ABSTRACT
Introduction
The escalating global changes have fostered conditions for the expansion and transmission of diverse biological factors, leading to the rise of emerging and reemerging infectious diseases. Complex viral infections, such as COVID-19, influenza, HIV, and Ebola, continue to surface, necessitating the development of effective vaccine technologies.
Areas covered
This review article highlights recent advancements in molecular biology, virology, and genomics that have propelled the design and development of innovative molecular tools. These tools have promoted new vaccine research platforms and directly improved vaccine efficacy. The review summarizes the cutting-edge molecular engineering tools used in creating novel vaccines and explores the rapidly expanding molecular tools landscape and potential directions for future vaccine development.
Expert opinion
The strategic application of advanced molecular engineering tools can address conventional vaccine limitations, enhance the overall efficacy of vaccine products, promote diversification in vaccine platforms, and form the foundation for future vaccine development. Prioritizing safety considerations of these novel molecular tools during vaccine development is crucial.
1. Introduction
Infectious pathogens present a considerable threat to global health, and their prevention and control remain a primary focus of the global public health system [Citation1]. Various strategies, such as monitoring, social distancing, and vaccination, are employed to mitigate and control infectious diseases [Citation2,Citation3]. Among these approaches, vaccination is the most cost-effective and efficient method for preventing and treating infectious diseases [Citation4]. Large-scale vaccination campaigns have successfully eradicated the highly virulent smallpox virus throughout history [Citation5]. Moreover, vaccination has significantly decreased the incidence and mortality rates of numerous severe infectious diseases, including polio [Citation6], rotavirus enteritis [Citation7], hepatitis B [Citation8], diphtheria, tetanus, pertussis [Citation9], cervical cancer, mumps [Citation10], and others. According to the World Health Organization (WHO) report, the widespread use of vaccines can save between 3.5 and 5 million lives annually. Despite varying acceptance and accessibility of vaccines across different nations and regions, their impact on public health is undeniable [Citation11]. However, as human society rapidly develops, the complexity of viruses we face continues to increase [Citation12]. The unpredictable COVID-19 pandemic demonstrates that emerging high-risk and highly variable viruses still pose substantial threats and present significant global health challenges [Citation13]. Furthermore, effective protective vaccines for pathogens such as human immunodeficiency virus (HIV) and Zika virus are still lacking [Citation14,Citation15]. Overcoming these challenges necessitates further advancements in vaccine research and development.
Vaccine research has been revolutionized by breakthroughs in virology, immunology, molecular biology, and other related disciplines [Citation16,Citation17]. These advancements have resulted in the creation of various molecular engineering tools, including gene editing tools [Citation18], protein modification tools [Citation19], molecular adjuvants [Citation20], viral vectors [Citation21], and molecular delivery tools [Citation22]. These tools have facilitated the development of novel vaccine technology platforms, the enhancement and improvement of existing vaccine products, and the increased safety and efficacy of vaccine formulations [Citation23,Citation24]. Moreover, molecular engineering tools have allowed for rapid responses to emerging high-risk pathogens, thus preventing and controlling large-scale epidemic events.
This review aims to provide a comprehensive overview of the current research and application status of molecular engineering tools, focusing on how these tools have contributed to the improvement of various vaccine technology platforms. Additionally, this review will discuss potential future research directions for vaccine technology. We believe this review will engage a broad range of readers and inspire further research and application of molecular engineering tools in vaccine development.
2. Genetic engineering tools
2.1. Cre-LoxP
Cre-LoxP is a site-specific DNA recombination system extensively employed in genetic engineering and research. The system comprises two components: the Cre recombinase enzyme and LoxP sites, which are specific DNA sequences that serve as recognition sites for Cre. Upon binding to LoxP sites, Cre catalyzes a recombination event( [Citation25,Citation26]. Molecular engineering tools, such as Cre-LoxP, can precisely modify the viral genome through deletion, insertion, or replacement, efficiently constructing attenuated vaccine strains.
