains, after three doses of VA-MENGOC-BC (A) or MenBVac (B). The vaccine homologous strain is represented by dotted histograms. The degree of strain-specific response varies with ages. Data represented in this figure are derived from [Citation4,Citation36].](/cms/asset/621013fd-b068-4eb9-8fc2-7856090366a5/ierv_a_2050697_f0006_b.gif)
Collectively, all these findings strongly support the role of additional OMV-antigens on the top of PorA in inducing protection. The protective effect ascribed only to anti-PorA antibodies is rather reductive considering the potential role that OMV-minor antigens may play in protection. More recently, many studies have been performed to characterize more in-depth the immune responses elicited by OMV-vaccines. Mono- and bi-dimensional immunoblots and protein-array serum profiling studies applied to sera from people convalescent from meningococcal B disease as well as to sera from people immunized with OMV-containing vaccines have revealed the presence of multiple functional antibodies targeting different OMV antigens [Citation40–46]. Among the OMV antigens identified as possible contributors to protection there are: PorB (Porin B) the most abundant OMP present in the meningococcal OMV (about 42.5% of the total OMV-protein content [Citation10]) [Citation45–47]; TbpA and TbpB (transferrin binding Proteins A and B) the two components of a functional complex devoted to sequester iron from host transferrin [Citation48–53]; LbpA and LbpB (lactoferrin-binding proteins A and B) responsible for iron uptake from human lactoferrin [Citation54,Citation55]; FetA (ferric enterobactin transporter A) acting as an iron transporter [Citation56–58]; Opc (class 5 outer membrane protein) which mediates adhesion of N. meningitidis to epithelial and endothelial cells by binding to vitronectin and proteoglycan host cell-surface receptors [Citation40,Citation41,Citation59–63]; ACP (adhesin complex protein) involved in N. meningitidis adhesion to epithelial, endothelial, and meningeal cells [Citation64,Citation65]; Pili (PilQ, PilE) which facilitate adhesion to host tissues [Citation66,Citation67]; RmpM (reduction modifiable protein M) involved in the bacterial structural maintenance anchoring the outer membrane to the underlying peptidoglycan layer [Citation43,Citation68,Citation69]; Omp85 (outer membrane protein 85 kDa) essential for folding and localization of OMP [Citation70,Citation71]; NspA (Neisserial surface protein A) still with an unknown role, but able to bind to the human factor H and hence to regulate the alternative complement pathway enhancing bacterial survival and serum-resistance [Citation10,Citation72–77]; MIP (macrophage infectivity potentiator) [Citation78–80] and the outer membrane protein P1 (NMB0088) [Citation81].
The role of minor OMV-antigens in protection has also been investigated at the preclinical level. To overcome the PorA-restricted protection of wild-type OMV, Weynants and coworkers engineered the H44/76 meningococcal strain to eliminate the expression of PorA, to remove the polysaccharide B capsule and to overproduce minor well-conserved outer membrane proteins (TbpA, NspA, Omp85, and NhhA, an autotransporter homologous to Hsf of Haemophilus influenzae), known to induce a protective response. OMV derived from this strain were used to immunize mice. The authors observed that one single minor OMP among TbpA, Omp85, NspA, and NhhA was not sufficient to induce antibodies with bactericidal activity; however, when antibodies against at least two of these minor OMP were combined they became bactericidal. The bactericidal titers induced by the combination of antibodies directed against more than one target were higher than those induced by antibodies targeting one single antigen, suggesting a synergistic effect in bactericidal killing. The authors demonstrated that some minor OMP, being sparsely distributed on the bacterial surface, were not dense enough and antibodies targeting each of them were not able to reach the threshold needed to mediate complement activation and bactericidal killing. In contrast, the threshold could be only reached by the simultaneous binding of antibodies to more than one antigen [Citation70]. The resulting mechanism proposed by the authors is that at least two adjacent Fc, not necessarily targeting the same antigen, are required to activate the complement cascade and induce bacterial killing [Citation70].
Various clinical trials have been performed to show the contribution of OMV minor antigens in protection. Marsay et al. described a phase I clinical trial with an OMV-vaccine (MenPF-1) from a N. meningitidis strain engineered to constitutively express FetA (NCT01640652). Following a three-dose schedule, the proportion of volunteers with a protective hSBA titer against the wild-type strain was 98%. Instead, the bactericidal activity against isogenic mutant strains, generated to measure the individual contribution of FetA and PorA, was lower suggesting that the bactericidal activity of the MenPF-1 vaccine is mediated by anti FetA and PorA antibodies acting simultaneously [Citation58]. However, it is worth noting that the immunogenicity profiling of sera from MenPF-1 vaccinees did not reveal FetA among the highest responding antigens following vaccination [Citation46]. Another phase I clinical trial was instead performed to evaluate the immunogenicity of a native outer membrane vesicle vaccine derived from the 44/76 strain mutated in the lpx gene to detoxify LPS (lpxL2-), in the synX gene to remove the capsule (synX-) and with opcA expression stabilized. Following three doses, 12 out of the 26 volunteers developed at least a fourfold increase in serum bactericidal activity when immunized with the vaccine strain with high opcA expression. Bactericidal depletion assays on sera from volunteers with high bactericidal titers suggest a major contribution of anti-OpcA and anti-lipooligosaccharide (LOS) antibodies to the bactericidal response [Citation82]. In a following study, the same research group performed additional mutations to the lpxL2- and synX- strain in order to enhance the expression of fHbp variant 1, to stabilize the expression of OpcA and to introduce a second PorA (P1.22,14). The resulting OMV were then tested in a phase I trial. After three doses of the OMV-vaccine, a fourfold or greater increase in bactericidal activity against the homologous strain was observed with sera from 27 out of the 34 volunteers while cross-bactericidal responses ranged from the 41% to the 82% of subjects, depending on the strains tested. Moreover, bactericidal depletion assays showed the contribution to the overall bactericidal activity of antibodies against LOS, fHbp v1, and OpcA [Citation83]. An intriguing evidence of the role played by OMV minor antigens derived also from a retrospective case–control study in which individuals vaccinated with OMV MeNZB vaccine were significantly less likely to contract gonorrhea compared with unvaccinated controls, with a predicted vaccine efficacy of 31% [Citation84]. Considering that the porA in N. gonorrhoeae is a pseudogene and it is hence not expressed, these results shed light on the pivotal role of additional OMV-antigens also in the cross-species protection. In agreement with this, Western blot analyses carried out with sera from 4CMenB immunized individuals revealed many gonococcal proteins, highlighting a high cross-reactivity between the two species and suggesting a contribution of non-PorA antigens in protection against gonococcal infection. In the same study, high cross-reactivity was detected also against the NHBA antigen, suggesting that the 4CMenB vaccine may have an even higher protective activity against gonococcal infection than a solely OMV-based vaccine [Citation85].
