ABSTRACT
Introduction
Developing a safe and efficacious vaccine that can induce broad and long-term immunity for SARS-CoV-2 infection is the most critical research to date. As the most potent APCs, dendritic cells (DCs) can induce a robust T cell immunity. In addition, DCs also play an essential role in COVID-19 pathogenesis, making them a potential vaccination target. However, the DCs-based vaccine with ex vivo loading has not yet been explored for COVID-19.
Areas covered
This review aims to provide the rationale for developing a DCs-based vaccine with ex vivo loading of SARS-CoV-2 antigen. Here, we discuss the role of DCs in immunity and the effect of SARS-CoV-2 infection on DCs. Then, we propose the mechanism of the DCs-based vaccine in inducing immunity and highlight the benefits of ex vivo loading of antigen.
Expert opinion
We make the case that an ex vivo loaded DC-based vaccination is appropriate for COVID-19 prevention.
1. Introduction
COVID-19 (coronavirus infection disease-2019) is a mild to severe respiratory disease caused by SARS-CoV-2 (Severe Acute Respiratory Syndrome Coronavirus-2) infection [Citation1]. This disease is a primary health concern worldwide due to the pandemic. Although several vaccines have already been approved for emergency use, herd immunity generally relies on vaccines [Citation2]. However, recent findings suggest that Variant of Concerns (VoC) might escape vaccine-induced immunity hindering its efficacy [Citation3]. In addition, immunity waning raises the concern for the long-term efficacy of currently available vaccines [Citation4,Citation5]. Thus, discovering safe and efficacious vaccines that lead to broader and long-term immunity remains challenging.
The DCs-based vaccine is one of the promising vaccine candidates due to the vital role DCs play in the immunity and pathogenesis of COVID-19 [Citation6]. Ex vivo loading of antigens to DCs is mainly developed for cancer therapy and chronic viral infection. Dendritic cell-based vaccine is a novel vaccination approach. Studies of dendritic cell vaccines in cancer patients that have been conducted to date show promising results [Citation7–12]. For example, adding the autologous tumor lysate-pulsed dendritic cell vaccine to standard therapy for glioblastoma patients may prolong survival [Citation9]. Other studies conducted on Wilms tumor patients using autologous dendritic cells incubated with WT1 peptides showed induction of systemic tumor antigen-specific immune response, increased infiltration of CD8+ tumor T cells, and increased tumor PD-L1 expression [Citation8]. This method was also developed for HIV-1 and Hepatitis B [Citation13,Citation14]. Furthermore, DCs-based vaccines were also developed for influenza vaccination in high-risk patients who respond poorly to traditional vaccines, such as immunocompromised and cancer patients [Citation15]. In light of this, this method might be used for other infections such as SARS-CoV-2. However, its potential as a COVID-19 vaccine has not been explored.
This review aims to provide the rationale for developing a DC-based vaccine with ex vivo loading of SARS-CoV-2 antigen. First, we discuss the role of dendritic cells in immunity against infection. Then, we review the effects of SARS-CoV-2 infection on dendritic cells. From here, we propose the mechanism of the DC-based vaccine with ex vivo loading of antigen in inducing immunity. Finally, we also highlight the benefits of ex vivo loading of antigen.
2. Dendritic cells in immunity
2.1. Dendritic cell in innate immunity
The human body has a defense system that works during pathogen attacks. There are two types of immune response: innate and adaptive immune responses [Citation16]. The innate immune response is the first line of defense that is activated instantly within minutes to hours after the pathogen invasion. However, innate immune responses are generally nonspecific and unadaptable. Meanwhile, the adaptive immune response can recognize antigens specifically through diversified somatic receptors on B cells and T cells. So that, although requiring a longer time to be acquired, the adaptive immune response will cause a much faster and more effective response upon antigen re-encounter [Citation17].
The innate immune response consists of various modalities: physical, chemical, and cellular defenses. Physical defense protects the body from pathogens using epithelial tissue and mucous membrane [Citation18]. When a pathogen successfully penetrates this defense, the cellular component of innate immunity reacts by destroying the pathogen through phagocytosis. The primary phagocytic cells are derived from myeloid progenitor cells, such as monocyte-macrophage cells, neutrophils, basophils, and eosinophils. Meanwhile, Natural Killer (NK) cells are nonspecific cytotoxic, which destroy cells infected with viruses or intracellular pathogens [Citation19]. Finally, Dendritic Cells (DCs) are also an essential cellular component in the innate immune response, which have phagocytic abilities, produce inflammatory mediators, and present antigen [Citation20] ().
Figure 1. DCs role in innate and adaptive immune response. Pathogens that pass through the physical barrier (epithelium and mucosa) activate the innate immune response and adaptive immune response. First, monocytes-macrophages, neutrophils, and DC capture pathogens, then express antigens on cell membranes. next, DCs as the primary APC migrate to the lymph nodes, where antigens are introduced to T and B cells, thus triggering memory development.
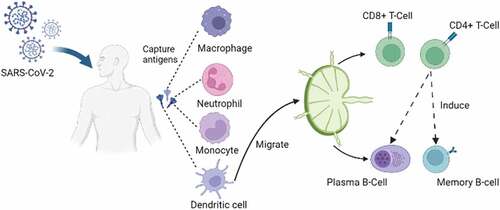
The effectiveness of the innate immune response in fighting viral infections depends on the production of IFN-1. IFN-1 suppresses the spread of the virus through the intrinsic antimicrobial activity of infected cells, promotes antigen presentation, and induces NK cells activation [Citation21]. Most IFN-1 is produced by plasmacytoid dendritic cells (pDC) [Citation22]. pDC recognizes the virus through Toll-Like Receptors (TLR) 7 and 9 [Citation23]. Stimulation of these receptors triggers massive IFN-1 release within 1–3 hours after viral infection, especially the IFN-α and IFN-β subtypes [Citation24].
In addition to IFN-1, cellular components of innate immunity express other types of inflammatory mediators that are important for cells communication. For example, NK cells produce IFN-γ that modulates DCs function [Citation25]. IFN-γ triggers DC maturation, increases MHC I and II expression, triggers antigen presentation, and boosts Interleukin 2 (IL-2) and Cluster of Differentiation 80 (CD80) expression, which are vital in signaling T cell and B cell activation [Citation26,Citation27]. Furthermore, communication between NK and DC is bidirectional, leading to the amplification of pro-inflammatory cytokines [Citation28].
M1 (inflammatory types) macrophages produce a variety of inflammatory cytokines, including TNF-α [Citation29]. TNF-α produced by these macrophages mediates DCs maturation and NK cells activation [Citation30]. In addition, TNF-α plays a role in immunoregulation by limiting the expression of CXR2 receptors and the TLR7/9-induced gene in pDC [Citation31]. In sepsis, TNF-α decreases autophagy function and causes DC dysfunction [Citation32]. Taken together, similar to NK cells, macrophages also modulate DCs function via TNF-α. Crosstalks between these cells create a balanced cytokine milieu in the innate immune response.