Figure 1. The diagram illustrates the working principles of several genetic engineering tools used in vaccine development. (a) Cre recombinase specifically recognizes LoxP sites to achieve site-specific gene recombination, allowing for the creation of conditional knockouts or gene expression modulation. (b) the λ Red system comprises three proteins, including Exo, Beta, and Gam, and functions to promote homologous recombination for gene editing. (c) CRISPR-Cas9 genome editing utilizes purified Cas9 and sgRnas to target and modify specific genomic sequences. (d) Genetic code expansion is a tool that employs specific aaRS/tRNA pairs to introduce unnatural amino acids into the genetic code, which can be used for antigenic modification or virus attenuation. (e) Codon deoptimization strategies utilize suboptimal codons to reduce protein translation efficiency, resulting in effective virus attenuation. These genetic engineering tools offer a range of possibilities for modifying genetic sequences, from small-scale modifications to large-scale genome editing, and hold great potential for vaccine applications. The schematic illustration was drawn using Figdraw tools (www.figdraw.com).
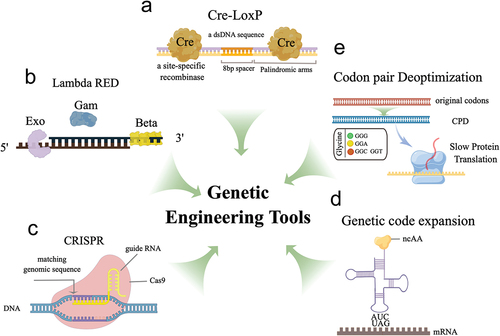
The development of replication-competent live attenuated vaccines (LAVs), consisting of weakened live viruses, has been closely examined since their inception [Citation27]. Due to the slow replication property of LAVs, they can mimic naturally occurring latent infections and induce an ideal immune response without causing clinical symptoms [Citation28]. Traditional virus attenuation methods depend on artificial mutagenesis or serial passaging, which face limitations due to safety and attenuation efficiency concerns [Citation29,Citation30]. In contrast, reverse genetics-based gene editing technology offers a more rational approach to virus attenuation [Citation31]. Numerous studies have confirmed the effectiveness of these attenuation strategies [Citation32,Citation33].
Wang et al. rationally developed the first VZV skin and neuro-attenuated live vaccine candidate for varicella, an ORF7-deficient vaccine based on the reverse genetics method using Cre-LoxP-mediated recombination tools, referred to as v7D [Citation34]. v7D exhibits immunogenicity both in vitro and in multiple small animal species, is well-tolerated, and retains the potency to elicit a protective immune response in children [Citation35]. The vaccine has advanced to a Phase I clinical trial in China (ChiCTR1900022284). Utilizing the Cre/Lox tool, Liang and colleagues introduced a novel vaccine development strategy to combat the reemerging pseudorabies virus (PRV). This system enables the precise excision of the marker gene on the viral strain, accelerating PRV vaccine development and ensuring the vaccine’s safety. The effectiveness of the candidate PRV vaccine was confirmed through vaccination and virus challenge experiments [Citation36]. These studies demonstrate the value of Cre/LoxP as a valuable research tool for rapid antiviral vaccine development in the future. Although Cre/LoxP has been widely used in genetic research, it has some limitations that can affect its precision and accuracy () [Citation37]. Some pseudo-LoxP sites have been identified, which possess sufficient identity to allow Cre recombinase binding and subsequent recombination at unintended sites [Citation38]. The extent of the problem may vary considerably from study to study but should still be carefully considered.
Table 1. Summary of molecular engineering tools.