6. Synergistic activity of monoclonal antibodies targeting 4CMenB antigens
Another interesting aspect of the 4CMenB-mediated immune response is the synergistic activity described for antibodies mapping different epitopes on the same antigen. Non-bactericidal antibodies can cooperate and elicit serum bactericidal activity when used in combination. Murine monoclonal anti-fHbp antibodies, JAR5 and 12C1, can efficiently trigger the C1q engagement and the activation of the classical complement pathway, when binding simultaneously [Citation86–88]. The model proposed implies that bactericidal killing by anti-fHbp monoclonal antibodies against strains expressing low levels of fHbp requires the binding to two non-overlapping epitopes and/or the inhibition of human factor H binding by one of the two antibodies, resulting in an increase in the susceptibility of the meningococcus to complement-mediated bacteriolysis [Citation86]. Biagini et al. performed an absolute quantification of the fHbp molecules on the bacterial cell to identify the critical threshold of fHbp required for bacterial killing [Citation89]. In addition, the authors determined the optimal distance between the fHbp antigens required for C1q engagement and consequent complement activation. C1q binds a single Fc segment with very low affinity and therefore triggering the complement cascade requires C1q to bind at least two immunoglobulins [Citation90]. Biagini et al. showed that a minimum of 757 molecules per cell were needed to properly activate complement [Citation89]. However, optimal killing was only triggered by more than thousands of molecules per cell. The distance between the two antibodies must be between 33 nm to 115 nm, which can be achieved with a density of 3,930 and 757 fHbp molecules per cell, respectively. New insights about synergies were also provided by Giuliani et al. which have isolated human monoclonal antibodies (mAbs) directly from subjects vaccinated with 4CMenB [Citation91]. In this paper, the authors showed that many of the monoclonal antibodies targeting each of the 4CMenB recombinant proteins and recognizing non-overlapping epitopes on the same antigens became bactericidal with human complement only when used in combination. Electron microscopy analysis of the mAb-fHbp-mAb cooperative complex, supported by surface plasmon resonance data, showed that cooperative human mAbs could form very stable quaternary complexes with fHbp. The complex assumed an overall rhomboid-shaped architecture with two fHbp molecules at two opposite vertices of the rhomboid and the two cooperative mAbs at the other two opposite vertices [Citation92]. This analysis supports the hypothesis that the density of the target antigen on the bacterial surface and the distance between the epitopes (16–20 Å) are important requirements for efficient complement activation and killing. Lastly, synergistic effect between monoclonal antibodies targeting different antigens has been shown by Natali and colleagues who demonstrated that mAbs recognizing fHbp and NHBA can act in concert to activate complement even when each single mAb is not bactericidal. In this study the authors showed that the synergistic effect of antibodies is modulated by the nature of the respective epitopes as well as by the antigen density on the bacterial cell surface [Citation93].
7. Proposed mechanism of cooperativity, synergy, and complement engagement
The mechanism of SBA relies on the activation of the complement classical pathway (CP). In particular, the outcome of the SBA assay is influenced by multiple factors: the concentration of the complement and of the bactericidal antibodies in the serum sample, the avidity of the antigen-specific antibody binding, and finally the density and the levels of expression of the targeted antigen on the bacterial surface. Indeed, the CP begins when the density of antigen–antibody complexes is enough to enable the proximity of the Fc regions of immunoglobulins, which in turn recruit the C1q present in the C1 complex. This binding triggers the activation of the entire complement pathway that ultimately leads to bacteriolysis.
For some multicomponent vaccines, like 4CMenB, the vaccination elicits the simultaneous production of a wide array of antibodies that overall contribute to the killing of different meningococcal isolates, although acting in a strain-specific fashion as schematically represented in . Indeed, every meningococcal strain can potentially express a plethora of qualitatively and quantitatively different surface antigens that can differently act as decoy for antibodies. Depending on the expression levels/density of specific antigens on the strain, the antibodies elicited against single proteins are or are not able to recruit complement factors ( A and B, respectively). However, in multicomponent vaccines, the presence of multiple antibodies with different recognition specificity significantly enhances the possibility to achieve protection even in the presence of suboptimal levels of expression and/or density of antigens. Indeed, antigens expressed simultaneously may be recognized by specific antibodies that could cooperate resulting in C1q recruitment and bacterial lysis by overcoming the density threshold required (). By acting in concert, these different antibodies mimic the density threshold reached when one single antigen is expressed at high levels/density onto bacterial surface. Sometimes, it can also occur that even the simultaneous binding of multiple antigens might not be enough to activate complement-mediated killing (). The model proposed here may explain why positive bactericidal results can be achieved even in case of strains which resulted not covered by MATS and gMATS.
Figure 7. Schematic representation of the possible mechanisms of C1q engagement following vaccination with multicomponent vaccines. Upon vaccination with a multicomponent vaccine formulation a plethora of different antibodies are simultaneously elicited. They can differently trigger complement cascade relying on the antigen density profile and the expression levels of each strain. In the proposed model a defined density and/or a defined level of expression of each antigen are required to trigger the killing mediated by complement. Here, the threshold density/levels of expression is represented by five or six antigens per cell that can be both identical or dissimilar (A and C). On the contrary, the presence of three molecules per cell is schematically represented as not dense enough to recruit C1q (B and D). In particular, an antigen can be constitutively highly expressed in bacterial isolates leading to highly dense antigen–antibody complexes able to recruit C1q and to lead to bacterial lysis (e.g. filled pink circles, (A)). Generally, the amount and the distribution profile of an antigen can also change between isolates influencing the SBA outcome (e.g. filled blue triangle, A and B). Moreover some determinants may be expressed at very low levels on bacterial surface (eg strains expressing only open pink circles or open blue triangles (B) and strains simultaneously expressing different antigens but at low density/levels, (D) leading to only few IgG molecules sparsely bound on the bacterial cell surface. This scattered profile is insufficient to trigger the activation of the C1 complement complex. Nevertheless, strains can also co-express multiple antigens onto their surface at low levels but at high density (e.g. open pink circles, open blue triangles and filled blue triangle, (C). They can act as a co-target of antibodies being able to work in concert with sub-bactericidal levels of antibodies which ultimately result in bacterial killing. The minimal threshold required for IgG/cell to achieve C1 complex recruitment would therefore be easily met in this condition.