2.2. Dendritic cell in adaptive immunity
When the innate immune response cannot destroy antigens, the body activates the adaptive immune response [Citation33] (). Adaptive immunity is antigen-dependent and specific. It can induce immunological memory that causes a faster reaction to antigen re-exposure. It is important to note that recent studies have found that immunological memory can also occur in innate immunity to a certain extent [Citation34]. However, it remains a fundamental feature of adaptive immunity.
Adaptive immunity consists of T-cell mediated immunity, and B-cell mediated immunity [Citation35]. B cells and T cells only eliminate antigens recognized by their receptors [Citation36]. So, the key to adaptive immunity is antigen recognition and presentation by Antigen Presenting Cells (APCs). APCs stimulate the expression of specific receptors on T cells and B cells. Compared to other APCs, DCs are more potent in inducing T cells [Citation37,Citation38]. Therefore, DCs are the most essential APCs that bridge innate and adaptive immune responses.
There are five types of DC subsets, namely Plasmacytoid DCs (pDCs), Conventional DCs 1 (cDC1), Conventional DCs 2 (cDC2), Inflammatory DCs, and Langerhans cells [Citation37]. pDC, cDC1, and cDC2 are present constantly in the body [Citation39]. While Inflammatory DCs are derived from monocyte precursors that differentiate into DCs in the event of infection, tissue damage, or the production of cytokines and chemokines [Citation37]. Inflammatory DCs are also called Monocyte Derived Dendritic Cells (MoDCs). Based on the path to access lymphoid organs, the cDCs consist of two types [Citation40]. First, Resident DCs, derived from bone marrow precursors within lymphoid organs, do not pass through peripheral tissues. Second, Migratory DC formed from precursors in peripheral tissues, after exposure to antigens, will transfer to lymphoid organs to further interact with T cells to induce adaptive immunity.
Although it has different classifications, phenotypes, and role specificities, the primary role of DCs is antigen presentation. Even pDC, whose primary function is to produce IFN-1, can be diversified into pDC with phenotypes that are able to stimulate T cells [Citation41]. The DCs will capture pathogenic particles during an infection, then undergo maturation and migrate to the local lymph nodes to activate T cells and B cells [Citation42]. DCs also serves as surveillance cells that continuously take up antigen samples from the environment. Exogenous antigen uptake is done through endocytosis, phagocytosis, micropinocytosis, and receptor-mediated endocytosis [Citation43]. In addition, TLRs on DCs can recognize parts of pathogens. For example, TLR3 can recognize the double-stranded ribonucleic acid (dsRNA), TLR7/8 can recognize single-stranded ribonucleic acid (ssRNA), and TLR9 can recognize 5’ – C – phosphate – G – 3’ deoxyribonucleic acid (CpG DNA). The interaction between pathogen-associated molecular patterns (PAMPS) or danger-associated molecular patterns (DAMPS) and TLR causes activation of various transcription factors resulting in DCs maturation [Citation44].
At the time of maturation, DCs undergo morphological and phenotype changes. Immature DCs have a rounded shape and high phagocytosis ability but express fewer co-stimulatory molecules. After interaction with pathogens, DCs transform their morphology to cells with longer dendrites and pseudopodia and express more co-stimulatory molecules such as CD80 and CD86. In addition, mature DCs have weaker phagocytosis ability but much higher migratory ability than immature DCs [Citation45].
In the acute phase of infection, especially infections caused by intracellular viruses or bacteria, some monocyte cells turn into MoDCs. IFN-γ primarily mediates the formation of MoDCs [Citation46]. Moreover, MoDCs isolated from peripheral blood can differentiate into MoDC outside the body. This manipulation is done by incubating peripheral blood mononuclear cells (PBMCs) in the differentiation media such as GM-CSF and IL-4 [Citation47]. This method is the most widely used DCs-based vaccine manufacturing.
As the primary APC, DCs have unique capabilities compared to others. DCs can present exogenous antigens through both MHC-I and MHC-II (cross-presentation). Cross-presentation can occur through the cytophilic pathway involving the Endoplasmic Reticulum (ER) and the vacuolar pathway in the endocytic compartment [Citation48,Citation49]. Through the cytophilic pathway, pathogenic proteins are translocated to ER. Inside ER, MHC-I binds those proteins to be translocated back (retrotranslocation) to the cytosol [Citation49]. Antigen presentation by DCs also involves extracellular vesicles, thus enabling cross-dressing (peptide-loaded MHC retrieval from dead cells or cells that have undergone apoptosis) [Citation50].
DCs with peptide-loaded MHC (pMHC) migrate to secondary lymphatic organs to activate T cells. The migration of DCs to the secondary lymphatic organs is regulated by chemokines C-C Chemokine Ligand (CCL) 19 and 21 secreted by lymphatic endothelial cells. It also relies on C-C Chemokine Receptor 7 (CCR7) for DCs positioning and initiation of specific immune responses [Citation51,Citation52]. In secondary lymphoid organs, DCs actively spread antigens to other DCs through cross-dressing, consequently increasing the number of effective APCs [Citation53]. So that, T cells priming can still occur even if the donor DCs cannot directly present the antigen.
T cell priming occurs in secondary lymphoid organs. This process requires complicated T cell and DC signaling to ensure contact. After migration to secondary lymphoid organs, DCs tend to settle, while T cells can scan through hundreds of DCs by moving from one lymph node to another. Although DCs are estimated to interact with 5000 T cells per hour, initiation of T cell response remains highly dependent on the possibility of T cells encountered by DCs [Citation52,Citation54]. A study in mice found that it takes at least 85 DCs to initiate T cell priming, so in order to induce a response, this threshold should be met [Citation55]. In addition to that, T cells priming requires three signals: 1) Interaction of T Cell Receptors (TCR) with pMHC; 2) Co-stimulatory signals through the attachment of CD28 with CD80 and CD86; 3) Cytokines expressed by DCs such as IL-12 [Citation52]. Damage to any of these signals will cause failure to T priming.
Antigens presented by MHC-I triggers CD8 + T cell response, while antigens presented by MHC-II triggers CD4 + T cell response. CD8 + T cells primarily kill host cells infected with viruses. Therefore, most CD8+ will proliferate during acute viral infections after exposure to antigens presented by DCs to assist viral clearance [Citation56]. Meanwhile, CD4 + T cells differentiate into various sub-types depending on the cytokines (e.g. Th1, Th2, Tfh, and Th17) [Citation57]. Each sub-types has a specific function in mediating humoral and cellular adaptive immunity. Moreover, after interaction with DCs, some T cells differentiate into T memory cells [Citation52]. DCs are also essential in germinal center (GC) development by facilitating T cell follicular helper (Tfh) differentiation and directly migrating to the light zone of GC [Citation58].