2.2. Lambda red recombinase
The Lambda Red recombination system is a powerful genetic recombination system found in bacteriophage λ, consisting of three proteins: Exo, Beta, and Gam. Exo is a 5’−3“ exonuclease that degrades single-stranded DNA (ssDNA) in a 5”−3’ direction, generating 3’ overhangs. Beta is a single-strand annealing protein that binds to the ssDNA overhangs produced by Exo and facilitates the annealing of complementary sequences [Citation39]. Together, these proteins enable homologous recombination between two DNA molecules, rendering the Red recombination system a valuable tool for genetic manipulation, including gene knockouts, insertions, and modifications (). One common application of the Red system is in creating ‘knockout’ strains of bacteria, in which a specific gene is removed from the genome [Citation40].
In producing vaccine antigens, the Escherichia coli system offers unique advantages due to various growth-related characteristics [Citation41]. However, endotoxin contamination and plasmid instability pose two significant limitations [Citation42,Citation43]. Wang et al. utilized the Red system to eliminate the genes related to endotoxin synthesis in E. coli and introduced the human papillomavirus (HPV) L1 protein into the expression frame of endotoxin synthesis-related genes, constructing nine constitutive expression strains [Citation44]. These constitutive expression strains significantly improved L1 protein expression efficiency and effectively reduced endotoxin contamination, providing a novel strategy for constructing recombinant E. coli strains and developing HPV vaccines. However, concerns have arisen due to the reliance on the Lambda Red recombination system in the recombination process, as it depends on multiple proteases and the recombination efficiency levels observed have been inconsistent. Although this system has demonstrated some success in engineering E. coli strains, an understanding of the Red system’s underlying mechanism still remains elusive, presenting a significant challenge in optimizing recombination efficiency [Citation45].
2.3. CRISPR-Cas9
The CRISPR-Cas9 system is an adaptive immune system initially identified in prokaryotes [Citation46]. The system consists of the Cas9 nuclease and single guide RNA (sgRNA), which form the ribonucleoprotein (RNP) complex, serving as the primary functional unit [Citation47]. The Cas9 protein can efficiently cleave the target sequence with precise guidance from sgRNA, allowing for accurate deletion, insertion, and modification (). Due to its high efficiency and accuracy in gene editing, this system has become a powerful tool for genetic manipulation. It is currently extensively used in modifying various animal and plant cells and viruses and is undergoing preclinical research for correcting human genetic diseases [Citation48,Citation49].
The CRISPR/Cas9 system is a highly efficient and accurate tool for modifying viral genomes, making it a valuable resource in vaccine research. This system enables precise and efficient editing of the genomes of various DNA viruses, such as herpes simplex virus type I [Citation50], adenovirus [Citation51], pseudorabies virus [Citation52], and Epstein-Barr virus [Citation53]. By accurately deleting viral virulence genes, the CRISPR/Cas9 system can ensure the safety of viral vaccines. Additionally, genetically edited cell lines can meet the need for rapid viral amplification, making them critical factors in the development of viral vaccines [Citation54].
Several studies have highlighted the potential of CRISPR/Cas9 in accelerating the development of viral vaccines. For instance, Gowripalan et al. used CRISPR to inhibit virus replication in culture, enabling efficient screening of conventionally generated recombinant vaccinia viruses within two weeks [Citation55]. Borca et al. used the CRISPR/Cas9 system to efficiently develop a recombinant African swine fever virus by deleting the nonessential gene 8-DR from the highly virulent wild strain Georgia07 genome. This technique made the study of recombinant ASFV more convenient and effective [Citation32]. Zhang and colleagues developed a new generation of label-free L. major strain (LmCen-/-) attenuated strains as vaccines using CRISPR/Cas9 tools, which showed good safety in preclinical studies and produced protection and immune responses equivalent to those of Leishmania [Citation56]. Additionally, Wu et al. identified key genes that affect the replication of rotavirus and used CRISPR/Cas9 to construct a NEU2 gene knockout Vero cell, which significantly increased the virus titers [Citation57]. Multiple single or double knockouts of ZNF205, EP300, and CNTD2 genes in Vero cells also significantly increased the virus titers of poliovirus and enterovirus 71 [Citation58]. Therefore, regulating host genes through the CRISPR/Cas9 system can effectively improve the production of virus vaccines.
