ABSTRACT
Objectives
A number of vaccines have now been developed against COVID-19. Differences in reactogenicity and safety profiles according to the vaccine technologies employed are becoming apparent from clinical trials.
Methods
Five databases (Medline, EMBASE, Science Citation Index, Cochrane Central Register of Controlled Trials, London School of Hygiene and Tropical Medicine COVID-19 vaccine tracker) were searched for relevant randomized controlled trials between 1 January 2020 and 12 January 2022 according to predetermined criteria with no language limitations.
Results
Forty-two datasets were identified, with 20 vaccines using four different technologies (viral vector, inactivated, mRNA and protein sub-unit). Adults and adolescents over 12 years were included. Control groups used saline placebos, adjuvants, and comparator vaccines. The most consistently reported solicited adverse events were fever, fatigue, headache, pain at injection site, redness, and swelling. Both doses of mRNA vaccines, the second dose of protein subunit and the first dose of adenovirus vectored vaccines were the most reactogenic, while the inactivated vaccines were the least reactogenic.
Conclusions
The different COVID-19 vaccines currently available appear to have distinct reactogenicity profiles, dependent on the vaccine technology employed. Awareness of these differences may allow targeted recommendations for specific populations. Greater standardization of methods for adverse event reporting will aid future research in this field.
1. Introduction
Severe acute respiratory syndrome coronavirus 2 (SARS-CoV-2) infection (COVID-19) has been associated with more than 513 million cases and 6.2 million deaths worldwide as of 6 May 2022 [Citation1]. To control this pandemic, safe and effective vaccines were developed rapidly and several vaccine candidates have emerged. Currently, 344 COVID-19 vaccine candidates are in various stages of development and 126 candidates have reached clinical trials [Citation2]. Thirty-eight vaccines have now been approved for emergency use in 197 countries [Citation3].
Different strategies and technologies have been utilized for the development of vaccines against SARS-CoV-2. While these include conventional approaches such as inactivated and protein subunit vaccines, they also include novel technologies such as messenger ribonucleic acid (mRNA) and viral-vectored vaccines. Already, clear differences in the efficacy of these vaccines are emerging from clinical trials [Citation4,Citation5] and it is also apparent that the reactogenicity and safety profiles differ according to the platform employed. A detailed understanding of the side effect profiles of different vaccines is required for decisions to be made about their deployment and in informing the general public about the risk-benefit ratios of vaccination. Additionally, such data may have implications for the populations in which they are to be used, for example, vaccines associated with low rates of adverse events (AEs) may be prioritized for use in pregnant women, young children, the immunocompromised and the elderly.
An early systematic review and meta-analysis of COVID-19 vaccines by Yuan et al. [Citation6] (to October 2020) showed that there were significant differences between vaccine and placebo recipients in terms of local and systemic AEs. Pormohammad et al. [Citation5] concluded that mRNA-based vaccines had the highest level of side effects (except for diarrhea and arthralgia) and aluminum-adjuvanted vaccines had the lowest side effect profile (except for injection site redness). Another review by Ling et al. [Citation4] concluded that the incidence of adverse reactions was highest for the adenovirus vector vaccines. Chen et al. [Citation7] drew a similar conclusion and also observed that the overall incidence of adverse events was higher for vaccinees aged 16–55 years than older adults (aged over 55 years), an observation also reported in the systematic review by Wang et al. [Citation8]. The most recently published meta-analyses of the safety of COVID-19 vaccines (to 17 June 2021) concluded that all vaccines increased the risk of non-serious AEs. Due to the inconsistencies reported in past reviews and the speed at which new data in this area is published, we undertook a systematic review and meta-analysis of the reactogenicity of COVID-19 vaccines assessed in randomized controlled trials (RCTs), with a focus on commonly reported systemic and local AEs.
2. Methods
2.1. Database and search terms
A systematic review and meta-analysis was carried out to compare the reactogenicity of COVID-19 vaccines developed using different technologies: viral vector, mRNA, inactivated and protein subunit vaccines. The following databases were searched: Medline, EMBASE, Science Citation Index (Web of Science), Cochrane Central Register of Controlled Trials (CENTRAL) and the London School of Hygiene and Tropical Medicine (LSHTM) COVID-19 vaccine tracker [Citation2]. Medical subject headings (MESH) terms and free text synonyms were used to search the databases for the following search themes: ‘vaccines,’ ‘reactogenicity,’ and ‘COVID-19.’ The Cochrane highly sensitive search strategy was used to narrow the search results to RCTs [Citation9]. The search results were limited to human studies published between January 2020 – 12 January 2022. The full search strategy for each database can be found in supplementary Table S1.
This review was registered in the PROSPERO International prospective register of systematic reviews (13 April 2021, PROSPERO 2021 CRD42021248766).
2.2. Systematic review: inclusion and exclusion criteria
The search results were imported into the web application Rayyan, a recommended screening tool for systematic reviews [Citation10]. Duplications were removed and the remaining papers were independently assessed in duplicate by NS, ASFR and EB against the inclusion and exclusion criteria, disagreements were resolved by consensus. Papers were deemed suitable for inclusion if they described a blinded randomized control trial of a COVID-19 vaccine, in participants aged 12 years and over, with either a placebo or control arm. Only studies of vaccines that were in active phase III clinical trials (recruitment or follow-up) before the 6th of January 2022, according to the LSHTM vaccine tracker [Citation11] were included. A full list of eligible vaccines can be found in supplementary Table S2. Studies were excluded if they described only i) heterologous or booster regimes, ii) immunogenicity or efficacy, iii) the study protocol, vi) vaccines that were not administered intramuscularly, and v) COVID-19 vaccines which were co-administered with other vaccines. Pre-print papers, not yet peer-reviewed and listed on the LSHTM tracker were not included.
2.3. Risk of bias assessment
Each paper was independently assessed in duplicate for risk of bias by NS, ASFR, EB, SI, DS, and YH using the Revised Cochrane risk-of-bias tool for randomized trials (RoB 2) [Citation12]. Any discrepancies were resolved by discussion between the two authors. If they were unable to resolve their differences, discrepancies were resolved by discussion with the rest of the review team. Studies which were assessed to have a high risk of bias were not included in the meta-analysis, and only data regarding study characteristics were extracted for these papers.