DCs have a vital role in the immune system, as previously described. The role of DCs begins from innate immunity through phagocytic abilities, inflammatory mediators production, and antigen presentation. DCs are professional APCs that connect innate and adaptive immune responses. In the adaptive immune response, DCs have several advantages in carrying out their function as APCs: 1) DCs are much more potential than other APCs; 2) Enable various antigen uptake pathways and can process both endogenic and exogenic pathogens; 3) The migration of DCs to secondary lymphoid organs and recruitment of T cells is a very efficient process so that the probability for the occurrence of T cell priming is very high; 4) Have cross-presentation capabilities to trigger a wide array of T cells response; 5) Lastly, Has cross-dressing capability to allow antigen transfer from other cells and active dissemination of antigens within secondary lymphatic organs.
3. SARS-CoV-2 infection dysregulate dendritic cell
Viruses that enter the body will try to avoid the immune response. Some viruses develop ways to escape immune response by interfering with DCs functions. For example, it has been suggested that DCs dysregulation plays a vital role in SARS-CoV-2 immune evasion [Citation6]. DCs dysregulation causes various abnormalities throughout the innate and adaptive immune response. In this section, we will highlight some of the important findings.
3.1. SARS-CoV-2 causes inadequate innate immune response
SARS-CoV-2 is known to infect cells through the ACE2 receptor and relies on TMPRSS2 [Citation59]. Several other receptors can be the entry point of this virus, including CD147, L-SIGN, and DC-SIGN [Citation60–62]. DCSIGN receptors are widely expressed in DCs and macrophages, while L-SIGN is mainly expressed in liver sinusoid endothelial cells and lymphatic nodes [Citation62]. The presence of such receptors suggests that this virus can directly infect DCs. Research on SARS-CoV infected MoDCs proves that these cells can be infected, but the infection does not cause cell death [Citation63]. In line with these findings, in vitro research proves that infected MoDC does not support viral replication [Citation64].
People infected by SARS-CoV-2 experience an increase in blood serums, pro-inflammatory cytokines, and chemokines such as TNF, IL-6, IL-8, Macrophage Inflammatory Protein-1-α (MIP-1), Monocyte Chemoattractant Protein-1 (MCP-1), and Interferon-inducible Protein-10 (IP-10) [Citation65]. There is a strong correlation between IP-10 and symptom severity [Citation66]. In addition, critical patients have an increased inflammatory response and dysfunctional response related to IFN [Citation67]. Infected DCs are thought to be the source of these excessive pro-inflammatory cytokines. Interestingly, a study shows that macrophages (not DCs) are the source of pro-inflammatory cytokines when infected with SARS-CoV-2 [Citation64,Citation68]. However, infected MoDCs express IP-10, resulting in decreased IFN production and IFN associated genes [Citation64]. Conflicting results on this matter still require further exploration, particularly the immune cells that contribute most to cytokine storms. There are complex interactions between immune cells that have yet been explained. Since specific signaling between immune cells orchestrates innate immunity, it is crucial to identify the checkpoint that causes this dysfunction. Therefore, there is still a possibility that MoDC is related to the increase of pro-inflammatory cytokines.
DCs communicates with NK cells and macrophages in the induced innate immunity [Citation26,Citation27,Citation30–32]. The myeloid compartment of COVID-19 patients is abnormal. Immature and dysfunctional monocytes and neutrophils are associated with the severity of the disease [Citation69]. In studies comparing acute and convalescent-phase COVID-19 patients, low DCs and monocyte count values were obtained in the convalescent phase. Although, the number of T and NK lymphocyte cell counts was increased. This finding indicates the possibility of suppressing the production of monocytes, DC, NK, and T cells, but NK and T cell suppression lasts shorter [Citation70]. As explained in the previous section, the interaction between NK cells, macrophages, and DCs is vital in creating a cytokine induction balance in the innate immune response. Suppression of these cells creates an imbalance in immunoregulation. Disruptions in the axiom of communication between these cells may cause clinical worsening.
There is an IFN-1 response abnormality in COVID-19 patients, as evidenced by the low value of the IFN-1 and IFN-3 [Citation71]. This finding is likely related to pDCs, the primary source of IFN-1. A decrease of pDCs is found in patients infected with SARS-CoV-2 [Citation70]. A timely IFN-1 response is necessary to activate an effective innate immunity. However, in coronaviruses infection, there is a delay in innate immunity for the first ten days in which during this period a steady increase of viral load occurs [Citation72,Citation73]. Ultimately, delayed IFN-1 response also leads to the accumulation of pathogenic macrophages that cause pulmonary infiltration, vascular leakage, and suboptimal T-cell response [Citation74].
Delayed IFN-1 response might be due to SARS-CoV-2 infected pDC. Protein in SARS-CoV-2 such as ORF9b, Nsp14, Nsp10, M, and Nsp1 may inhibit IFN-1 production. At the same time, other proteins such as ORF3a and ORF6 can inhibit the IFN-1 signaling pathway [Citation72]. Furthermore, congenital abnormalities (inborn errors) of the IFN-1 related genes, namely inborn error in TLR-3 and IFN regulatory factor 7 (IRF-7), are found in critical patients [Citation75]. In line with these findings, pDC as the primary source IFN-1 expresses much IRF-7 [Citation24,Citation76]. Furthermore, the presence of autoantibodies against IFN-1 is also found in life-threatening COVID-19 [Citation77]. So that, people with the IFN-1 genetic disorder become more susceptible to clinical worsening [Citation75].
3.2. SARS-CoV-2 causes adaptive immunity disruption
The adaptive immune response to SARS-CoV-2 relies heavily on specific T and B lymphocytes. In COVID-19 patients, there is a tendency for lymphopenia, where the number of CD4 + and CD8 + T cells were low [Citation78]. Decreased number and fatigue of T cells are related to high mortality and severe COVID-19 [Citation79–81]. Low CD4 + T cell counts were also associated with the longer length of ICU treatment [Citation82]. One reason that might explain low T cell count is the direct inhibition from cytokines. For example, increased serum IL-17 is found in critical patients [Citation67]. IL-17, which Th17 produces, is responsible for T cell suppression. In addition, direct T cell inhibition from cytokines such as IL-6, IL-10, or TNF may also contribute [Citation78].
However, another elegant explanation is that the decreased number and lower DCs maturation causes the lack of optimal priming of T cells, resulting in delays of T cells movement. Studies on 32 COVID-19 patients with severe symptoms treated at Heidelberg University Hospital showed low CD8+ and myeloid DCs counts of patients’ blood cultures [Citation67]. The study results to look at the immune system profiles of COVID-19 patients in Prague with peripheral and culture blood isolation methods showed decreased expression of HLA-DR and DCs maturation markers such as CD80 and CD86 in all subsets of DCs [Citation83]. As explained in the previous section, T cell priming would not have occurred without DCs’ co-stimulatory molecules.
Lastly, moDC infected with SARS-CoV-2 may cause T cell death. Research shows increased Tumor Necrosis Factor-related Apoptosis-Inducing Ligand (TRAIL) activity in MoDCs of SARS-CoV patients where TRAIL expression is associated with the amount of SARS-CoV virus [Citation84]. Single-Cell Sequencing of blood mononuclear cells infected with SARS-CoV-2 showed a similar thing: increased expression of TRAIL and its receptors on T cells [Citation85]. TRAIL is a molecule that plays a role in inducing cellular apoptosis pathways. The bond between TRAIL and T cells leads to the Death-Inducing Signaling Complex (DISC) recruitment, resulting in cell death [Citation86]. Thus, infected DCs can directly affect T cell lymphopenia due to apoptosis activity through TRAIL.