Despite its remarkable effectiveness and applicability, the CRISPR/Cas9 system still faces limitations such as potential off-target effects, efficiency factors, and delivery methods [Citation59]. An effective approach to preventing off-target effects includes the proper selection of the targeting site and the rational design of sgRNA [Citation60]. Furthermore, the CRISPR/Cas9 system shows a preference for specific base recognition, resulting in varied gene editing efficiencies across different targeted gene loci [Citation61]. Innovative targeted integration strategies, such as homology-independent targeted integration (HITI), have the potential to enhance CRISPR/Cas9 editing efficiency [Citation62]. However, efficient delivery of CRISPR/Cas9 remains a significant challenge in clinical applications [Citation63]. Combining other tools to complement its shortcomings may further expand the applicability of the CRISPR system and accelerate the research and production of various viral vaccines [Citation40,Citation64].
2.4. Genetic code expansion
Genetic code expansion (GCE) is a groundbreaking method for expanding the genetic code, developed by Wang and Schultz [Citation65]. It involves the introduction of modified translation machinery into cells to enable the encoding of non-natural amino acids in genes [Citation66]. The key component of this method is the orthogonal translation system (OTS), which is composed of aaRS/tRNA pairs that facilitate the recognition of non-natural amino acids. With the aid of OTS, tRNA can carry non-natural amino acids to recognize nonsense codons, typically amber codons, and incorporate them into the protein synthesis process, thereby enabling the introduction of non-natural amino acids () [Citation67]. Currently, this technique is mainly used in the fields of protein imaging, protein function research, and vaccine development [Citation68].
The OTS-based GCE tool relies on genetically modified cell lines and cannot function in conventional mammalian cells, making it a valuable attenuated virus construction tool. By introducing amber codons into the viral genome in a controlled manner, the virus can depend on GCE for normal proliferation in genetically modified cell lines. Progeny viruses generated in this way maintain complete infectivity but cannot replicate in normal mammalian cells, making it an ideal attenuated vaccine construction approach [Citation69]. Si et al. created a type A influenza virus carrying a premature termination codon (PTC) that can generate proliferative and genetically stable progeny viruses in transgenic cell lines containing an orthogonal translation mechanism. These PTC viruses are completely safe in conventional cells, and in mouse, ferret, and guinea pig models, PTC virus inoculation stimulated robust humoral, mucosal, and T cell-mediated immunity against antigenically diverse influenza viruses [Citation70]. Wang et al. presented a novel application of genetic code expansion. They achieved virus attenuation by introducing unnatural amino acid-encoding codons into the HIV-1 genome, successfully demonstrating that HIV-1 replication can be precisely controlled in vitro [Citation71]. A similar attenuation principle also holds promise for designing safe and effective vaccines against the Zika and RSV viruses [Citation72,Citation73]. The strategy of constructing attenuated strains through genetic code expansion (GCE) has broad applicability, particularly for addressing virulent strains that are difficult to attenuate using conventional methods. However, the safety of the PTC virus remains a concern, primarily due to the potential for mutation in its termination codon, particularly in virus strains known for their propensity to mutate. Such a mutation could restore the virus’s functional replication capabilities [Citation74]. Additionally, the selection of PTC sites and the types of non-natural amino acids pose significant challenges, as these factors are closely linked to both the viral genome and viral particle assembly [Citation75]. Furthermore, the safety of PTC viruses carrying non-natural amino acids in vivo must be rigorously evaluated.