2.4. Data extraction
The following data regarding the study characteristics were extracted: the countries where the trials were conducted, participant characteristics (age, sex, and ethnicity), vaccine characteristics (vaccine platform, dose, and schedule) and the placebo or control used. For the studies which were assessed to have a ‘low’ or ‘some concerns’ risk of bias, data on reactogenicity were extracted. For each dose of vaccine or control, the number of participants who experienced fever, fatigue, headache, pain at injection site, redness, swelling, any local AE, any systemic AE or any AEs were extracted. Although differences in the grading of AEs used in the different trials were small, in order to minimize any potential bias, data was analyzed for ‘all’ AEs in each category and not further categorized by AE grade. If the trials reported data on different doses of vaccines, only data related to the dose that was taken forward into Phase 3 trials were collected.
2.5. Missing data requested from authors
For papers which did not report the reactogenicity separately for each vaccine dose administered, or papers where the data were only presented in graphs, the authors were contacted to request the data. Raw data received by authors were summarized in R studio statistical software (version 1.4.1717). The data received from authors had two potential denominators – number of participants who received a vaccine dose and should have completed the reactogenicity diary and number of participants who completed any part of the diary (per-protocol). We used the former in our analysis to remain consistent with the approach taken by other papers included in this review. When data was not provided by authors but was available in graph format in the manuscript, a web-based plot digitizer tool (WebPlotDigitiser V 4.5) [Citation13] was used for data extraction.
2.6. Statistical analysis
The descriptive analyses were performed and summarized using percentage, frequency, and median with minimum-maximum ranges. Meta-analysis was carried out in RevMan Version 5.4. The studies were divided into two groups: studies which had data for each vaccine dose and studies in which the data were combined for the whole vaccine course. Single dose vaccines were included in the analysis of studies where data were available by dose. The Mantel-Haenszel random-effects model was performed to estimate risk ratios (RRs) and 95% confidence intervals (CIs) for each symptom by vaccine platform for dose 1 and dose 2, or all doses combined. This model was selected because of the high potential heterogeneity across trials. Low, moderate, and high heterogeneity were defined as I2 values of 25%, 50%, and 75%, respectively [Citation14].
2.7. Sensitivity analysis
A sensitivity analysis was carried out comparing intention to treat (ITT) and per-protocol populations by plotting separate forest plots with the same denominators for the first and second doses. In the per-protocol population, the denominators for participants who received dose one and dose two were different as some participants did not receive the second dose. In the ITT population, the denominators for doses one and two were the same, based on the number of participants who received dose one of the vaccine. If there was no significant difference between the two analyses, the per-protocol data was reported in order to be consistent with the published trial reports.
2.8. Investigating heterogeneity and publication bias
Factors that could contribute to high heterogeneity were identified as age, phase of vaccine trial, type of placebo or active control used by different trials, and multiple vaccines being included in each vaccine platform group. Four additional groups of forest plots were created which included only adult participants aged 16–65 years, only trials which used a placebo control (0.9% saline or water for injection), only phase II and III studies, and only vaccines which had three or more papers published. The I2 statistic was compared to assess which of the factors was contributing to high heterogeneity. Due to lack of granularity in the data, we were unable to perform meta-regression to further investigate heterogeneity in this review.
To assess publication bias of included clinical trials, funnel plots of the RRs against the standard error for each individual study were performed.
2.9. Comparison of control types and analysis of individual vaccines
Further analysis was carried out to assess the impact of the different controls that were used across trials. For the vaccines with trials using different control groups, forest plots were constructed with each control group as an independent subgroup. Additional forest plots were constructed to compare individual vaccines which had at least three papers published.
3. Results
The database search was carried out on 12 January 2022 and yielded 1335 results. After duplications were deleted, 865 papers were screened. See for the study selection flowchart. Forty-eight papers describing 20 vaccines met the inclusion criteria for the review and underwent a risk of bias assessment. Most papers were assessed as having a low risk of bias, six had some concerns [Citation15–20] and four had a high risk of bias [Citation21–24]. A breakdown of the risk of bias assessment for each paper can be found in the supplementary Table S3. Seven papers were excluded from the meta-analysis: four [Citation21–24] due to a high risk of bias and three [Citation25–27] due to missing data which was not available from the authors. One of the papers [Citation28] included data on two vaccines, resulting in 42 datasets describing 17 vaccines. Thirty-two of the datasets[Citation15–18,Citation20,Citation29–55] presented data divided by dose, whereas ten [Citation19,Citation28,Citation56–61] combined data for all doses.
Of the 20 vaccines included in the systematic review, the vaccine platforms were: viral vector (4), inactivated (6), mRNA (3), and protein sub-unit (7). For the control groups, 29 of the trials used a placebo (0.9% saline, water for injection, or vaccine excipients), 18 used an adjuvant (aluminum hydroxide, Algel, or Algel-IMDG) and two used a Meningococcal ACWY (MenACWY) conjugate vaccine. Most trials recruited adults over the age of 18 years. Four trials included adolescent participants (aged over 12 years) [Citation38,Citation41,Citation44,Citation62]. Overall, there was an equal mix of male and female participants in the trials, although three trials had less than 30% female trial participants [Citation28,Citation31,Citation49]. The trials took place across all continents (see ), which resulted in a mix of ethnicities among participants. See for a summary of the study characteristics.
Figure 2. World map of countries hosting vaccine trial sites by vaccine type (note: some trials took place in more than one country).
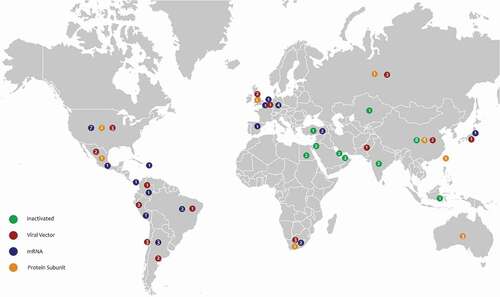
Table 1. Study characteristics.
Analysis of solicited AEs focused on the six symptoms which were consistently reported in most papers: fever, fatigue, headache, pain at injection site, redness, and swelling. There was variability in the number of local and systemic AEs that were solicited in the individual trials (median four [range 3–7] and eight [range 4–16] respectively). For this reason, data on total local AEs, total systemic AEs and total ‘any AEs’ were not analyzed.