Abnormalities in the adaptive immune response can also be caused through other pathways. For example, the SARS-CoV-2 virus can activate the nuclear factor kappa-light-chain enhancer of activated B cells (NFkB). Furthermore, NFkB induces tolerogenic phenotypes, which are immunosuppressive, through the production of IL-10 [Citation87]. Therefore, in a state of viral infection, suppression of NFkB activity is needed to increase the activity of the immune response mediated by IFN [Citation88]. In contrast, there was an increase in the expression of the Programmed Death-Ligand 1 (PD-L1) program, which plays a role in inhibiting the adaptive immune response [Citation83].
Failure to change from innate to the adaptive immune response in SARS-CoV-2 infection led to worsening cases of COVID-19 infection. Disruption of DCs’ ability to accommodate such changes is an integral part of the pathogenesis of COVID-19 (). In addition, DCs has an extensive role in the immune response. Therefore, the COVID-19 vaccination approach that focuses on protecting and improving DCs can result in faster virus clearance and a better prognosis.
Figure 2. Immune Response to SARS-COV-2 Infection. SARS-CoV-2 can infect DC mainly via DC-SIGN receptors. Infected DC become dysfunctional, causing inadequate IFN-1 response, decreased DC maturation, upregulation of pro-inflammatory cytokines, inhibition of adaptive immunity via PD-L1 and NFkB, and T cell apoptosis via TRAIL. These cause the failure of innate and adaptive immunity.
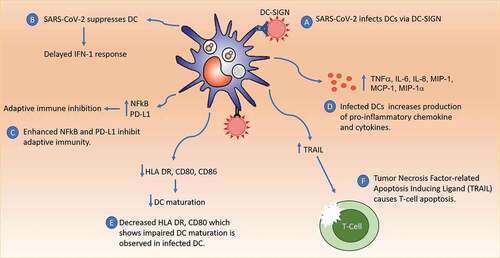
4. Dendritic cell-based vaccine against SARS-CoV-2
There are several reasons to develop DCs-based vaccines for the prevention of COVID-19. First, SARS-CoV-2 causes DCs dysfunction, making DCs an appropriate vaccination target (). In addition, stimulation of DCs results in a robust T cell response [Citation46]. The response of T cells to SARS-CoV-2 lasted longer than the humoral response, which is up to 10 months after infection [Citation89]. Interestingly, research on SARS-CoV showed that memory T cell responses to the virus could survive up to 17 years after infection [Citation90]. DCs-based vaccine might elicit T cell response, which is protective against infection, this includes formation of memory T cells [Citation46]. Unlike neutralizing antibody, which is expected to diminish over time, several findings suggest that T cell responses can be retained for a long period, even years [Citation89,Citation90]. In this case, booster doses might only need to be given after a long time, and might not even be necessary. Hence, DCs-based vaccine can be a way to acquire long-term immunity.
Figure 3. DC-based vaccine with ex vivo loading of antigens. Antigen loaded mature DCs are injected back to body, then migrate to secondary lymphoid organ to induce T cells. DCs are not infected by SARS-CoV-2 so that there’s no immunological dysfunction.
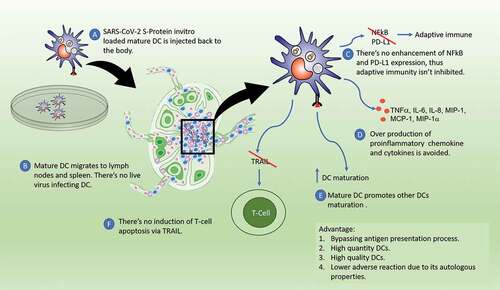
Second, the DCs-based vaccine also might induce broader spectrum immunity. Evidence shows that memory T cell responses remain effective against VoC [Citation91]. DCs-based vaccine can elicit such a response. In addition, DCs can trigger the formation of germinal center (GC) response to form B cells that can recognize variants [Citation92]. DCs induces GC response in two ways: converting naïve T cells into follicular helper T cells and migration of follicular DCs [Citation58]. Thus, DCs-based vaccination is particularly suitable for fighting viruses with high mutation rates such as SARS-CoV-2.
5. Ex vivo approach: challenges and opportunities
Immunization targeting DCs can be done via ex vivo loading and in vivo targeting. In the ex vivo loading approach, antigens are exposed outside the body to DCs isolated from peripheral blood monocyte cells and then injected back into the body [Citation93]. This vaccine is specific and individual. Despite still being experimental, this approach is also currently being developed for SARS-CoV-2 vaccination [Citation94]. In contrast, in vivo targeting, antigen delivery is done by conjugating the desired antigen with antibodies specific to DCs receptors (e.g. anti-CD40 or C-type lectin receptor) [Citation95]. Both of these methods have their respective advantages and disadvantages. However, the ex vivo loading method is still the primary choice in developing dendritic cell-based vaccines. Several dendritic cell vaccine studies that entered the clinical trial employ the ex vivo loading method [Citation7–12,Citation96].
Some studies using ex vivo generated DCs for therapy of infections are summarized in . This approach is mostly developed for chronic infection immunotherapy such as HIV-1, Hepatitis B, Hepatitis C and HSV [Citation13,Citation14,Citation97–99]. Ex vivo generated DCs-based vaccine for prevention of viral infection is deemed unjustifiable due to the high cost of production. This hinders the progress to understand the full scope of ex vivo generated DCs in inducing immunity. However, in the case of COVID-19, the justification to develop this approach still require a comprehensive cost-benefit analysis. The efficacy of currently available COVID-19 vaccines are decreasing overtime, and the emergence of variants are pushing for the discovery of next generation vaccines [Citation4,Citation5]. The potential in inducing long-term and broad immunity by DCs-based vaccine might eliminate the need for frequent booster vaccines, thus in the long run the total cost of production and distribution of ex vivo generated DCs vaccine can be comparable to conventional vaccines. Another aspect that should be considered is the feasibility of wide distribution due to the fact that this vaccine production requires a highly trained staff in specific facilities. However, vaccines made point-of-care such as hospitals and medical laboratories can be the solution to this problem [Citation100]. In conclusion, cost and practical limitation of this approach should not limit the potential benefit ().
Table 1. Studies of DCs-based vaccines for chronic infections.
Table 2. Advantages and disadvantages of Ex vivo loading.