2.5. Codon pair deoptimization
Codon pair deoptimization (CPD) is a highly effective and widely applicable viral attenuation strategy that limits virus replication by increasing the frequency of suboptimal codons through synonymous substitutions in one or more open reading frames of the pathogen [Citation76]. The substitution of synonymous codons does not alter the amino acid composition, allowing the CPD-modified pathogen to induce immune responses similar to those elicited by the wild-type pathogen () [Citation77]. Groenke and colleagues have demonstrated that CPD reduces mRNA stability, resulting in the inhibition or interruption of viral protein translation as the primary mechanism of CPD [Citation78]. Recently, CPD has been successfully employed to attenuate several viruses, including poliovirus [Citation79,Citation80], influenza A virus [Citation81], respiratory syncytial virus [Citation82], and type 1 HIV [Citation83]. For example, Cyril Le Nouën and colleagues designed a new attenuated RSV vaccine using CPD and showed that the CPD strain exhibited lower growth efficiency in vitro and growth restriction in mice and African green monkeys, indicating that CPD of non-segmented negative-strand RNA viruses can rapidly produce candidate vaccines with a certain degree of attenuation [Citation82].
Using a similar attenuation strategy, Li et al. designed a new CPD Zika vaccine. They demonstrated that the Min E+NS1 CPD mutant significantly reduced replication in Vero cells, had lower virulence in a mouse model, and induced a strong neutralizing antibody titer to combat lethal challenge [Citation84]. However, vaccine development strategies that rely on CPD necessitate a thorough assessment of the degree of virus weakening to achieve a delicate balance between attenuation and immunogenicity, a frequently labor-intensive procedure [Citation85]. Therefore, it is crucial to balance the reduction in translation efficiency with the maintenance of sufficient levels of immunogenicity to ensure the effectiveness of this strategy in developing vaccines or other applications.
3. Protein engineering tools
3.1. Covalent Isopeptide interactions: SpyTag/SpyCatcher system
The SpyTag/SpyCatcher system is a peptide-based bioconjugation technology derived from the CnaB2 domain of the FbaB protein in Streptococcus pyogenes. SpyTag, a 13-amino acid peptide, spontaneously forms an isopeptide bond with the 116-amino acid SpyCatcher domain under a wide range of conditions () [Citation86]. This covalent interaction addresses the limitations of traditional peptide tags that are unable to stably bind, making it a versatile and robust tool for protein structure extension and bioconjugation. With its ‘molecular glue’ characteristic, the SpyTag/SpyCatcher system has advanced research in enzymology, multivalent antibodies, and innovative vaccines [Citation87].
Figure 2. A schematic representation of the working principles of various protein engineering tools. (a) the SpyTag/SpyCatcher system utilizes intramolecular natural isopeptide bonds to enable covalent linkages between molecules. (b) Sortase a recognizes distinct motifs and mediates the covalent attachment of protein molecules. (c) PROTACs are a category of molecules that recruit E3 ubiquitin ligases to target proteins, resulting in their ubiquitination and subsequent degradation by the proteasome system. (d) I53–50 functions as a platform for developing multiple nanoparticle vaccines by displaying antigens on their surface. (e) Oligosaccharyltransferase possesses the capacity to covalently bind natural polysaccharides to specific sites on carrier proteins, streamlining the preparation of glycoconjugated vaccines. These protein engineering tools offer a spectrum of possibilities for modifying proteins, from small-scale modifications to extensive protein conjugation and vaccine production, holding substantial potential for future research and clinical applications. The schematic illustration was drawn using Figdraw tools (www.figdraw.com).