3.1. Systemic adverse events
3.1.1. Fever
shows the RR of developing fever, as compared with the control, by each vaccine type, for those papers that divided data by dose. The overall RR of developing fever after any vaccine type was 4.21 (95% Confidence Interval [CI] 2.56–6.94). The mRNA vaccines had the highest RR for fever, especially after the second vaccination: 6.64 (95% CI 2.21–19.96) after dose one and 31.17 (95% CI 15.91–61.05) after dose two, compared to 5.97 (95% CI 2.95–12.09) for dose one of the adenovirus-vectored vaccines and 5.61 (95% CI 1.94–16.23) for dose two of the protein subunit vaccines. Inactivated vaccines had the lowest RR of fever against control for both doses (1.38 [95% CI 1.05–1.81] for dose 1 and 1.10 [95% CI 0.82–1.47] for dose two), while the risk after dose one for the adenovirus vectored (1.52 [95% CI 0.89–2.59]) and protein subunit vaccines (1.14 [95% CI 0.85–1.54]), was no greater than that of the control group.
3.1.2. Fatigue
Data on fatigue according to dose is shown in . Overall, pooled RR for fatigue after any dose was 1.69 (95% CI 1.50–1.90). A second dose of vaccine was associated with a higher RR for both mRNA (1.54 [95% CI 1.31–1.82] for dose 1 and 2.65 [95% CI 2.44–2.87] for dose 2) and protein subunit vaccines (1.14 [95% CI 1.10–1.19] and 2.00 [95% CI 1.63–2.45]). In contrast, adenovirus-vectored vaccines showed similar RRs for dose one (1.68 [95% CI 1.50–1.59]) and dose two (1.40 [95% CI 1.25–1.57]). RR for fatigue in the inactivated vaccine studies were not different to control for either dose one or two (1.34 [95% CI 0.97–1.86] and 1.27 [95% CI 0.68–2.34] respectively).
3.1.3. Headache
The RR of headache for all subgroups was 1.59 (95% CI 1.41–1.80). Data divided by dose () show that the second dose of mRNA vaccines had the highest RR (2.63 [95% CI 2.42–2.85]) compared to 1.44 [95% CI 1.16–1.77] for the first dose. An increase between doses was also seen for protein subunit vaccines (1.09 [95% CI 1.05–1.14] for dose one and 1.73 [95% CI 1.33–2.23] for dose two) while it was similar between doses for the adenovirus-vectored vaccines (1.64 [95% CI 1.47–1.83] for dose one and 1.38 [95% CI 1.24–1.54] for dose two). Overall, inactivated vaccines had smaller RRs (1.21 [95% CI 1.00–1.45] and 1.18 [95% CI 0.91–1.55] for the first and second doses respectively).
3.2. Local adverse events
3.2.1. Pain at the injection site
Data for localized pain divided by dose is presented in . Overall risk of developing pain at the injection site versus control after any dose of vaccine was 3.30 [95% CI 2.92–3.72]. Second doses of mRNA (5.22 [95% CI 4.04–6.74]) and protein subunit vaccines (4.67 [95% CI 3.76–5.80]) had the highest RR, followed by mRNA first dose (4.75 [95% CI 3.62–6.24]), adenovirus-vectored vaccines (3.59 [95% CI 2.65–4.88] for first dose and 2.62 [95% CI 1.53–4.49] for second dose) and the second dose of protein subunit vaccines 3.23 [95% CI 2.78–3.76]. Inactivated vaccines had the lowest RRs (1.38 [95% CI 1.11–1.72] for dose one and 1.19 [95% CI 0.96–1.46] for dose 2).
3.2.2. Redness
RR of redness at the injection site after any vaccine dose versus control was 3.71 [95% CI 2.52–5.48]. Data divided by dose are shown in . Adenovirus vaccines had comparable rates following each dose (1.77 [95% CI 0.96–3.27] and 2.03 [95% CI 1.46–2.81] respectively). Conversely, there was a marked increase in the risk of redness compared to control for mRNA (first dose 6.32 [95% CI 3.69–10.82] versus second dose 12.77 [95% CI 7.64–21.34]) and protein subunit vaccines (first dose 3.39 [95% CI 2.37–4.83] versus second dose 18.78 [95% CI 10.82–32.57]). Inactivated vaccines did not show statistically significant RRs for redness compared to controls.
3.2.3. Swelling
Data analysis divided by dose is shown in forest plot 6. Inactivated vaccines had non-significant RR versus placebo for both doses and RR were similar for adenovirus-vectored vaccine dose 1 (2.50 [95% CI 1.29–4.84]) and dose 2 (1.95 [95% CI 1.52–2.50]). mRNA vaccines were at least 10 times more likely to cause local swelling compared to placebo (RR 10.78 [95% CI 7.40–15.70] for dose 1 and 17.06 [95% CI 9.97–29.18] for dose 2). There was a substantial increase in the risk of swelling after the second dose of protein subunit vaccines (18.43 [95% CI 12.47–27.23]) compared to the first (RR 3.42 [95% CI 2.41–4.84]). The RR of swelling for pooled subgroups was 4.23 (95% CI 2.74–6.52).
3.3. Analysis of data not divided by dose
Forest plots for all six symptoms for studies which did not report data divided by dose can be found in the supplement (Forest plots S1-S6). These only included inactivated and protein subunit vaccines. There were non-statistically significant RRs for fever, fatigue, headache, pain at injection site and redness for both vaccine types. For swelling, protein subunit vaccines had an overall RR of 5.87 (95% CI 1.93–17.86) whereas inactivated vaccines showed no statistically significant increased risk compared to control.
3.4. Sensitivity analysis
The sensitivity analyses were performed to compare ITT and per-protocol population for the six selected solicited AEs. Results for sensitivity analyses are presented in the supplement (Forest plots S7-S12). For all six events, there were no significant differences in the RRs for the second doses in the ITT versus the per-protocol populations except for dose two injection site pain for inactivated vaccines (original RR 1.19 [95% CI 0.96–1.46] versus 1.50 [95% CI 1.01–2.24]). This reflects the fact that the vast majority of participants who received a first dose of vaccine went on to receive their scheduled second dose.
3.5. Heterogeneity and publication bias
Forest plots showing I2 values for the following analyses: younger adult population only (16–65 years), 0.9% saline control studies only and phase II/III studies can be found in the supplement (Forest plots S13 – S30). When all studies were plotted, the heterogeneity was moderate to high for adenovirus-vectored vaccines dose one (59–92%), mRNA vaccines dose one (54–97%) and dose two (65–96%), generally lowest for inactivated vaccines (both doses), the first dose of protein subunit vaccines and variable for the remaining subgroups. Heterogeneity could not be calculated for inactivated vaccines (both doses) for the 0.9% saline control analysis due to only one study being in the group. A reduction in the heterogeneity values was seen within each vaccine platform subgroup; the effect was greater when the extremes of age (teenagers and older adults) were removed from the analysis, and the heterogeneity was smaller when Phase I trials or non-0.9% saline-controlled studies were not included in the analysis. See Table S4 in the supplement for a summary of I2 values. The total heterogeneity remained high (90–98%) in all the analyses in keeping with an expected difference in reactogenicity between vaccine types.