Furthermore, in the context of the SARS-CoV-2 vaccine, the world is in a race against time [Citation101]. Therefore, the ex vivo presentation of antigens is currently an appropriate method. Meanwhile, in vivo DCs targeting still needs to be refined. This method also has the following advantages:
First, the presentation of antigens outside the body will bypass DCs antigen presentation process that is key in initiating the immune response. The process of antigen presentation in the body is highly complex. Not all antigens that enter the body are successfully taken up and presented through DCs. Antigens must meet with DCs directly for the process to occur. This process is influenced by several factors: availability of dendritic cells, the presence of PRR (Pathogen Recognition Receptors), a condition that supports DCs maturation and differentiation, and activation of antigen processing pathways through the corresponding compartment [Citation23,Citation43,Citation49]. Deficiency in one of these factors will make the failure of antigen presentation by DCs. Ex vivo antigen presentation will cut through such a complicated process.
Second, ex vivo antigen presentation results in a sufficient number of mature DCs for T cells priming. A study shows that it takes at least 85 mature DCs to induce T cells response [Citation55]. Moreover, large amounts of antigens are required because the pMHC only lasts for a short time [Citation40]. Appropriate cytokines are also needed as maturation signals [Citation43]. When done outside the body, antigen presentation occurs in a controlled environment, where the number of DCs, maturation signals, and antigens are known. In addition, by isolating DCs ex vivo, the antigen is presented only to the DCs and not other cells. Thus, this method ensures the development of mature DCs in sufficient quantities for T cells priming.
Third, the ex vivo antigen presentation method produces high-quality DCs. DCs can be derived from peripheral blood mononuclear cells when stimulated by IL-4 and GM-CSF [Citation47,Citation102]. In our study, DCs were obtained by incubation of peripheral blood mononuclear cells for 5 days within differentiation media [Citation100]. Those DCs are subsequently incubated with the S-protein of SARS-CoV-2 for 2 days. This process is carefully controlled, and the quality of the DCs can be checked prior to administration. High-quality mature DCs can migrate efficiently to the secondary lymphoid organs, where they initiate the innate and adaptive immune response to SARS-CoV-2. In addition, there is evidence that DCs actively spread antigens to other APCs through cross-dressing [Citation53]. This ability increases the adequate number of APCs and keeps T cell priming from occurring even though the donor DCs cannot directly prime T cells. Whereas in vivo DCs-based vaccination, the quality of DCs is affected by the difficulty of controlling each individual’s state of immunity. For example, there is a decrease in the quality of DCs in people with chronic diseases and the elderly [Citation103–105]. Furthermore, a DCs-related genetic disorder can impair DCs’ function [Citation106]. Therefore, in vivo DCs targeting vaccination in that population might be ineffective.
Fourth, the body can tolerate autologous DCs vaccine with ex vivo antigen presentation. This method is proved safe from previous studies and caused only negligible toxicity [Citation7–12]. Side effects are limited to mild local and systemic reactions such as injection pain, joint pain, and flu-like syndrome. There are no deaths or life-threatening events related to the autologous DCs vaccine. When an antigen enters the body, the immune system activates the signaling pathways distinguishing self and non-self [Citation107]. However, autologous DCs are recognized as a part of the self so that the body can tolerate the material well without a reaction of foreign body rejection.
6. Conclusion
We have outlined that DCs are the most potent APCs that can activate a wide array of T-cell immunity. DCs play a crucial role in innate and adaptive immunity. SARS-CoV-2 can infect DCs resulting in disruption of innate and adaptive immunity. Dendritic cell vaccine using ex vivo approach can result in better clinical outcome.
7. Expert opinion
DCs can activate various T-cells responses and are essential in T-cells fate determination [Citation38]. In innate immunity, pDCs are the primary source of IFN-1. Crosstalk between DCs, NK cells, and macrophages create the cytokine milieu suitable for innate immune responses. Furthermore, DCs are responsible for antigen presentation, which is key to adaptive immune response. T-cells priming by DC is a highly efficient process orchestrated by various cytokines and chemokines for optimal cell positioning. On the other hand, SARS-CoV-2 can infect DCs and causes dysfunction in innate and adaptive immunity [Citation6]. As postulated in this review, this virus can directly infect DCs resulting in abnormal cytokines production, T-cell depletion and fatigue, and adaptive immunity inhibition. Therefore, the vaccination approach by targeting and protecting DCs might result in faster viral clearance and better clinical outcomes.
Here, we have outlined the rationale to develop DCs-based vaccine for prevention of COVID-19. Although mainly developed for treatment of cancer and chronic infections, this method can also be extended for prevention of SARS-CoV-2 infection [Citation7–12,Citation96]. Some previous studies show that DCs based vaccine can induce immunity against viral infection through activation of T-cells immunity [Citation13,Citation14,Citation98,Citation99,Citation108]. Interestingly, even when the immune system is compromised such as in HIV infection, DCs vaccines are still capable to induce T-cell response resulting in reduction of viral load [Citation13,Citation98]. So that, it is possible that DCs vaccine will remain effective for people with weaker immunity, such as children, elderly, and people with chronic illnesses.
DCs are responsible for a wide array of T cell responses, thus utilizing DCs as vaccine carrier might induce a broad and long-term immunity for SARS-CoV-2 prevention [Citation46,Citation89,Citation90]. There is also a possibility of induction of GC response by DCs. Even though the mechanism is still unclear, GCs response can induce production of antibody with broader neutralization capacity through clonal evolution [Citation92]. So that, alongside clinical efficacy, T-cell and GC response elicited by DCs should be taken into consideration in future studies.
Currently, ex vivo loading is the most feasible method for DC-based vaccine development. Most of the studies that entered clinical trial employ this approach [Citation7–12]. There are several advantages to this approach as previously mentioned. This method bypasses DCs antigen presentation in a highly controllable environment so T cells priming can occur. This approach ensures the generation of a high-quality DCs in sufficient quantities for T cells priming. Furthermore, ex vivo loading produces a vaccine with very few adverse reactions due to its autologous properties.
However, the cost of production of this approach is considerably high, but this depends on the number of productions. Vaccine production by this method also requires certain facilities, so wide distribution might not be practical. On the other hand, the possibility of inducing long-term and broader immunity will eliminate the need for frequent boosters. So that, the total cost of production might eventually become cost-effective in comparison to conventional vaccine. Production at point-of-care, such as hospital and medical laboratories, can be the solution to practical limitation of this approach. In conclusion, this approach is appropriate for the COVID-19 vaccine, and the safety profile of this approach should allow clinical study to proceed.
Article highlights
DCs are essential in the innate immune response through the production of IFN-1 and immune cells modulation.
DCs are the most important APCs that activate various T cells immune responses.
SARS-CoV-2 can directly infect DCs causing inadequate innate and adaptive immune response.
COVID-19 vaccination targeting DCs might result in better outcomes and potentially causes long-term and broader spectrum immunity.
Ex vivo loading of antigens is an appropriate approach in manufacturing DCs-based vaccines for COVID-19. Because this approach bypasses the rigorous process of antigen presentation inside the body, results in high quality and quantity antigen-loaded mature DCs and has a well-established safety profile.
Declaration of interest
The authors have no relevant affiliations or financial involvement with any organization or entity with a financial interest in or financial conflict with the subject matter or materials discussed in the manuscript. This includes employment, consultancies, honoraria, stock ownership or options, expert testimony, grants or patents received or pending, or royalties.