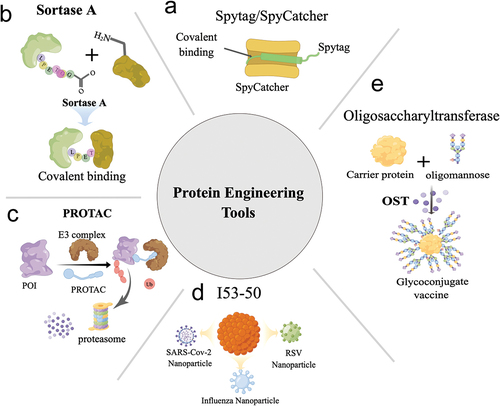
Ma et al. reported a novel strategy for developing a nanoparticle antigen using SpyCatcher. The authors fused the SpyCatcher domain to the N-terminus of a 24-subunit ferritin protein. Subsequently, a SpyTag was introduced to the receptor-binding domain (RBD) of the SARS-CoV-2 spike protein. The resulting covalent bond between the RBD and ferritin particles formed a 24-mer nanoparticle antigen. Moreover, the authors constructed a hybrid particle vaccine by incorporating the heptad repeat (HR) subunit of SARS-CoV-2 into the RBD particle. Remarkably, the nanoparticle vaccine generated through the SpyTag/SpyCatcher approach elicited stronger neutralizing antibody and cellular immune responses than the monomer vaccine. Furthermore, hACE2 transgenic mice immunized with the nanoparticle vaccine demonstrated resistance to SARS-CoV-2 challenge [Citation88].
In a related study, Cohen et al. employed the SpyTag/SpyCatcher system to create a broader-spectrum RBD nanoparticle vaccine. They fused SpyCatcher to the surface of a 60-mer Mi3 protein nanoparticle as a carrier, enabling the covalent display of SpyTag-labeled RBDs from four or eight distinct viral strains on the Mi3 particle surface (Mosaic-RBD). The neutralizing antibodies induced by Mosaic-RBD exhibited enhanced cross-reactivity with heterologous RBDs, highlighting its potential as a promising broad-spectrum vaccine candidate [Citation89].
The development of the SpyTag/SpyCatcher system has broadened the scope of vaccine antigen design and introduced a novel approach for constructing wide-spectrum immunogens. This system is expected to garner increased interest in vaccine design for variable pathogens such as influenza, SARS-CoV-2, and HIV. The primary limitation of the vaccine based on SpyTag/SpyCatcher technology pertains to its safety. While the vaccine has shown promising results in preclinical studies, its safety profile remains unverified in human clinical trials, warranting further research to thoroughly evaluate both its safety and efficacy [Citation90].
3.2. Sortase A
Sortase A, an enzyme superfamily widely distributed in Gram-positive bacteria, covalently attaches specific proteins to the bacterial cell wall [Citation91]. In Staphylococcus aureus, Sortase A recognizes the LPXTG motif, cleaving between serine and glycine residues to create an acylated intermediate. This intermediate is then released through nucleophilic attack of oligoglycine and covalently linked to the amino group of pentaglycine in the cell wall crossbridge, resulting in cell wall-anchored proteins () [Citation92]. As a useful tool in protein site-specific modification, Sortase A has gained popularity in protein labeling and bispecific antibody research. Its site-specific properties make it an effective immunogen modification tool and a popular research target in molecular biology [Citation93].
Tang et al. proposed a novel vaccine design strategy targeting the AD-4 domain of human cytomegalovirus (HCMV) glycoprotein gB. Using the hepatitis B virus core antigen (a virus-like particle, VLP) as a scaffold, they introduced the LPETGG motif on the VLP surface. This motif served as a substrate for Sortase A, a transpeptidase catalyzing the cleavage of the LPETGG motif at the serine and glycine junction, leading to an acylated intermediate with the LPRT motif. Sortase A then mediated the nucleophilic attack of GGGS-AD-4, resulting in the covalent linkage of the oligoglycine antigen GGGS-AD-4 to the VLP surface, forming a multivalent particle antigen. The researchers achieved over 90% coupling efficiency for two confirmed linear epitopes of EV71, GGG-SP55 and GGG-SP7021, using the same LPETGG-labeled HBc VLP and Sortase A strategy. The resulting epitope chimeric particle generated strong specific IgG antibodies and provided immune protection against lethal EV71 virus challenge [Citation94].