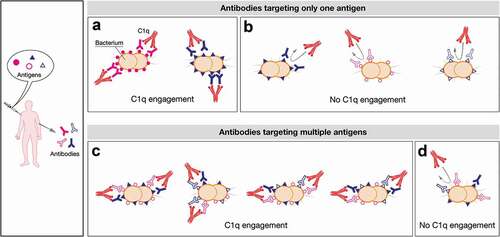
It is also important to notice that several of the meningococcal minor OMPs, as well as the vaccine components fHbp and NHBA, can interact with different complement factors. Indeed, the complement system is pivotal in the defense against N. meningitidis, as suggested by the elevated susceptibility to meningococcal infections exhibited by individuals with complement deficiencies [Citation94]. Activation and repression of the host complement system requires strictly regulated mechanisms in order to prevent self-damage. The complement physiological function is guaranteed by the simultaneous presence in vivo of several regulators, like factor H (fH), factor H-like protein-1 (FHL-1) and C4-binding protein (C4BP). N. meningitidis has evolved several strategies for immune-evasion, which involve the recruitment of complement regulators [Citation95,Citation96]. Bacterial proteins like fHbp, NspA, PorB and indirectly NHBA (possibly through binding to heparin) are able to engage the negative complement regulator factor H [Citation97–100]. Likewise, the C4BP protein and the vitronectin are targeted by bacterial PorA and OpcA, respectively [Citation96,Citation101]. Scavenging host complement inhibitors by meningococcal proteins constitutes an important mechanism of subverting complement attack. This leads to a decreased susceptibility to complement-mediated lysis and to a bacterial serum resistance. Therefore, antibodies targeting bacterial proteins involved in complement-binding can have a dual function: to induce bactericidal activity and to displace fH from the bacterial surface subverting the mechanism of complement evasion, thereby compromising bacterial survival in vivo. Indeed, antibodies against PorB and NspA may inhibit fH binding and increase strain susceptibility to complement mediated killing. Overall, these results show that a meningococcal vaccine able to simultaneously target multiple antigens is more likely to confer broader protection [Citation102].
8. Conclusion
The scientific highlights reported in this review support the existence of a cooperative and synergistic effect induced by antibodies targeting the different antigens in the 4CMenB vaccine. The high plasticity of the MenB genome which leads to high rates of antigenic and phase variation, and the different degree of antigen expression pose severe challenges for a meningococcal vaccine with broad strain coverage. Hence, a multicomponent vaccine like 4CMenB, which includes an array of different antigens, is more likely to provide broader protective immunity. It has been shown that antibodies induced by fHbp, NHBA, and minor OMV components can bind simultaneously the bacterial surface overcoming the limitations of a low surface expression and/or a high antigenic diversity and triggering complement mediated bacterial lysis. MenB strain coverage prediction tools underestimate overall vaccine potential, since they only measure one antigen at time. This review shows how different antibodies can play a key role in bacterial killing in a concerted manner. The inclusion of more than one antigen in a vaccine may offer added and underappreciated value due to the protective effect mediated by the cooperative/synergistic activity of antibodies.
9. Expert opinion
Mechanism of antibody-mediated protection by multicomponent vaccines cannot be always ascribed to the contribution of each single component but can rather be due to a complex interplay of antibodies acting in synergy in fighting the infection. The molecular deconvolution of the immune response of complex vaccines, such in the case of 4CMenB, is challenging, but is becoming possible thanks to the significant amount of strain coverage data and real-world evidence supporting also the potential beneficial secondary effects [Citation103]. Vaccine coverage predictor tools based on ELISA or genetics, measure the independent contribution of each individual antigen and not of their combination, resulting in an underestimation of real vaccine coverage. Therefore, the use of functional assays, like SBA, on a representative panel of disease-causing isolates may be the unique solution to measure synergies among antibodies elicited by each vaccine component. This is particularly important in case of vaccines containing complex antigens, like in the case of OMV, in which only PorA is considered as playing a role in protection. It is conceivable that other antigens in OMV induce antibodies that may contribute to protection and cross-protection, and to the immunostimulatory activities. As a consequence, a complete dissection of the OMV determinants able to contribute to anti-pathogen immunity is fundamental for an in-depth understanding of the mechanisms of protection and for tailoring novel methods to predict coverage. Although several studies have investigated the phenomenon of antibody cooperativity in complement-mediated bactericidal activity, the specific molecular mechanisms by which couples of antibodies non-bactericidal on their own become functional only when combined still remains unclear. A complete deconvolution of the immune response induced by complex multicomponent vaccine formulation may be possible in future thanks to the advances in resolution of high complex structures together with sophisticated protein modeling studies. These analyses may provide key insights on the interaction and structure of antigen–antibody complexes, on the specific epitopes engaged in the simultaneous binding of the antibodies and of the signal activated by these bindings. Identification of epitopes and antigens contributing synergistically may allow a complete understanding of the immune response induced by multicomponent vaccines and may open the way to new coverage prediction tools that better reflect real vaccine coverage and guide the design of more effective multicomponent vaccines.
Article highlights
Multicomponent vaccines may induce antibodies acting cooperatively and synergistically in tackling the infection.
Monoclonal and polyclonal antibodies raised by vaccination with 4CMenB show cooperative and synergistic activity in complement-mediated bacterial killing.
Antibodies induced by fHbp, NHBA, and minor OMV components can act in concert and be functional against meningococcal strains not predicted to be covered by classical MenB prediction tools.
The simultaneous binding of antibodies to various surface-exposed antigens can overcome the threshold density of antigen–antibody complexes needed for complement activation.