Reviewer disclosures
Peer reviewers on this manuscript have no relevant financial or other relationships to disclose.
Additional information
Funding
References
- Zhu N, Zhang D, Wang W, et al. A novel coronavirus from patients with Pneumonia in China, 2019. N Engl J Med. 2020;382:727–733.
- Randolph HE, Barreiro LB. Herd immunity: understanding COVID-19. Immunity. 2020;52:737–741.
- Lopez Bernal J, Andrews N, Gower C, et al. Effectiveness of Covid-19 vaccines against the B.1.617.2 (Delta) variant. N Engl J Med. 2021;385:585–594.
- Sabino EC, Buss LF, Carvalho MPS, et al. Resurgence of COVID-19 in Manaus, Brazil, despite high seroprevalence. Lancet. 2021;397:452–455.
- Wang P, Nair MS, Liu L, et al. Antibody resistance of SARS-CoV-2 variants B.1.351 and B.1.1.7. Nature. 2021;593:130–135.
- Borges RC, Hohmann MS, Borghi SM. Dendritic cells in COVID-19 immunopathogenesis: insights for a possible role in determining disease outcome. Int Rev Immunol. 2021;40:108–125. 1–2
- Jiang N, Qiao G, Wang X, et al. Dendritic Cell/Cytokine-Induced killer cell immunotherapy combined with S-1 in patients with advanced pancreatic cancer: a prospective study. Clin Cancer Res. 2017;23:5066–5073.
- Zhang W, Lu X, Cui P, et al. Phase I/II clinical trial of a Wilms’ tumor 1-targeted dendritic cell vaccination-based immunotherapy in patients with advanced cancer. Cancer Immunol Immunother. 2019;68:121–130.
- Liau LM, Ashkan K, Tran DD, et al. First results on survival from a large phase 3 clinical trial of an autologous dendritic cell vaccine in newly diagnosed glioblastoma. J Transl Med. 2018;16:142.
- Wang Q-T, Nie Y, Sun S-N, et al. Tumor-associated antigen-based personalized dendritic cell vaccine in solid tumor patients. Cancer Immunol Immunother. 2020;69:1375–1387.
- Bulgarelli J, Tazzari M, Granato AM, et al. Dendritic cell vaccination in metastatic melanoma turns “Non-T Cell inflamed” Into “T-Cell inflamed” Tumors. Front Immunol. 2019;10:2353.
- Lee JM, Lee M-H, Garon E, et al. Phase I Trial of Intratumoral Injection of CCL21 Gene-Modified dendritic cells in lung cancer elicits Tumor-Specific immune responses and CD8(+) T-cell infiltration. Clin Cancer Res. 2017;23:4556–4568.
- Lu W, Arraes LC, Ferreira WT, et al. Therapeutic dendritic-cell vaccine for chronic HIV-1 infection. Nat Med. 2004;10:1359–1365.
- Chen M, Y-G L, Zhang D-Z, et al. Therapeutic effect of autologous dendritic cell vaccine on patients with chronic hepatitis B: a clinical study. World J Gastroenterol. 2005;11:1806–1808.
- Decker WK, Safdar A. Dendritic cell vaccines for the immunocompromised patient: prevention of influenza virus infection. Expert Rev Vaccines. 2010;9:721–730.
- Marshall JS, Warrington R, Watson W, et al. An introduction to immunology and immunopathology. Allergy, Asthma Clin Immunol. 2018;14:5–14.
- Varadé J, Magadán S, González-Fernández Á. Human immunology and immunotherapy: main achievements and challenges. Cell Mol Immunol. 2021;18:805–828.
- Stambas J, Lu C, Tripp RA. Innate and adaptive immune responses in respiratory virus infection: implications for the clinic. Expert Rev Respir Med. 2020;14:1141–1147.
- Riera Romo M, Pérez-Martínez D, Castillo Ferrer C. Innate immunity in vertebrates: an overview. Immunology. 2016 April 05. 2016;148:125–139.
- Bieber K, Autenrieth SE. Dendritic cell development in infection. Mol Immunol. 2020;121:111–117.
- Ivashkiv LB, Donlin LT. Regulation of type I interferon responses. Nat Rev Immunol. 2014;14:36–49.
- Mitchell D, Chintala S, Dey M. Plasmacytoid dendritic cell in immunity and cancer. J Neuroimmunol. 2018;322:63–73.
- Amon L, Lehmann CHK, Baranska A, et al. Transcriptional control of dendritic cell development and functions. 1st (Amsterdam, Nedherland: Elsevier Inc) ed. Int. Rev. Cell Mol. Biol. Elsevier Inc.; 2019.
- Reizis B. Plasmacytoid dendritic cells: development, regulation, and function. Immunity. 2019;50:37–50.
- Oth T, Vanderlocht J, Van Elssen CHMJ, et al. Pathogen-Associated molecular patterns induced crosstalk between dendritic cells, T helper cells, and natural killer helper cells can improve dendritic cell vaccination. Mediators Inflamm. 2016; 2016. 1–12
- Lee AJ, Ashkar AA. The dual nature of type I and type II interferons. Front Immunol. 2018. p. 2061. 9
- Kwon B. IFN-γ in tissue-immune homeostasis and antitumor immunity. Cell Mol Immunol. 2018;15:531–532.
- Oth T, Habets THPM, Germeraad WTV, et al. Pathogen recognition by NK cells amplifies the pro-inflammatory cytokine production of monocyte-derived DC via IFN-γ. BMC Immunol. 2018;19:1–13.
- Kang S, Kumanogoh A. The spectrum of macrophage activation by immunometabolism. Int Immunol. 2020;32:467–473.
- Gupta U, Hira SK, Singh R, et al. Essential role of TNF-α in gamma c cytokine aided crosstalk between dendritic cells and natural killer cells in experimental murine lymphoma. Int Immunopharmacol. 2020;78:106031.
- Kobayashi S, Sakurai T, So T, et al. TNF Receptor–Associated factor 5 limits function of plasmacytoid dendritic cells by controlling IFN regulatory factor 5 expression. J Immunol. 2019;203:1447–1456.
- Liu S-Q, Ren C, Yao R-Q, et al. TNF-α-induced protein 8-like 2 negatively regulates the immune function of dendritic cells by suppressing autophagy via the TAK1/JNK pathway in septic mice. Cell Death Dis. 2021 12 ;1032.
- Murphy K, Weaver C. Janeway’s immunobiology. 9th ed. New York and London: Garland Science : Taylor & Francis Group; 2017.
- Netea MG, Schlitzer A, Placek K, et al. Innate and adaptive immune memory: an evolutionary continuum in the hosts response to pathogens. Cell Host Microbe. 2019;25:13–26.
- Sun L, Wang X, Saredy J, et al. Innate-adaptive immunity interplay and redox regulation in immune response. Redox Biol. 2020;37:101759.