Analysis of publication bias of included clinical trials is shown in Funnel plots S1-6 in the supplement. The publication bias was assessed for each individual AE. Funnel plot asymmetry varied for each symptom. One potential reason for this may be due to the high heterogeneity observed between different trials and vaccine platforms.
3.6. Choice of control groups
Most trials for the same vaccine type used the same control for all included trials, for example, all the mRNA and protein subunit vaccine trials used 0.9% saline as a control. Four trials of the ChAdOx-1 (Oxford-AstraZeneca) vaccine used 0.9% saline as the control group [Citation16,Citation33,Citation34,Citation36] and two used a MenACWY vaccine [Citation32,Citation35]. Forest plots for selected symptoms (fatigue and injection site pain) are shown in the supplement (Forest plots S31 and S32). Trials with 0.9% saline control had higher RR of local pain after each vaccine dose compared to those with MenACWY control (dose one: 4.01 [2.71–5.95] vs 1.80 [1.59–2.04]; dose two 3.61 [3.10–4.21] vs 0.90 [0.62–1.330]), with a similar trend for other symptoms (data not shown). One trial of the CoronaVac vaccine [Citation47] used water for injection (WFI) as the control while three [Citation45,Citation46,Citation48] used an adjuvant (aluminum hydroxide). Forest plots for fatigue and pain are shown in the supplement (Forest plots S33 and S34). RRs were smaller for trials which used an adjuvant as the control compared to those that used WFI, although the differences were not statistically significant.
3.7. Individual vaccines
Six individual vaccines had at least three papers with data available for meta-analysis: Ad5-nCov (Cansino, adenovirus-vector), BNT162b2 (Pfizer-BionTech, mRNA), ChAdOx-1 (Oxford-AstraZeneca, adenovirus-vector), CoronaVac (inactivated), mRNA-1273 (Moderna, mRNA) and NVX-CoV2373 (Novavax, protein subunit). The pooled RR were estimated as individual vaccine subgroups rather than vaccine technology (forest plots S35 – S40 in the supplement).
All vaccines except CoronaVac had statistically significant RRs for fatigue against control, with mRNA-1273 (dose one RR 1.36 [95%CI 1.31–1.40] and dose two RR 2.64 [95%CI 2.28–3.06]) and NVX-CoV2373 (dose one RR 1.14 [95%CI 1.09–1.19] and dose two RR 2.19 [95%CI 1.83–2.62]) having higher risk after the second dose compared to the first.
All vaccines were associated with increased risk of fever against control except for CoronaVac (both doses), ChAdOx-1 (second dose), and NVX-CoV2373 (first dose). The second doses of both mRNA vaccines: BNT162b2 (RR 27.26 [95%CI 15.38–48.33] versus 8.88 [95%CI 5.48–14.39]) and mRNA-1273 (RR 25.9 [95%CI 7.78–86.46] versus 2.56 [95%CI 1.90–3.47]) and the protein subunit vaccine NVX-CoV2373 (RR 6.76 [95%CI 2.21–20.60] versus 1.13 [95%CI 0.83–1.52]) had significantly higher risk of fever compared to the first dose.
For headache, all vaccines except CoronaVac had significant RRs against control, which were higher for the second doses of BNT162b2 (2.67 [95%CI 2.43–2.92]), mRNA-1273 (2.46 [95%CI 2.28–2.65]) and NVX-CoV2373 (1.90 [95%CI 1.49–2.43]) compared to the first dose (1.54 [95%CI 1.42–1.66], 1.22 [95%CI 1.18–1.26] and 1.09 [95%CI 1.05–1.14] respectively).
All doses, except for the second dose of CoronaVac, were associated with increased risk of pain at the injection site, with no statistically significant differences between both doses. Analysis for redness showed that all vaccines except CoronaVac had increased risk versus control, statistically higher for dose two in NVX-CoV2373 (17.82 [95%CI 7.57–41.97] versus 3.25 [95%CI 2.26–4.66]) for dose one. Risk of swelling was significantly higher than control for all vaccines except CoronaVac, with increased risk for dose two compared to dose one in mRNA-1273 (28.01 [95%CI 22.21–35.32] versus 14.62 [95%CI 11.64–18.36]) and NVX-CoV2373 (16.81 [95%CI 9.71–29.08] versus 3.01 [2.07–4.38]).
4. Discussion
This systematic review and meta-analysis focuses on data reported in blinded RCTs of COVID-19 vaccines utilizing either placebo or control arms. We believe it is the most comprehensive systematic review and meta-analysis of COVID-19 vaccine reactogenicity available, with data on approximately 200,000 administered vaccine doses. Only studies of vaccines that had reached phase III clinical trials by the start of 2022 were included and thus the results of this review provide a perspective on the reactogenicity of vaccines that are currently relevant to the global community. In contrast to earlier reviews, studies of all relevant vaccine technologies were available for analysis.
Several conclusions can be drawn from these analyses. It is clear that the vaccine type (technology) does influence the likelihood of AEs occurring. This will reflect inherent biological differences between such vaccines (e.g. mRNA vs protein) as well as the inclusion of different adjuvants or vaccine dosage. In general, the mRNA vaccines are associated with the highest risk of AE and the inactivated vaccines with the lowest. This does, however, vary by dose, with higher rates of events after the second dose for both mRNA and protein subunit vaccines (compared to the first dose), higher rates after the first dose for adenovirus vectored vaccines (compared to the second dose) and equivalent rates of AEs after first and second doses for the inactivated vaccines. In fact, for the inactivated vaccines such rates were often no different from those seen in the respective control groups.
We hypothesized that the nature of the control group employed in the different trials would have a significant impact on the relative risk of AEs. The controls used in the trials varied from 0.9% saline placebo, to aluminum or another adjuvant, through to a (non-COVID-19) active vaccine. As each of these ‘controls’ will have a different intrinsic AE profile, describing the relative risk of AEs of a specific COVID-19 vaccine will vary according to the control group chosen, as demonstrated in our analysis of the ChAdOx-1 and CoronaVac vaccines by control type. The choice of a control group may be influenced by different considerations; for example, a control group that is likely to be associated with few AEs (such as 0.9% saline) might allow a ‘blinded’ trial participant to work out whether they have in fact received the COVID-19 vaccine, and thus change their behavior, with a potential impact on trial integrity. However, use of such an inert placebo will allow a full description of the AEs associated with the COVID-19 vaccine being tested. This analysis may be too simplistic however, as it ignores the ‘nocebo’ effect. Nocebo responses are thought to be caused by misattribution of routine background symptoms, anxiety, and expectations of AEs. In their recent systematic review, Haas et al. [Citation63] focused only on the frequencies of AEs reported in the placebo groups of COVID-19 vaccine trials by excluding studies using a non-inert placebo. They estimated that 76% of systemic AEs and 24% of local AEs after the first vaccination were attributed to nocebo responses and 52% of systemic AEs and 16% of local AEs after the second dose.