A full understanding of the synergistic mechanisms may allow the definition of new coverage prediction tools and guide the design of multicomponent vaccines.
Trademark
Bexsero is a trademark owned by or licensed to the GSK group of companies. Trumenba is a trademark owned by Pfizer. VA-MENGOC-BC is a trademark of the Finlay Institute, Cuba. MenBvac is a trademark of the Norwegian Institute of Public Health. MeNZB is a trademark of Novartis.
Human samples
The human infant samples used in the study were obtained via a Phase II clinical trial (V72P16, NCT00937521) conducted in multiple centres after Ethical committee approval in the Czech Republic, Italy, Hungary, Chile, and Argentina between July 2009 and November 2010 in accordance with Good Clinical Practices and according to the Declaration of Helsinki. An informed consent form, which included permission for potential re-use of the biological samples, was obtained from parents or guardian of the participant in the clinical trial. Serum samples used in this analysis are pool of antiserum from 25 subjects assuring the anonymity of any subject so that no further ethical committee approval was needed. Human complement source used in the study was obtained according to Good Clinical Practice in accordance with the declaration of Helsinki. Patients have given their written consent for the use of samples of study MENB REC 2ND GEN-074 (V72_92). The study was approved by the Western Institutional Review Board (WIRB).
Declaration of interest
V Viviani was a PhD student at the University of Bologna and participated in a postgraduate studentship program at GSK at the time of the study and she is now an employee of the GSK group of companies. A Biolchi and M Pizza are employees of the GSK group of companies. The authors have no other relevant affiliations or financial involvement with any organization or entity with a financial interest in or financial conflict with the subject matter or materials discussed in the manuscript apart from those disclosed.
Reviewer disclosures
Peer reviewers on this manuscript have no relevant financial or other relationships to disclose.
Author contributions
VV, AB, and MP have substantially contributed to the conception and design of the review article and to the interpretation of the relevant literature and they have all been involved in writing the review article.
Acknowledgments
The authors would like to thank Sarah Nosari for the contribution to the study and Giorgio Corsi for kind assistance in preparation of the artwork.
Additional information
Funding
References
- Acevedo R, Bai X, Borrow R, et al. The Global Meningococcal Initiative meeting on prevention of meningococcal disease worldwide: epidemiology, surveillance, hypervirulent strains, antibiotic resistance and high-risk populations. Expert Rev Vaccines. 2019;18(1):15–30. PubMed PMID: 30526162.
- Pelton SI. The global evolution of meningococcal epidemiology following the introduction of meningococcal vaccines. J Adolesc Health. 2016;59(2 Suppl):S3–S11. PubMed PMID: 27449148.
- Finne J, Leinonen M, Makela PH. Antigenic similarities between brain components and bacteria causing meningitis. Implications for vaccine development and pathogenesis. Lancet. 1983;2(8346):355–357. PubMed PMID: 6135869.
- Tappero JW, Lagos R, Ballesteros AM, et al. Immunogenicity of 2 serogroup B outer-membrane protein meningococcal vaccines. a randomized controlled trial in Chile. JAMA. 1999;281(16):1520–1527. PubMed PMID: 10227322.
- Fredriksen JH, Rosenqvist E, Wedege E, et al. Production, characterization and control of MenB-vaccine “Folkehelsa”: an outer membrane vesicle vaccine against group B meningococcal disease. NIPH Ann. 1991;14(2):67–79; discussion 79-80. PubMed PMID: 1812438.
- Sierra GV, Campa HC, Varcacel NM, et al. Vaccine against group B Neisseria meningitidis: protection trial and mass vaccination results in Cuba. NIPH Ann. 1991;14(2):195–207; discussion 208-10. PubMed PMID: 1812432.
- Oster P, Lennon D, O’Hallahan J, et al.MeNZB: a safe and highly immunogenic tailor-made vaccine against the New Zealand Neisseria meningitidis serogroup B disease epidemic strain. Vaccine. 2005;23(17–18):2191–2196. PubMed PMID: 15755593
- Sevestre J, Hong E, Delbos V, et al. Durability of immunogenicity and strain coverage of MenBvac, a meningococcal vaccine based on outer membrane vesicles: lessons of the Normandy campaign. Vaccine. 2017;35(32):4029–4033. PubMed PMID: 28624305.
- Milagres LG, Ramos SR, Sacchi CT, et al. Immune response of Brazilian children to a Neisseria meningitidis serogroup B outer membrane protein vaccine: comparison with efficacy. Infect Immun. 1994;62(10):4419–4424. PubMed PMID: 7927704; PubMed Central PMCID: PMCPMC303125.
- Tani C, Stella M, Donnarumma D, et al. Quantification by LC-MS(E) of outer membrane vesicle proteins of the Bexsero(R) vaccine. Vaccine. 2014;32(11):1273–1279. PubMed PMID: 24462403.
- Martin DR, Ruijne N, McCallum L, et al. The VR2 epitope on the PorA P1.7-2,4 protein is the major target for the immune response elicited by the strain-specific group B meningococcal vaccine MeNZB. Clin Vaccine Immunol. 2006;13(4):486–491. PubMed PMID: 16603616; PubMed Central PMCID: PMCPMC1459632.
- Burman C, Alderfer J, Snow VT. A review of the immunogenicity, safety and current recommendations for the meningococcal serogroup B vaccine, MenB-FHbp. J Clin Pharm Ther. 2020;45(2):270–281. PubMed PMID: 31820483.
- Pizza M, Scarlato V, Masignani V, et al.Identification of vaccine candidates against serogroup B meningococcus by whole-genome sequencing. Science. 2000;287(5459):1816–1820. PubMed PMID: 10710308
- Watson PS, Turner DP. Clinical experience with the meningococcal B vaccine, Bexsero((R)): prospects for reducing the burden of meningococcal serogroup B disease. Vaccine. 2016;34(7):875–880. PubMed PMID: 26686570.
- Giuliani MM, Adu-Bobie J, Comanducci M, et al.A universal vaccine for serogroup B meningococcus. Proc Natl Acad Sci USA. 2006;103(29):10834–10839. PubMed PMID: 16825336; PubMed Central PMCID: PMCPMC2047628
- Goldschneider I, Gotschlich EC, Artenstein MS. Human immunity to the meningococcus. I. The role of humoral antibodies. J Exp Med. 1969;129(6):1307–1326. PubMed PMID: 4977280; PubMed Central PMCID: PMCPMC2138650.