- Normann N, Tietz G, Kühn A, et al. Pathogen-specific antibody profiles in patients with severe systemic infections. Eur Cells Mater. 2020;39:171–182.
- Balan S, Saxena M, Bhardwaj N. Dendritic cell subsets and locations. 1st (Amsterdam, Netherland: Elsevier Inc) ed. Int. Rev. Cell Mol. Biol. Elsevier Inc. 2019.
- Constantino J, Gomes C, Falcão A, et al. Dendritic cell-based immunotherapy: a basic review and recent advances. Immunol Res. 2017;65:798–810.
- Collin M, Bigley V. Human dendritic cell subsets: an update. Immunology. 2018;154:3–20.
- Villadangos JA, Schnorrer P. Intrinsic and cooperative antigen-presenting functions of dendritic-cell subsets in vivo. Nat Rev Immunol. 2007;7:543–555.
- Alculumbre SG, Saint-André V, Di Domizio J, et al. Diversification of human plasmacytoid predendritic cells in response to a single stimulus article. Nat Immunol. 2018;19:63–75.
- Worbs T, Hammerschmidt SI, Förster R. . Nat. Rev. Immunol. (Berlin, Germany: Nature Publishing Group); 2017. p. 30–48.
- Hilligan KL, Ronchese F. Antigen presentation by dendritic cells and their instruction of CD4+ T helper cell responses. Cell Mol Immunol. 2020;17:587–599.
- Fitzgerald KA, Kagan JC. Toll-like receptors and the control of immunity. Cell. 2020;180:1044–1066.
- Kim MK, Kim J. Properties of immature and mature dendritic cells: phenotype, morphology, phagocytosis, and migration. RSC Adv. 2019;9:11230–11238.
- Shin KS, Jeon I, Kim BS, et al. Monocyte-derived dendritic cells dictate the memory differentiation of CD8+ T cells during acute infection. Front Immunol. 2019;10:5–7.
- Gu Y, Zhao X, rong SX. Ex vivo pulsed dendritic cell vaccination against cancer. Acta Pharmacol Sin. 2020;41:959–969.
- Blum JS, Wearsch PA, Cresswell P. Pathways of antigen processing. Annu Rev Immunol. 2013;31:443–473.
- Roche PA, Cresswell P. . Myeloid Cells Heal Dis A Synth (Wiley Online Book). 2017;209–223:9781683670667 doi:10.1128/9781555819194.ch11.
- Lindenbergh MFS, Stoorvogel W. Antigen presentation by extracellular vesicles from professional Antigen-Presenting cells. Annu Rev Immunol. 2018;36:435–459.
- Tiberio L, Del Prete A, Schioppa T, et al. Chemokine and chemotactic signals in dendritic cell migration review-article. Cell Mol Immunol. 2018;15. 15
- Ugur M, Mueller SN. T cell and dendritic cell interactions in lymphoid organs: more than just being in the right place at the right time. Immunol Rev. 2019;289:115–128.
- Gurevich I, Feferman T, Milo I, et al. Active dissemination of cellular antigens by DCs facilitates CD8+ T-cell priming in lymph nodes. Eur J Immunol. 2017;47:1802–1818.
- Miller MJ, Hejazi AS, Wei SH, et al. T cell repertoire scanning is promoted by dynamic dendritic cell behavior and random T cell motility in the lymph node. Proc Natl Acad Sci U S A. 2004;101:998–1003.
- Celli S, Day M, Müller AJ, et al. How many dendritic cells are required to initiate a T-cell response? Blood. 2012;120:3945–3948.
- Schmidt ME, Varga SM. The CD8 T cell response to respiratory virus infections. Front Immunol. 2018;9. 10.3389/fimmu.2018.00678
- Reed J, Wetzel SA. CD4 + T cell differentiation and activation. Methods Mol Biol. 2018 1803 . p. 335–351.
- Koutsakos M, Nguyen THO, Kedzierska K. With a little help from T follicular helper friends: humoral immunity to influenza vaccination. J Immunol. 2019;202:360–367.
- Hirano T, Murakami M. COVID-19: a new virus, but a familiar receptor and cytokine release syndrome. Immunity. 2020;52:731–733.
- Wang K, Chen W, Zhang Z, et al. CD147-spike protein is a novel route for SARS-CoV-2 infection to host cells. Signal Transduct Target Ther. 2020;1–10. 5
- Taefehshokr N, Taefehshokr S, Hemmat N, et al. Covid-19: perspectives on innate immune evasion. Front Immunol. 2020;11:580641.
- Cai G, Du M, Boss Y, et al. SARS-CoV-2 impairs dendritic cells and regulates DC-SIGN gene expression in tissues. Int J Mol Sci. 2021;22:1–20.
- Chu H, Zhou J, Ho-Yin Wong B, et al. Productive replication of middle East respiratory syndrome coronavirus in monocyte-derived dendritic cells modulates innate immune response. Virology. 2014;454–455:197–205.
- Yang D, Chu H, Hou Y, et al. Attenuated interferon and proinflammatory response in SARS-CoV-2-infected human dendritic cells is associated with viral antagonism of STAT1 phosphorylation. J Infect Dis. 2020;222:734–745.
- Chi Y, Ge Y, Wu B, et al. Serum cytokine and chemokine profile in relation to the severity of coronavirus disease 2019 in China. J Infect Dis. 2020;222:746–754.
- Huang C, Wang Y, Li X, et al. Clinical features of patients infected with 2019 novel coronavirus in Wuhan, China. Lancet. 2020;395:497–506.
- Tiwari-Heckler S, Rauber C, Longhi MS, et al. Dysregulated host response in severe acute respiratory syndrome coronavirus 2-Induced critical illness. Open Forum Infect Dis. 2021;8:1–9.
- Niles MA, Gogesch P, Kronhart S, et al. Macrophages and dendritic cells are not the major source of Pro-Inflammatory cytokines upon SARS-CoV-2 infection. Front Immunol. 2021;12:1–12.
- Schulte-Schrepping J, Reusch N, Paclik D, et al. Severe COVID-19 is marked by a dysregulated myeloid cell compartment. Cell. 2020;182:1419–1440.e23.
- Zhou R, Kkw T, Wong YC, et al. Acute SARS-CoV-2 infection impairs dendritic cell and T cell responses. Immunity. 2020;53:1–14.
- Blanco-Melo D, Nilsson-Payant BE, Liu WC, et al. Imbalanced host response to SARS-CoV-2 drives development of COVID-19. Cell. 2020;181:1036–1045.e9.
- Ribero MS, Jouvenet N, Dreux M, et al. Interplay between SARS-CoV-2 and the type I interferon response. PLoS Pathog. 2020;16:1–22.
- Peiris JSM, Chu CM, Cheng VCC Clinical progression and viral load in a community outbreak of coronavirus-associated SARS pneumonia: a prospective study , et al. Lancet. , . 2003;361:1767–1772.
- Channappanavar R, Fehr AR, Vijay R, et al. Dysregulated Type I interferon and inflammatory Monocyte-Macrophage responses cause lethal Pneumonia in SARS-CoV-Infected mice. Cell Host Microbe. 2016;19:181–193.