Recently, Sortase A has emerged as a crucial tool for particleization of the SARS-CoV-2 receptor-binding domain (RBD). The RBD protein can maintain its activity and proper orientation on ferritin particle surfaces, stimulating cross-neutralizing antibodies in macaques [Citation95]. Additionally, Sortase A offers a novel approach to developing subunit vaccines with self-adjuvant activity. Xu et al. demonstrated Sortase A’s potential by site-specifically attaching a fibroblast-stimulating lipopeptide-1 (a TLR2 agonist) to a recombinant antigen of group A streptococcus (GAS), leading to the design of a new vaccine with adjuvant effects. This vaccine, without additional adjuvants, elicited high-titer specific IgG antibodies in mice and conferred protection against subcutaneous challenge with GAS strains [Citation96].
Sortase A serves as a powerful tool for designing antigen-presenting cell (APC)-targeted vaccines. Duarte et al. utilized Sortase A to specifically link recombinant immunogens with APC-targeting nanobodies, maintaining the immunogenicity of the antigen and the targeting ability of the nanobody. This approach significantly improved cell-mediated immune response efficiency. The antigen construction strategy offers a modular design platform for APC-targeted vaccine design, enabling efficient coupling of various antigens with modular molecules [Citation97].
However, the widespread application of Sortase A has yet to be realized. The technique faces limitations, such as the widespread application of Sortase A has yet to be realized due to limitations such as the need for designing LPXTG motifs at appropriate sites, typically at the N- or C-terminus of the protein. The accessibility and flexibility of these terminal regions can significantly impact the efficiency of the reaction, thereby restricting the applicability of Sortase A. Additionally, reduced reaction rates due to the complexity of substrate binding and challenges in product yield presented by the LPXTG motif [Citation98]. Advancements in enzymatic kinetics and substrate specificity will be crucial to expanding the innovative applications of Sortase A tools across diverse fields.
3.3. PROTAC for vaccine development
Proteolysis targeting chimeras (PROTACs) are precise and controllable protein degradation systems that target proteins of interest (POIs) for degradation through the endogenous ubiquitin-proteasome system [Citation99]. These bifunctional molecules consist of a domain recognizing E3 ubiquitin ligases and a domain targeting the POI. Upon capture by PROTAC, the POI is recruited to E3 ubiquitin ligases, leading to its ubiquitination and subsequent proteasomal degradation () [Citation100]. Initially developed for chemical small molecule drugs against cancers, PROTAC technology has emerged as a powerful tool for vaccine development.
Si et al. designed a novel PROTAC virus vaccine by incorporating a protein-targeting domain (PTD) that includes a proteasome-targeting peptide and a tobacco etch virus protease (TEVp) recognition site. They introduced PTD into the influenza A virus genome and fused it with the M1 protein, allowing TEVp to specifically cleave M1-PTD and enable the normal assembly of virus particles. In the absence of TEVp, however, M1-PTD is degraded by the ubiquitin-proteasome system, rendering the virus incapable of self-replication in conventional cells. The PROTAC virus vaccine exhibited a high level of attenuation in mouse and ferret models while eliciting robust and broad immune responses against homologous and heterologous viruses [Citation101].
PROTAC has shown remarkable success in antiviral applications. Chatterjee et al. reported a novel approach to combat SARS-CoV-2 by designing a peptide specifically recognizing the receptor binding domain (RBD) and fusing it with the Fc domain of an antibody at its C-terminus. The Fc-fused peptide recognized intracellular RBD protein and recruited the E3 ubiquitin ligase TRIM21 via the Fc domain, leading to intracellular degradation of RBD [Citation102]. This study demonstrated the potent RBD degradation capabilities of the PROTAC molecule in human cells. Although PROTAC technology is relatively new, it holds significant potential in prophylactic and therapeutic vaccine research [Citation103]. To fully exploit this tool, researchers must focus on the specificity and high affinity between PROTAC and target molecules, as well as the potential impact of molecular design and chemical composition of PROTAC on degradation efficiency [Citation104–106]. Additionally, only a handful of E3 ligases (VHL, CRBN, cIAPs, and MDM2) have been used in the design of PROTACs, despite more than 600 E3 ubiquitin ligases having been identified in humans. Expanding the repertoire of these ligases poses a significant challenge in this field [Citation107].