- Bash MC, Lynn F, Mocca B, et al. Development and use of a serum bactericidal assay using pooled human complement to assess responses to a meningococcal group A conjugate vaccine in African toddlers. Clin Vaccine Immunol. 2014;21(5):755–761. PubMed PMID: 24671551; PubMed Central PMCID: PMCPMC4018891.
- Giuliani MM, Biolchi A, Serruto D, et al.Measuring antigen-specific bactericidal responses to a multicomponent vaccine against serogroup B meningococcus. Vaccine. 2010;28(31):5023–5030. PubMed PMID: 20493284
- Donnelly J, Medini D, Boccadifuoco G, et al.Qualitative and quantitative assessment of meningococcal antigens to evaluate the potential strain coverage of protein-based vaccines. Proc Natl Acad Sci USA. 2010;107(45):19490–19495. PubMed PMID: 20962280; PubMed Central PMCID: PMCPMC2984153
- Medini D, Stella M, Wassil J. MATS: global coverage estimates for 4CMenB, a novel multicomponent meningococcal B vaccine. Vaccine. 2015;33(23):2629–2636. PubMed PMID: 25882169.
- Frosi G, Biolchi A, Lo Sapio M, et al.Bactericidal antibody against a representative epidemiological meningococcal serogroup B panel confirms that MATS underestimates 4CMenB vaccine strain coverage. Vaccine. 2013;31(43):4968–4974. PubMed PMID: 23954380
- Stella M, Giuliani M, Biolchi A, et al. Does vaccination with 4CMenB convey protection against meningococcal serogroup B strains not predicted to be covered by MATS? A study of the UK clonal complex cc269. Hum Vaccin Immunother. 2020;16(4):945–948. PubMed PMID: 31770063; PubMed Central PMCID: PMCPMC7227617.
- Abad R, Biolchi A, Moschioni M, et al. A large portion of meningococcal antigen typing system-negative meningococcal strains from Spain is killed by sera from adolescents and infants immunized with 4CMenB. Clin Vaccine Immunol. 2015;22(4):357–360. PubMed PMID: 25630407; PubMed Central PMCID: PMCPMC4375344.
- Brehony C, Rodrigues CMC, Borrow R, et al.Distribution of Bexsero(R) Antigen Sequence Types (BASTs) in invasive meningococcal disease isolates: implications for immunisation. Vaccine. 2016;34(39):4690–4697. PubMed PMID: 27521232; PubMed Central PMCID: PMCPMC5012890
- Muzzi A, Brozzi A, Serino L, et al.Genetic Meningococcal Antigen Typing System (gMATS): a genotyping tool that predicts 4CMenB strain coverage worldwide. Vaccine. 2019;37(7):991–1000. PubMed PMID: 30661831
- Findlow J, Borrow R, Snape MD, et al.Multicenter, open-label, randomized phase II controlled trial of an investigational recombinant Meningococcal serogroup B vaccine with and without outer membrane vesicles, administered in infancy. Clin Infect Dis. 2010;51(10):1127–1137. PubMed PMID: 20954968
- Snape MD, Dawson T, Oster P, et al. Immunogenicity of two investigational serogroup B meningococcal vaccines in the first year of life: a randomized comparative trial. Pediatr Infect Dis J. 2010;29(11):e71–9. PubMed PMID: 20844462.
- Esposito S, Prymula R, Zuccotti GV, et al. A phase 2 randomized controlled trial of a multicomponent meningococcal serogroup B vaccine, 4CMenB, in infants (II). Hum Vaccin Immunother. 2014;10(7):2005–2014. PubMed PMID: 25424810; PubMed Central PMCID: PMCPMC4186018.
- Prymula R, Esposito S, Zuccotti GV, et al. A phase 2 randomized controlled trial of a multicomponent meningococcal serogroup B vaccine (I). Hum Vaccin Immunother. 2014;10(7):1993–2004. PubMed PMID: 25424809; PubMed Central PMCID: PMCPMC4186040.
- Vu DM, Wong TT, Granoff DM. Cooperative serum bactericidal activity between human antibodies to meningococcal factor H binding protein and neisserial heparin binding antigen. Vaccine. 2011;29(10):1968–1973. PubMed PMID: 21241734; PubMed Central PMCID: PMCPMC3043162.
- Partridge E, Lujan E, Giuntini S, et al.The role of anti-NHba antibody in bactericidal activity elicited by the meningococcal serogroup B vaccine, MenB-4C. Vaccine. 2017;35(33):4236–4244. PubMed PMID: 28651840; PubMed Central PMCID: PMCPMC5560085
- Welsch JA, Senders S, Essink B, et al. Breadth of coverage against a panel of 110 invasive disease isolates, immunogenicity and safety for 2 and 3 doses of an investigational MenABCWY vaccine in US adolescents - Results from a randomized, controlled, observer-blind phase II study. Vaccine. 2018;36(35):5309–5317. PubMed PMID: 30061029.
- Biolchi A, De Angelis G, Moschioni M, et al. Multicomponent meningococcal serogroup B vaccination elicits cross-reactive immunity in infants against genetically diverse serogroup C, W and Y invasive disease isolates. Vaccine. 2020;38(47):7542–7550. PubMed PMID: 33036804.
- de Moraes JC, Perkins BA, Camargo MC, et al. Protective efficacy of a serogroup B meningococcal vaccine in Sao Paulo, Brazil. Lancet. 1992;340(8827):1074–1078. PubMed PMID: 1357461.
- Ochoa-Azze RF. Cross-protection induced by VA-MENGOC-BC(R) vaccine. Hum Vaccin Immunother. 2018;14(5):1064–1068. PubMed PMID: 29420119; PubMed Central PMCID: PMCPMC5989903.
- Holst J, Feiring B, Naess LM, et al. The concept of “tailor-made,” protein-based, outer membrane vesicle vaccines against meningococcal disease. Vaccine. 2005;23(17–18):2202–2205. PubMed PMID: 15755595.
- Holst J, Martin D, Arnold R, et al. Properties and clinical performance of vaccines containing outer membrane vesicles from Neisseria meningitidis. Vaccine. 2009;27 Suppl 2:B3–12. PubMed PMID: 19481313.