Another cause for heterogeneity was extremes of age. Other reviews have drawn similar conclusions, observing that the overall adverse event incidence is higher for vaccinees aged 16–55 years than among older adults aged over 55 years [Citation7,Citation8].
The information provided in this review is important for health-care workers, policy makers and the general public when making decisions around receipt of COVID-19 vaccines. It may also allow better matching of specific vaccines with specific populations; for example, the preferred use of the least reactogenic vaccine platform in pregnant women. All of these considerations, however, are likely to be significantly offset by considerations around the efficacy of the respective vaccines, as an individual may prefer a more effective vaccine despite its greater reactogenicity. Clear differences in efficacy and effectiveness are now evident among the different vaccine types [Citation4,Citation5]. It does raise the possibility that heterologous vaccine schedules may allow matching of different vaccines with different reactogenicity and efficacy profiles in order to provide an overall schedule with lower reactogenicity and preserved efficacy. Such a hypothesis requires further study.
There are a few limitations to our study. We focused on the side effect profile of homologous primary dosing vaccine schedules and excluded studies evaluating booster doses or heterologous vaccine regimens, which are the focus of multiple on-going COVID-19 vaccine trials.
We were unable to fully evaluate several factors which may affect reactogenicity, for example age, ethnicity and prior COVID-19 infection, due to lack of granularity of the data. Some of the trials reported in this review are still on-going, therefore full safety results are not yet published or available for researchers to include in meta-analyses. Once larger datasets are available and additional trials on the pediatric population are published, meta regression would be useful to infer the effect of these variables.
Despite not limiting language in our search method, we only used English language databases and therefore will have missed publications in other languages, resulting in publication bias. In addition to this, there is an underrepresentation of trials from developing countries.
Lack of a standardized study design for the COVID-19 vaccine studies made comparing studies challenging. The three most common differences noted were: a variation in the number and type of symptoms participants were asked to report, the choice of control used, and whether data was reported by single dose or by combined dose.
We have demonstrated considerable variability in the number of local and systemic AEs that were solicited in the individual trials. Inviting participants to report a greater number of symptoms may result in the overall vaccine reactogenicity appearing to be more severe than studies that stipulate reporting of fewer symptoms. Reporting of a standard list of symptoms (as well as using standard definitions of events and of severity) would allow a more accurate and complete comparison of the reactogenicity profile of different vaccines. Such calls have been made previously by the Brighton Collaboration for vaccine studies in general [Citation64].
The majority of trials reported reactogenicity data for each of the vaccine doses given. Some combined the data and reported it for the whole vaccine course. As the data was most commonly presented as occurrence of each symptom per participant for the whole vaccine course, this may underestimate the reactogenicity, as the same participant could have experienced the symptom twice, once with each vaccine dose. This is reflected in our results, as there was no statistically significant difference in the occurrence of reactogenicity symptoms between the control and vaccine group for the studies with results presented in this way.
5. Conclusions
Among COVID-19 vaccines currently available and/or in Phase III trials, the four vaccine types (platforms) appear to have a distinct reactogenicity profiles, which also varies between the first and second dose of each individual vaccine. Both doses of mRNA vaccines, the second dose of protein subunit and first dose of adenovirus vectored vaccines were the most reactogenic, while the inactivated vaccines were the least reactogenic. Awareness of the reactogenicity profiles of different vaccine types can allow different vaccines to be recommended for specific populations. The lack of standardization of COVID-19 vaccine trials and the way data is reported made comparisons challenging. Greater standardization of this will aid research in the future.
6. Expert opinion
Current COVID-19 vaccine trials have shifted their original focus on safety and efficacy of doses in unvaccinated participants to booster studies (third or fourth doses), which commonly include heterologous ‘mix-match’ schedules combining more than one vaccine platform, rather than the homologous primary dosing schedules which are the subject of this review. As a result, there may be significant differences in the reactogenicity of booster dosing schedules. In addition to this, previous COVID-19 immunity may impact the range and intensity of side effects experienced after vaccination. Furthermore, novel studies are being performed on population groups previously excluded from the earlier trials: children, pregnant women and immunocompromised patients, who may have a different reactogenicity profile compared to the general public.
Side effect data reporting on vaccine trials are still very heterogeneous, despite international efforts to unify definitions such as through the Brighton collaboration. More efforts should be made to standardize COVID-19 vaccine trial design: from the choice of control, follow-up duration, definitions of solicited and unsolicited adverse events to safety data reporting and choice of denominator to calculate adverse event rates (participants receiving a vaccine versus participants reporting adverse events).
Ultimately, the choice of COVID-19 vaccine will need to consider multiple factors, including reactogenicity, frequency of rare and serious adverse events, efficacy against circulating COVID-19 variant strains, availability of doses and costs.
For obvious reasons, the development, testing, and implementation of COVID-19 vaccines have occurred at an unprecedented pace. This now means there are likely to be fewer opportunities to assess new COVID-19 vaccine candidates in the context of a placebo-controlled trial. Comparisons will need to be made against licensed COVID-19 vaccines, rather than against non-COVID vaccine control groups. This may complicate any ongoing analysis of the reactogenicity of COVID-19 vaccines and will require creative trial designs to ensure that the reactogenicity of new candidate vaccines can be accurately reported. In addition to this, safety monitoring will shift from RCTs to large community cohort longitudinal follow-up data or Phase IV pharmacovigilance studies to detect infrequent adverse events.
It is likely that studies of the reactogenicity of COVID-19 vaccines as booster doses, as part of heterologous schedules and in other important groups such as children will become more common over the next 5 years.
Article highlights
The scientific community’s response to the COVID-19 pandemic has resulted in the development of numerous COVID-19 vaccines in a short period of time.
Four main vaccine types (mRNA, adenovirus-vector, protein subunit and inactivated) are in advanced clinical trials or approved for general use in different populations.