- Zhang Q, Liu Z, Moncada-Velez M, et al. Inborn errors of type I IFN immunity in patients with life-threatening COVID-19. Science (80). 2020;370.
- Galati D, Zanotta S, Capitelli L, et al. A bird’s eye view on the role of dendritic cells in SARS-CoV-2 infection: perspectives for immune-based vaccines. Allergy. 2021: 00. p. 1–11.
- Bastard P, Rosen LB, Zhang Q, et al. Autoantibodies against type I IFNs in patients with life-threatening COVID-19. Science (80). 2020;370.
- Chen Z, John Wherry E. T cell responses in patients with COVID-19. Nat Rev Immunol. 2020;20:529–536.
- Du RH, Liang LR, Yang CQ, et al. Predictors of mortality for patients with COVID-19 pneumonia caused by SARSCoV- 2: a prospective cohort study. Eur Respir J. 2020;3:55.
- Xu B, yu FC, lu WANGA, et al. Suppressed T cell-mediated immunity in patients with COVID-19: a clinical retrospective study in Wuhan, China. J Infect. 2020;81:e51–e60.
- Diao B, Wang C, Tan Y, et al. Reduction and functional exhaustion of T cells in patients with coronavirus disease 2019 (COVID-19). Front Immunol. 2020. p. 827. 11
- Chen J, Qi T, Liu L, et al. Clinical progression of patients with COVID-19 in Shanghai, China. J Infect. 2020;80:e1–e6.
- Parackova Z, Zentsova I, Bloomfield M. Disharmonic inflammatory signatures in COVID-19 : augmented neutrophils’ but impaired monocytes’ and dendritic cells’ responsiveness. Cells. 2020;9. 10 2206
- Law HKW, Cheung CY, Sia SF, et al. Toll-like receptors, chemokine receptors and death receptor ligands responses in SARS coronavirus infected human monocyte derived dendritic cells. BMC Immunol. 2009;10:1–12.
- Zhu L, Yang P, Zhao Y, et al. Single-Cell sequencing of peripheral mononuclear cells reveals distinct immune response landscapes of COVID-19 and influenza patients. Immunity. 2020;53:685–696.e3.
- von Karstedt S, Montinaro A, Walczak H. Exploring the TRAILs less travelled: TRAIL in cancer biology and therapy. Nat Rev Cancer. 2017;17:352–366.
- Catanzaro M, Fagiani F, Racchi M, et al. Immune response in COVID-19: addressing a pharmacological challenge by targeting pathways triggered by SARS-CoV-2. Signal Transduct Target Ther. 2020;5. 10.1038/s41392-020-0191-1
- DeDiego ML, Nieto-Torres JL, Regla-Nava JA, et al. Inhibition of NF- B-Mediated inflammation in severe acute respiratory syndrome Coronavirus-Infected mice increases survival. J Virol. 2014;88:913–924.
- Jung JH, Rha MS, Sa M, et al. SARS-CoV-2-specific T cell memory is sustained in COVID-19 convalescent patients for 10 months with successful development of stem cell-like memory T cells. Nat Commun. 2021;12:1–12.
- Le Bert N, Tan AT, Kunasegaran K, et al. SARS-CoV-2-specific T cell immunity in cases of COVID-19 and SARS, and uninfected controls. Nature. 2020;584:457–462.
- Tarke A, Sidney J, Methot N, et al. Impact of SARS-CoV-2 variants on the total CD4+ and CD8+ T cell reactivity in infected or vaccinated individuals. Cell Reports Med. 2021;2:100355.
- Laidlaw BJ, Ellebedy AH. The germinal centre B cell response to SARS-CoV-2. Nat Rev Immunol. 2022;22:7–18.
- Sabado RL, Balan S, Bhardwaj N. Dendritic cell-based immunotherapy. Cell Res. 2017;27:74–95.
- Martínez L, Malaina I, Salcines-Cuevas D, et al. First computational design using lambda-superstrings and in vivo validation of SARS-CoV-2 vaccine. Sci Rep. 2022;12:2–13.
- Tacken PJ, De Vries IJM, Torensma R, et al. Dendritic-cell immunotherapy: from ex vivo loading to in vivo targeting. Nat Rev Immunol. 2007. p. 790–802. 7
- Dillman RO, Cornforth AN, Nistor GI, et al. Randomized phase II trial of autologous dendritic cell vaccines versus autologous tumor cell vaccines in metastatic melanoma: 5-year follow up and additional analyses. J Immunother Cancer. 2018;6:19.
- Wang X, Bayer ME, Chen X, et al. Phase I trial of active specific immunotherapy with autologous dendritic cells pulsed with autologous irradiated tumor stem cells in hepatitis B-positive patients with hepatocellular carcinoma. J Surg Oncol. 2015;111:862–867.
- Surenaud M, Montes M, Lindestam Arlehamn CS, et al. Anti-HIV potency of T-cell responses elicited by dendritic cell therapeutic vaccination. PLoS Pathog. 2019;15:1–21.
- Leplina O, Starostina N, Zheltova O, et al. Dendritic cell-based vaccines in treating recurrent herpes labialis: results of pilot clinical study. Hum Vaccines Immunother. 2016;12:3029–3035.
- Jonny J. Preventive dendritic cell vaccine, AV-COVID-19, in subjects not actively infected with COVID-19 [Internet]. Identifier NCT00103181. cited 2022 Apr 27]. Available from 2022 Apr 27: https://clinicaltrials.gov/ct2/show/NCT05007496.
- Khuroo MS, Khuroo M, Khuroo MS, et al. COVID-19 vaccines: a race against time in the middle of death and devastation! J Clin Exp Hepatol. 2020;10:610–621.
- Sung SSJ. Monocyte-derived dendritic cells as antigen-presenting cells in T-cell proliferation and cytokine production. Methods Mol Biol. 2019;2020:131–141.
- Wong C, Goldstein DR. Impact of aging on antigen presentation cell function of dendritic cells. Curr Opin Immunol. 2013;25:535–541.
- Zacca ER, Crespo MI, Acland RP, et al. Aging Impairs the ability of conventional dendritic cells to cross-prime CD8+ T cells upon stimulation with a TLR7 Ligand. PLoS One. 2015;10:1–20.
- Li S, Wu J, Zhu S, et al. Disease-associated plasmacytoid dendritic cells. Front Immunol. 2017;8:1–12.
- Asano T, Immunol S, Asano T, et al. X-linked recessive TLR7 deficiency in ~ 1 % of men under 60 years old with life-threatening COVID-19. Sci Immunol. 2021;4348:1–31.
- Forsdyke DR. Lymphocyte repertoire selection and intracellular self/non-self-discrimination: historical overview. Immunol Cell Biol. 2015;93:297–304.
- Mekonnen ZA, Masavuli MG, Yu W, et al. Enhanced T cell responses induced by a necrotic dendritic cell vaccine, expressing HCV NS3. Front Microbiol. 2020;11:1–13.