- Holst J, Oster P, Arnold R, et al.Vaccines against meningococcal serogroup B disease containing outer membrane vesicles (OMV): lessons from past programs and implications for the future. Hum Vaccin Immunother. 2013;9(6):1241–1253. PubMed PMID: 23857274; PubMed Central PMCID: PMCPMC3901813
- Arnold R, Galloway Y, McNicholas A, et al.Effectiveness of a vaccination programme for an epidemic of meningococcal B in New Zealand. Vaccine. 2011;29(40):7100–7106. PubMed PMID: 21803101
- Wedege E, Bolstad K, Aase A, et al. Functional and specific antibody responses in adult volunteers in New Zealand who were given one of two different meningococcal serogroup B outer membrane vesicle vaccines. Clin Vaccine Immunol. 2007;14(7):830–838. PubMed PMID: 17494638; PubMed Central PMCID: PMCPMC1951067.
- Rosenqvist E, Hoiby EA, Wedege E, et al. Human antibody responses to meningococcal outer membrane antigens after three doses of the Norwegian group B meningococcal vaccine. Infect Immun. 1995;63(12):4642–4652. PubMed PMID: 7591118; PubMed Central PMCID: PMCPMC173667.
- Wedege E, Hoiby EA, Rosenqvist E, et al. Immune responses against major outer membrane antigens of Neisseria meningitidis in vaccinees and controls who contracted meningococcal disease during the Norwegian serogroup B protection trial. Infect Immun. 1998;66(7):3223–3231. PubMed PMID: 9632589; PubMed Central PMCID: PMCPMC108336.
- Williams JN, Weynants V, Poolman JT, et al.Immuno-proteomic analysis of human immune responses to experimental Neisseria meningitidis outer membrane vesicle vaccines identifies potential cross-reactive antigens. Vaccine. 2014;32(11):1280–1286. PubMed PMID: 24486354
- Mendum TA, Newcombe J, McNeilly CL, et al. Towards the immunoproteome of Neisseria meningitidis. PLoS One. 2009;4(6):e5940. PubMed PMID: 19529772; PubMed Central PMCID: PMCPMC2691954.
- Williams JN, Skipp PJ, O’Connor CD, et al. Immunoproteomic analysis of the development of natural immunity in subjects colonized by Neisseria meningitidis reveals potential vaccine candidates. Infect Immun. 2009;77(11):5080–5089. PubMed PMID: 19737898; PubMed Central PMCID: PMCPMC2772525.
- Awanye AM, Chang CM, Wheeler JX, et al.Immunogenicity profiling of protein antigens from capsular group B Neisseria meningitidis. Sci Rep. 2019;9(1):6843. PubMed PMID: 31048732; PubMed Central PMCID: PMCPMC6497663
- Wright JC, Williams JN, Christodoulides M, et al. Immunization with the recombinant PorB outer membrane protein induces a bactericidal immune response against Neisseria meningitidis. Infect Immun. 2002;70(8):4028–4034. PubMed PMID: 12117908; PubMed Central PMCID: PMCPMC128133.
- Ala’Aldeen DA, Borriello SP. The meningococcal transferrin-binding proteins 1 and 2 are both surface exposed and generate bactericidal antibodies capable of killing homologous and heterologous strains. Vaccine. 1996;14(1):49–53. PubMed PMID: 8821649.
- Gorringe AR, Borrow R, Fox AJ, et al. Human antibody response to meningococcal transferrin binding proteins: evidence for vaccine potential. Vaccine. 1995;13(13):1207–1212. PubMed PMID: 8578805.
- Danve B, Lissolo L, Mignon M, et al. Transferrin-binding proteins isolated from Neisseria meningitidis elicit protective and bactericidal antibodies in laboratory animals. Vaccine. 1993;11(12):1214–1220. PubMed PMID: 8256502.
- West D, Reddin K, Matheson M, et al. Recombinant Neisseria meningitidis transferrin binding protein A protects against experimental meningococcal infection. Infect Immun. 2001;69(3):1561–1567. PubMed PMID: 11179327; PubMed Central PMCID: PMCPMC98056.
- Rokbi B, Mignon M, Maitre-Wilmotte G, et al. Evaluation of recombinant transferrin-binding protein B variants from Neisseria meningitidis for their ability to induce cross-reactive and bactericidal antibodies against a genetically diverse collection of serogroup B strains. Infect Immun. 1997;65(1):55–63. PubMed PMID: 8975892; PubMed Central PMCID: PMCPMC174556.
- Ala’Aldeen DA, Stevenson P, Griffiths E, et al. Immune responses in humans and animals to meningococcal transferrin-binding proteins: implications for vaccine design. Infect Immun. 1994;62(7):2984–2990. PubMed PMID: 8005685; PubMed Central PMCID: PMCPMC302908.
- Pettersson A, Kortekaas J, Weynants VE, et al. Vaccine potential of the Neisseria meningitidis lactoferrin-binding proteins LbpA and LbpB. Vaccine. 2006;24(17):3545–3557. PubMed PMID: 16517031.
- Pettersson A, Prinz T, Umar A, et al. Molecular characterization of LbpB, the second lactoferrin-binding protein of Neisseria meningitidis. Mol Microbiol. 1998;27(3):599–610. PubMed PMID: 9489671.
- Black JR, Dyer DW, Thompson MK, et al. Human immune response to iron-repressible outer membrane proteins of Neisseria meningitidis. Infect Immun. 1986;54(3):710–713. PubMed PMID: 3096888; PubMed Central PMCID: PMCPMC260227.
- Sanders H, Norheim G, Chan H, et al. FetA antibodies induced by an outer membrane vesicle vaccine derived from a serogroup B meningococcal isolate with constitutive FetA expression. PLoS One. 2015;10(10):e0140345. PubMed PMID: 26466091; PubMed Central PMCID: PMCPMC4605655.
- Marsay L, Dold C, Green CA, et al. A novel meningococcal outer membrane vesicle vaccine with constitutive expression of FetA: a phase I clinical trial. J Infect. 2015;71(3):326–337. PubMed PMID: 25982025; PubMed Central PMCID: PMCPMC4535279.