Each vaccine type has a unique reactogenicity profile with mRNA vaccines being most reactogenic and inactivated vaccines being least reactogenic.
High heterogeneity is evident within each vaccine type. This reduced when age was restricted to adults aged 16–55 years and individual vaccines were analyzed separately.
Lack of standardization of COVID-19 vaccine trial design makes comparison of different vaccines challenging. The main differences noted were in variation in the number and type of post-vaccine symptoms participants were asked to report, the choice of control used and whether data was reported by single dose or combined doses.
Use of a control (adjuvants or MenACWY vaccine) instead of a placebo (0.9% saline) reduced the risk ratios of AEs, thereby underestimating reactogenicity.
Standardization of vaccine trial design and reporting will aid comparison of vaccines in the future.
Awareness of the reactogenicity profile of different vaccine types will aid health-care workers and policy makers to make decisions around the use of different vaccine types in different settings and populations. For example, the use of less reactogenic vaccines for pregnant women and children.
Declaration of interest
PT Heath coordinates research on behalf of St Georges, University of London which is funded by vaccine manufacturers, including those that manufacture COVID-19 vaccines (Pfizer, AZ, Novavax, Moderna, Valneva, Janssen). The authors have no other relevant affiliations or financial involvement with any organization or entity with a financial interest in or financial conflict with the subject matter or materials discussed in the manuscript apart from those disclosed.
Reviewer disclosures
Peer reviewers on this manuscript have no relevant financial or other relationships to disclose.
Author contributions
PH, NS, SI, DS, EG, and YH conceptualized and designed the work. NS, ASFR, and EB carried out the database search and identified eligible studies. NS, ASFR, EB, DS, SI, and YH carried out risk of bias assessments and data extraction. DS summarized raw data from authors using R. Funnel plots were created by ASFR. Data analysis and interpretation was guided by YH. PH, NS, ASFR, EB, and EG drafted the manuscript. All authors contributed to, reviewed, and approved the final manuscript.
Supplemental Material
Download Zip (50.3 MB)Acknowledgments
We thank Jonathan Lewis-Bridgeman and Atul Kumar-Beurg for their help with Excel and data management, and Caroline Albrecht for creating .
Supplementary material
Supplemental data for this article can be accessed online at https://doi.org/10.1080/14760584.2022.2098719
Additional information
Funding
References
- World Health Organisation. WHO coronavirus (COVID-19) dashboard [Internet]. [ cited 2022 May 6th]. Available from: https://covid19.who.int
- The Vaccine Centre at the London School of Hygiene and Tropical Medicine. Vaccine landscape [internet]. COVID-19 vaccine tracker; 2022 [cited 2022 May 6th]. Available from: https://vac-lshtm.shinyapps.io/ncov_vaccine_landscape
- VIPER Group COVID19 Vaccine Tracker Team. COVID19 vaccine tracker [Internet]. [ cited 2022 May 6th]. Available from: https://covid19.trackvaccines.org
- Ling Y, Zhong J, and Luo J. Safety and effectiveness of SARS-CoV-2 vaccines: a systematic review and meta-analysis. J Med Virol. 2021 Dec;93(12):6486–6495.
- Pormohammad A, Zarei M, Ghorbani S, et al. Efficacy and safety of COVID-19 vaccines: a systematic review and meta-analysis of randomized clinical trials. Vaccines (Basel) [Internet]. 2021 May 6;9(5). DOI:10.3390/vaccines9050467.
- Yuan P, Ai P, Liu Y, et al. Safety, tolerability, and immunogenicity of COVID-19 vaccines: a systematic review and meta-analysis. medRxiv [Internet]. 2020 Nov 4. DOI:10.1101/2020.11.03.20224998.
- Chen M, Yuan Y, Zhou Y, et al. Safety of SARS-CoV-2 vaccines: a systematic review and meta-analysis of randomized controlled trials. Infect Dis Poverty. 2021 Jul 5;10(1):94.
- Wang J, Tong Y, and Li D, et al. The impact of age difference on the efficacy and safety of COVID-19 vaccines: a systematic review and meta-analysis. Front Immunol. 2021 Dec 6;12:758294.
- Glanville J, Kotas E, Featherstone R, et al. Which are the most sensitive search filters to identify randomized controlled trials in medline? J Med Libr Assoc. 2020 Oct 1;108(4):556–563.
- Harrison H, Griffin SJ, Kuhn I, et al. Software tools to support title and abstract screening for systematic reviews in healthcare: an evaluation. BMC Med Res Methodol. 2020 Jan 13;20(1):7.
- Shrotri M, Swinnen T, Kampmann B, et al. An interactive website tracking COVID-19 vaccine development. Lancet Glob Health. 2021 May;9(5):e590–2.
- Sterne JAC, Savović J, Page MJ, et al. RoB 2: a revised tool for assessing risk of bias in randomised trials. BMJ. 2019 Aug 28;366:l4898.
- Rohatgi A. WebPlotDigitizer [Internet]. Available from: https://automeris.io/WebPlotDigitizer
- Higgins JPT, Thompson SG, Deeks JJ, et al. Measuring inconsistency in meta-analyses. BMJ. 2003 Sep 6;327(7414):557–560.
- Sadoff J, Le Gars M, Shukarev G, et al. Interim results of a phase 1-2a trial of Ad26.COV2.S Covid-19 vaccine. N Engl J Med. 2021 May 13;384(19):1824–1835.
- Falsey AR, Sobieszczyk ME, and Hirsch I, et al. Phase 3 safety and efficacy of AZD1222 (ChAdOx1 nCoV-19) Covid-19 vaccine. N Engl J Med. 2021 Dec 16;385(25):2348–2360.
- Keech C, Albert G, Cho I, et al. Phase 1-2 trial of a SARS-CoV-2 recombinant spike protein nanoparticle vaccine. N Engl J Med. 2020 Dec 10;383(24):2320–2332.
- Richmond P, Hatchuel L, Dong M, et al. Safety and immunogenicity of S-Trimer (SCB-2019), a protein subunit vaccine candidate for COVID-19 in healthy adults: a phase 1, randomised, double-blind, placebo-controlled trial. Lancet. 2021 Feb 20;397(10275):682–694.
- Meng F-Y, Gao F, Jia S-Y, et al. Safety and immunogenicity of a recombinant COVID-19 vaccine (Sf9 cells) in healthy population aged 18 years or older: two single-center, randomised, double-blind, placebo-controlled, phase 1 and phase 2 trials. Signal Transduct Target Ther. 2021 Jul 15;6(1):271.