- Rosenqvist E, Hoiby EA, Wedege E, et al. The 5C protein of Neisseria meningitidis is highly immunogenic in humans and induces bactericidal antibodies. J Infect Dis. 1993;167(5):1065–1073. PubMed PMID: 8486938.
- Jolley KA, Appleby L, Wright JC, et al. Immunization with recombinant Opc outer membrane protein from Neisseria meningitidis: influence of sequence variation and levels of expression on the bactericidal immune response against meningococci. Infect Immun. 2001;69(6):3809–3816. PubMed PMID: 11349046; PubMed Central PMCID: PMCPMC98398.
- Musacchio A, Carmenate T, Delgado M, et al. Recombinant Opc meningococcal protein, folded in vitro, elicits bactericidal antibodies after immunization. Vaccine. 1997;15(6–7):751–758. PubMed PMID: 9178478.
- Carmenate T, Mesa C, Menendez T, et al. Recombinant Opc protein from Neisseria meningitidis reconstituted into liposomes elicits opsonic antibodies following immunization. Biotechnol Appl Biochem. 2001;34(1):63–69. PubMed PMID: 11483156.
- Perez RE, Lasa AM, Rodriguez RS, et al. Scale-up of recombinant Opc protein production in Escherichia coli for a meningococcal vaccine. J Biotechnol. 2006;127(1):109–114. PubMed PMID: 16893582.
- Hung MC, Heckels JE, Christodoulides M. The adhesin complex protein (ACP) of Neisseria meningitidis is a new adhesin with vaccine potential. mBio. 2013;4(2): PubMed PMID: 23443003; PubMed Central PMCID: PMCPMC3585444. DOI:https://doi.org/10.1128/mBio.00041-13.
- Hung MC, Heckels JE, Christodoulides M. Potential of the adhesin complex protein of Neisseria meningitidis for next-generation meningococcal vaccines. Expert Rev Vaccines. 2013;12(9):981–984. PubMed PMID: 24053391.
- Kolappan S, Coureuil M, Yu X, et al. Structure of the Neisseria meningitidis Type IV pilus. Nat Commun. 2016;7:13015. PubMed PMID: 27698424; PubMed Central PMCID: PMCPMC5059446.
- Haghi F, Peerayeh SN, Siadat SD, et al. Recombinant outer membrane secretin PilQ(406-770) as a vaccine candidate for serogroup B Neisseria meningitidis. Vaccine. 2012;30(9):1710–1714. PubMed PMID: 22234267.
- Maharjan S, Saleem M, Feavers IM, et al. Dissection of the function of the RmpM periplasmic protein from Neisseria meningitidis. Microbiology. 2016;162(2):364–375. PubMed PMID: 26678687.
- Rosenqvist E, Musacchio A, Aase A, et al. Functional activities and epitope specificity of human and murine antibodies against the class 4 outer membrane protein (Rmp) of Neisseria meningitidis. Infect Immun. 1999;67(3):1267–1276. PubMed PMID: 10024570; PubMed Central PMCID: PMCPMC96456.
- Weynants VE, Feron CM, Goraj KK, et al. Additive and synergistic bactericidal activity of antibodies directed against minor outer membrane proteins of Neisseria meningitidis. Infect Immun. 2007;75(11):5434–5442.
- Wedege E, Lie K, Bolstad K, et al. Meningococcal omp85 in detergent-extracted outer membrane vesicle vaccines induces high levels of non-functional antibodies in mice. Scand J Immunol. 2013;77(6):452–459. PubMed PMID: 23521186.
- Cadieux N, Plante M, Rioux CR, et al. Bactericidal and cross-protective activities of a monoclonal antibody directed against Neisseria meningitidis NspA outer membrane protein. Infect Immun. 1999;67(9):4955–4959. PubMed PMID: 10456958; PubMed Central PMCID: PMCPMC96836.
- Moe GR, Tan S, Granoff DM. Differences in surface expression of NspA among Neisseria meningitidis group B strains. Infect Immun. 1999;67(11):5664–5675. PubMed PMID: 10531214; PubMed Central PMCID: PMCPMC96940.
- Ying S, He J, Yu M, et al. Recombinant Neisseria surface protein A is a potential vaccine candidate against Neisseria meningitides serogroup B. Mol Med Rep. 2014;10(3):1619–1625. PubMed PMID: 24926810.
- Martin D, Brodeur BR, Hamel J, et al. Candidate Neisseria meningitidis NspA vaccine. J Biotechnol. 2000;83(1–2):27–31. PubMed PMID: 11000456.
- Martin D, Cadieux N, Hamel J, et al. Highly conserved Neisseria meningitidis surface protein confers protection against experimental infection. J Exp Med. 1997;185(7):1173–1183. PubMed PMID: 9104804; PubMed Central PMCID: PMCPMC2196255.
- Halperin SA, Langley JM, Smith B, et al. Phase 1 first-in-human studies of the reactogenicity and immunogenicity of a recombinant meningococcal NspA vaccine in healthy adults. Vaccine. 2007;25(3):450–457. PubMed PMID: 17052819.
- Hung MC, Salim O, Williams JN, et al. The Neisseria meningitidis macrophage infectivity potentiator protein induces cross-strain serum bactericidal activity and is a potential serogroup B vaccine candidate. Infect Immun. 2011;79(9):3784–3791. PubMed PMID: 21708989; PubMed Central PMCID: PMCPMC3165472.
- Bielecka MK, Devos N, Gilbert M, et al. Recombinant protein truncation strategy for inducing bactericidal antibodies to the macrophage infectivity potentiator protein of Neisseria meningitidis and circumventing potential cross-reactivity with human FK506-binding proteins. Infect Immun. 2015;83(2):730–742. PubMed PMID: 25452551; PubMed Central PMCID: PMCPMC4294229.
- Costoya L, Marzoa J, Ferreiros C, et al. Liposomes or traditional adjuvants: induction of bactericidal activity by the macrophage infectivity potentiator protein (Mip) of Neisseria meningitidis. APMIS. 2017;125(8):725–731. PubMed PMID: 28543600.
- Sardinas G, Yero D, Climent Y, et al. Neisseria meningitidis antigen NMB0088: sequence variability, protein topology and vaccine potential. J Med Microbiol. 2009;58(Pt 2):196–208. PubMed PMID: 19141737.