- Pu J, Yu Q, Yin Z, et al. The safety and immunogenicity of an inactivated SARS-CoV-2 vaccine in Chinese adults aged 18-59 years: a phase I randomized, double-blinded, controlled trial. Vaccine. 2021 May 12;39(20):2746–2754.
- Logunov DY, Dolzhikova IV, Shcheblyakov DV, et al. Safety and efficacy of an rAd26 and rAd5 vector-based heterologous prime-boost COVID-19 vaccine: an interim analysis of a randomised controlled phase 3 trial in Russia. Lancet. 2021 Feb 20;397(10275):671–681.
- Che Y, Liu X, Pu Y, et al. Randomized, double-blinded, placebo-controlled phase 2 trial of an inactivated severe acute respiratory syndrome coronavirus 2 vaccine in healthy adults. Clin Infect Dis. 2021 Dec 6;73(11):e3949–55.
- Zakarya K, Kutumbetov L, Orynbayev M, et al. Safety and immunogenicity of a QazCovid-in® inactivated whole-virion vaccine against COVID-19 in healthy adults: a single-centre, randomised, single-blind, placebo-controlled phase 1 and an open-label phase 2 clinical trials with a 6 months follow-up in Kazakhstan. E Clin Med. 2021;39:101078.
- Ryzhikov AB, Еа R, Bogryantseva MP, et al. A single blind, placebo-controlled randomized study of the safety, reactogenicity and immunogenicity of the “EpiVacCorona” vaccine for the prevention of COVID-19, in volunteers aged 18–60 years (phase I–II). Infektsiia Immun. 2021 Mar 24;11(2):283–296.
- Thomas SJ, Moreira ED Jr, and Kitchin N, et al. Safety and efficacy of the BNT162b2 mRNA Covid-19 vaccine through 6 months. N Engl J Med. 2021 Nov 4;385(19):1761–1773.
- Polack FP, Thomas SJ, Kitchin N, et al. Safety and efficacy of the BNT162b2 mRNA Covid-19 vaccine. N Engl J Med. 2020 Dec 31;383(27):2603–2615.
- Sadoff J, Gray G, and Vandebosch A, et al. Safety and efficacy of single-dose Ad26.COV2.S vaccine against Covid-19. N Engl J Med. 2021 Jun 10;384(23):2187–2201.
- Al Kaabi N, Zhang Y, Xia S, et al. Effect of 2 inactivated SARS-CoV-2 vaccines on symptomatic COVID-19 infection in adults: a randomized clinical trial. JAMA. 2021 Jul 6;326(1):35–45.
- Zhu F, Jin P, and Zhu T, et al. Safety and immunogenicity of a recombinant adenovirus type-5–vectored coronavirus disease 2019 (COVID-19) vaccine with a homologous prime-boost regimen in healthy participants aged ≥6 years: a randomized, double-blind, placebo-controlled, phase 2b trial. Clin Infect Dis [Internet]. 2021 Sep 22:ciab845.
- Zhu F-C, Guan X-H, Li Y-H, et al. Immunogenicity and safety of a recombinant adenovirus type-5-vectored COVID-19 vaccine in healthy adults aged 18 years or older: a randomised, double-blind, placebo-controlled, phase 2 trial. Lancet. 2020 Aug 15;396(10249):479–488.
- Halperin SA, Ye L, MacKinnon-Cameron D, et al. Final efficacy analysis, interim safety analysis, and immunogenicity of a single dose of recombinant novel coronavirus vaccine (adenovirus type 5 vector) in adults 18 years and older: an international, multicentre, randomised, double-blinded, placebo-controlled phase 3 trial. Lancet. 2022 Jan 15;399(10321):237–248.
- Folegatti PM, Ewer KJ, Aley PK, et al. Safety and immunogenicity of the ChAdOx1 nCoV-19 vaccine against SARS-CoV-2: a preliminary report of a phase 1/2, single-blind, randomised controlled trial. Lancet. 2020 Aug 15;396(10249):467–478.
- Madhi SA, Baillie V, Cutland CL, et al. Efficacy of the ChAdOx1 nCoV-19 Covid-19 vaccine against the B.1.351 variant. N Engl J Med. 2021 May 20;384(20):1885–1898.
- Madhi SA, Koen AL, Izu A, et al. Safety and immunogenicity of the ChAdOx1 nCoV-19 (AZD1222) vaccine against SARS-CoV-2 in people living with and without HIV in South Africa: an interim analysis of a randomised, double-blind, placebo-controlled, phase 1B/2A trial. Lancet HIV. 2021 Sep;8(9):e568–80.
- Ramasamy MN, Minassian AM, Ewer KJ, et al. Safety and immunogenicity of ChAdOx1 nCoV-19 vaccine administered in a prime-boost regimen in young and old adults (COV002): a single-blind, randomised, controlled, phase 2/3 trial. Lancet. 2021 Dec 19;396(10267):1979–1993.
- Asano M, Okada H, Itoh Y, et al. Immunogenicity and safety of AZD1222 (ChAdOx1 nCoV-19) against SARS-CoV-2 in Japan: a double-blind, randomized controlled phase 1/2 trial. Int J Infect Dis. 2022 Jan;114:165–174.
- Kremsner PG, Ahuad Guerrero RA, Arana-Arri E, et al. Efficacy and safety of the CVnCoV SARS-CoV-2 mRNA vaccine candidate in ten countries in Europe and Latin America (HERALD): a randomised, observer-blinded, placebo-controlled, phase 2b/3 trial. Lancet Infect Dis. 2022 Mar;22(3):329–340.
- Frenck RW Jr, Klein NP, Kitchin N, et al. Safety, immunogenicity, and efficacy of the BNT162b2 Covid-19 vaccine in adolescents. N Engl J Med. 2021 Jul 15;385(3):239–250.
- Walsh EE, Frenck RW Jr, Falsey AR, et al. Safety and immunogenicity of Two RNA-Based Covid-19 vaccine candidates. N Engl J Med. 2020 Dec 17;383(25):2439–2450.
- Haranaka M, Baber J, Ogama Y, et al. A randomized study to evaluate safety and immunogenicity of the BNT162b2 COVID-19 vaccine in healthy Japanese adults. Nat Commun. 2021 Dec 14;12(1):7105.
- Ali K, Berman G, Zhou H, et al. Evaluation of mRNA-1273 SARS-CoV-2 vaccine in adolescents. N Engl J Med. 2021 Dec 9;385(24):2241–2251.
- Chu L, McPhee R, Huang W, et al. A preliminary report of a randomized controlled phase 2 trial of the safety and immunogenicity of mRNA-1273 SARS-CoV-2 vaccine. Vaccine. 2021 May 12;39(20):2791–2799.
- El Sahly HM, Baden LR, and Essink B, et al. Efficacy of the mRNA-1273 SARS-CoV-2 vaccine at completion of blinded phase. N Engl J Med. 2021 Nov 4;385(19):1774–1785.
- Xia S, Zhang Y, Wang Y, et al. Safety and immunogenicity of an inactivated COVID-19 vaccine, BBIBP-CorV, in people younger than 18 years: a randomised, double-blind, controlled, phase 1/2 trial. Lancet Infect Dis. 2022 Feb;22(2):196–208.
- Wu Z, Hu Y, Xu M, et al. Safety, tolerability, and immunogenicity of an inactivated SARS-CoV-2 vaccine (CoronaVac) in healthy adults aged 60 years and older: a randomised, double-blind, placebo-controlled, phase 1/2 clinical trial. Lancet Infect Dis. 2021 Jun;21(6):803–812.
- Zhang Y, Zeng G, Pan H, et al. Safety, tolerability, and immunogenicity of an inactivated SARS-CoV-2 vaccine in healthy adults aged 18–59 years: a randomised, double-blind, placebo-controlled, phase 1/2 clinical trial. Lancet Infect Dis. 2021 Feb 1;21(2):181–192.
- Fadlyana E, Rusmil K, Tarigan R, et al. A phase III, observer-blind, randomized, placebo-controlled study of the efficacy, safety, and immunogenicity of SARS-CoV-2 inactivated vaccine in healthy adults aged 18-59 years: an interim analysis in Indonesia. Vaccine. 2021 Oct 22;39(44):6520–6528.
- Bueno SM, Abarca K, and González PA, et al. Safety and Immunogenicity of an Inactivated SARS-CoV-2 vaccine in a subgroup of healthy adults in Chile. Clin Infect Dis [Internet]. 2021 Sep 19:ciab823.
- Ella R, Vadrevu KM, Jogdand H, et al. Safety and immunogenicity of an inactivated SARS-CoV-2 vaccine, BBV152: a double-blind, randomised, phase 1 trial. Lancet Infect Dis. 2021 May;21(5):637–646.
- Ella R, Reddy S, Blackwelder W, et al. Efficacy, safety, and lot-to-lot immunogenicity of an inactivated SARS-CoV-2 vaccine (BBV152): interim results of a randomised, double-blind, controlled, phase 3 trial. Lancet. 2021 Dec 11;398(10317):2173–2184.
- Heath PT, Galiza EP, Baxter DN, et al. Safety and efficacy of NVX-CoV2373 Covid-19 vaccine. N Engl J Med. 2021 Sep 23;385(13):1172–1183.
- Shinde V, Bhikha S, Hoosain Z, et al. Efficacy of NVX-CoV2373 Covid-19 vaccine against the B.1.351 variant. N Engl J Med. 2021 May 20;384(20):1899–1909.
- Formica N, Mallory R, Albert G, et al. Different dose regimens of a SARS-CoV-2 recombinant spike protein vaccine (NVX-CoV2373) in younger and older adults: a phase 2 randomized placebo-controlled trial. PLoS Med. 2021 Oct;18(10):e1003769.
- Dunkle LM, Kotloff KL, and Gay CL, et al. Efficacy and safety of NVX-CoV2373 in adults in the United States and Mexico. N Engl J Med. 2022 Feb 10;386(6):531–543.
- Hsieh S-M, Liu M-C, Chen Y-H, et al. Safety and immunogenicity of CpG 1018 and aluminium hydroxide-adjuvanted SARS-CoV-2 S-2P protein vaccine MVC-COV1901: interim results of a large-scale, double-blind, randomised, placebo-controlled phase 2 trial in Taiwan. Lancet Respir Med. 2021 Dec;9(12):1396–1406.
- Xia S, Zhang Y, Wang Y, et al. Safety and immunogenicity of an inactivated SARS-CoV-2 vaccine, BBIBP-CorV: a randomised, double-blind, placebo-controlled, phase 1/2 trial. Lancet Infect Dis. 2021 Jan;21(1):39–51.
- Tanriover MD, Doğanay HL, and Akova M, et al. Efficacy and safety of an inactivated whole-virion SARS-CoV-2 vaccine (CoronaVac): interim results of a double-blind, randomised, placebo-controlled, phase 3 trial in Turkey. Lancet. 2021 Jul 17;398(10296):213–222.
- Guo W, Duan K, Zhang Y, et al. Safety and immunogenicity of an inactivated SARS-CoV-2 vaccine in healthy adults aged 18 years or older: a randomized, double-blind, placebo-controlled, phase 1/2 trial. EClinicalMedicine. 2021 Aug;38:101010.
- Yang S, Li Y, Dai L, et al. Safety and immunogenicity of a recombinant tandem-repeat dimeric RBD-based protein subunit vaccine (ZF2001) against COVID-19 in adults: two randomised, double-blind, placebo-controlled, phase 1 and 2 trials. Lancet Infect Dis. 2021 Aug;21(8):1107–1119.
- Zhang J, Hu Z, He J, et al. Safety and immunogenicity of a recombinant interferon-armed RBD dimer vaccine (V-01) for COVID-19 in healthy adults: a randomized, double-blind, placebo-controlled, Phase I trial. Emerg Microbes Infect. 2021 Dec;10(1):1589–1597.
- Shu Y-J, He J-F, Pei R-J, et al. Immunogenicity and safety of a recombinant fusion protein vaccine (V-01) against coronavirus disease 2019 in healthy adults: a randomized, double-blind, placebo-controlled, phase II trial. Chin Med J. 2021 Jul 22;134(16):1967–1976.
- Han B, Song Y, Li C, et al. Safety, tolerability, and immunogenicity of an inactivated SARS-CoV-2 vaccine (CoronaVac) in healthy children and adolescents: a double-blind, randomised, controlled, phase 1/2 clinical trial. Lancet Infect Dis. 2021 Dec;21(12):1645–1653.
- Haas JW, Bender FL, Ballou S, et al. Frequency of adverse events in the placebo arms of COVID-19 vaccine trials: a systematic review and meta-analysis. JAMA Network Open. 2022 Jan 4;5(1):e2143955.
- Bonhoeffer J, Kohl K, Chen R, et al. The Brighton Collaboration: addressing the need for standardized case definitions of adverse events following immunization (AEFI). Vaccine. 2002 Dec 13;21(3–4):298–302.