- Keiser PB, Gibbs BT, Coster TS, et al. A phase 1 study of a group B meningococcal native outer membrane vesicle vaccine made from a strain with deleted lpxL2 and synX and stable expression of opcA. Vaccine. 2010;28(43):6970–6976. PubMed PMID: 20732470.
- Keiser PB, Biggs-Cicatelli S, Moran EE, et al. A phase 1 study of a meningococcal native outer membrane vesicle vaccine made from a group B strain with deleted lpxL1 and synX, over-expressed factor H binding protein, two PorAs and stabilized OpcA expression. Vaccine. 2011;29(7):1413–1420. PubMed PMID: 21199704.
- Petousis-Harris H, Paynter J, Morgan J, et al.Effectiveness of a group B outer membrane vesicle meningococcal vaccine against gonorrhoea in New Zealand: a retrospective case-control study. Lancet. 2017;390(10102):1603–1610. PubMed PMID: 28705462
- Semchenko EA, Tan A, Borrow R, et al. The serogroup B meningococcal vaccine bexsero elicits antibodies to Neisseria gonorrhoeae. Clin Infect Dis. 2019;69(7):1101–1111. PubMed PMID: 30551148; PubMed Central PMCID: PMCPMC6743822.
- Beernink PT, Welsch JA, Bar-Lev M, et al. Fine antigenic specificity and cooperative bactericidal activity of monoclonal antibodies directed at the meningococcal vaccine candidate factor h-binding protein. Infect Immun. 2008;76(9):4232–4240. PubMed PMID: 18591239; PubMed Central PMCID: PMCPMC2519416.
- Faleri A, Santini L, Brier S, et al. Two cross-reactive monoclonal antibodies recognize overlapping epitopes on Neisseria meningitidis factor H binding protein but have different functional properties. FASEB J. 2014;28(4):1644–1653. PubMed PMID: 24371123.
- Malito E, Lo Surdo P, Veggi D, et al. Neisseria meningitidis factor H-binding protein bound to monoclonal antibody JAR5: implications for antibody synergy. Biochem J. 2016;473(24):4699–4713. PubMed PMID: 27784765; PubMed Central PMCID: PMCPMC6398935.
- Biagini M, Spinsanti M, De Angelis G, et al. Expression of factor H binding protein in meningococcal strains can vary at least 15-fold and is genetically determined. Proc Natl Acad Sci USA. 2016;113(10):2714–2719. PubMed PMID: 26888286; PubMed Central PMCID: PMCPMC4791009.
- Diebolder CA, Beurskens FJ, de Jong RN, et al. Complement is activated by IgG hexamers assembled at the cell surface. Science. 2014;343(6176):1260–1263. PubMed PMID: 24626930; PubMed Central PMCID: PMCPMC4250092.
- Giuliani M, Bartolini E, Galli B, et al. Human protective response induced by meningococcus B vaccine is mediated by the synergy of multiple bactericidal epitopes. Sci Rep. 2018;8(1):3700. PubMed PMID: 29487324; PubMed Central PMCID: PMCPMC5829249.
- Peschiera I, Giuliani M, Giusti F, et al. Structural basis for cooperativity of human monoclonal antibodies to meningococcal factor H-binding protein. Commun Biol. 2019;2:241. PubMed PMID: 31263785; PubMed Central PMCID: PMCPMC6595007.
- Natali EN, Principato S, Ferlicca F, et al. Synergic complement-mediated bactericidal activity of monoclonal antibodies with distinct specificity. FASEB J. 2020;34:10329–10341. PubMed PMID: 32725956.
- Figueroa J, Andreoni J, Densen P. Complement deficiency states and meningococcal disease. Immunol Res. 1993;12(3):295–311. PubMed PMID: 8288947.
- Blom AM, Hallstrom T, Riesbeck K. Complement evasion strategies of pathogens-acquisition of inhibitors and beyond. Mol Immunol. 2009;46(14):2808–2817. PubMed PMID: 19477524.
- Lewis LA, Ram S. Complement interactions with the pathogenic Neisseriae: clinical features, deficiency states, and evasion mechanisms. FEBS Lett. 2020;594:2670–2694. PubMed PMID: 32058583.
- Lewis LA, Ngampasutadol J, Wallace R, et al. The meningococcal vaccine candidate neisserial surface protein A (NspA) binds to factor H and enhances meningococcal resistance to complement. PLoS Pathog. 2010;6(7):e1001027. PubMed PMID: 20686663; PubMed Central PMCID: PMCPMC2912398.
- Lewis LA, Vu DM, Vasudhev S, et al. Factor H-dependent alternative pathway inhibition mediated by porin B contributes to virulence of Neisseria meningitidis. mBio. 2013;4(5):e00339–13. PubMed PMID: 24129254; PubMed Central PMCID: PMCPMC3812710.
- Madico G, Welsch JA, Lewis LA, et al. The meningococcal vaccine candidate GNA1870 binds the complement regulatory protein factor H and enhances serum resistance. J Immunol. 2006;177(1):501–510. PubMed PMID: 16785547; PubMed Central PMCID: PMCPMC2248442.
- Serruto D, Spadafina T, Ciucchi L, et al. Neisseria meningitidis GNA2132, a heparin-binding protein that induces protective immunity in humans. Proc Natl Acad Sci USA. 2010;107(8):3770–3775. PubMed PMID: 20133713; PubMed Central PMCID: PMCPMC2840514.
- Jarva H, Ram S, Vogel U, et al. Binding of the complement inhibitor C4bp to serogroup B Neisseria meningitidis. J Immunol. 2005;174(10):6299–6307. PubMed PMID: 15879129.
- Giuntini S, Pajon R, Ram S, et al. Binding of complement factor H to PorB3 and NspA enhances resistance of Neisseria meningitidis to anti-factor H binding protein bactericidal activity. Infect Immun. 2015;83(4):1536–1545. PubMed PMID: 25644002; PubMed Central PMCID: PMCPMC4363443.
- Thompson KM, Badizadegan K. Health economic analyses of secondary vaccine effects: a systematic review and policy insights. Expert Rev Vaccines. 2022; 1–16. PubMed PMID: 34927511. DOI: https://doi.org/10.1080/14760584.2022.2017287