3.4. I53–50 nanoparticles
Advancements in structural biology and bioinformatics have driven new breakthroughs in computational protein design [Citation108]. The I53–50 nanoparticle, a prime example of computational protein design, was first reported by Bale et al. in 2016. Its structure consists of 12 pentameric and 20 trimeric structural units arranged along the fivefold and threefold icosahedral symmetry axes () [Citation109]. The self-assembling I53–50 nanoparticle, designed through computational methods, has garnered significant interest from researchers and has impacted the direction of nanovaccine research. Its development offers a powerful approach for designing immunogens with broad protective capabilities against various infectious diseases.
Arunachalam and colleagues used the I53–50 scaffold protein to display the receptor-binding domain (RBD) of the SARS-CoV-2 spike protein on its surface and evaluated its effectiveness with four different adjuvants in a macaque model. The I53–50-based nanoparticle vaccine elicited a potent and durable neutralizing antibody response, protecting macaques from SARS-CoV-2 infection. These promising results have led to further clinical investigations (NCT04750343) [Citation110].
The controllable self-assembly properties of I53–50 nanoparticles offer a promising approach for designing immunogens with broad protective capabilities. In a recent study, Boyoglu-Barnum and colleagues fused four distinct hemagglutinin (HA) proteins from the influenza virus onto the trimeric structural units of I53–50 particles. Utilizing controllable self-assembly, these antigens with diverse immunogenic epitopes were assembled into chimeric forms, inducing broad-spectrum immune protection [Citation111]. Employing a similar construction strategy, RSV and HCV nanoparticle vaccines based on I53–50 demonstrated significant efficacy in vivo [Citation112,Citation113]. The impressive results of the I53–50 vector showcase its potential as a powerful tool for developing other viral vaccines. This tool is expected to provide better solutions to the challenges of weak immunogenicity and viral escape in the future. However, a primary limitation stems from the potential impact of displaying the target protein on the surface of I53–50, as it could disrupt the nanocarrier’s normal assembly. As such, the structural features of the target protein must align suitably with the nanocarrier to ensure efficient assembly.
3.5. Oligosaccharyltransferase in glycoconjugate vaccines
Glycoconjugate vaccines have demonstrated efficacy in reducing the incidence of severe bacterial infections. These vaccines are produced by covalently linking bacterial polysaccharides and carrier proteins using chemical conjugation techniques () [Citation114]. Currently, licensed examples of glycoconjugate vaccines are available for Haemophilus influenzae type b [Citation115], Neisseria meningitis [Citation116], and Streptococcus pneumoniae [Citation117]. Despite their proven safety and effectiveness, the production of glycoconjugate vaccines involves multiple steps and requires rigorous quality control. Additionally, the chemical conjugation process can be unpredictable, which limits the widespread application of these vaccines. Further research is needed to optimize the production process of glycoconjugate vaccines and develop alternative conjugation methods that can enhance their applicability [Citation118].
To address these limitations, Protein Glycan Coupling Technology (PGCT) was developed as a novel approach for producing glycoconjugate vaccines. PGCT utilizes an engineered oligosaccharyltransferase (OST) capable of covalently binding native polysaccharides to specific sites on the carrier protein, simplifying the traditional process of glycoconjugate vaccine production. This efficient, streamlined polysaccharide-protein preparation technique effectively expands the study of glycoconjugate vaccines [Citation119].
Studies by Reglinski et al. have demonstrated the effectiveness of PGCT in producing glycoconjugate vaccines. PGCT-generated glycoconjugate vaccines induced strong antibody responses to both capsule polysaccharides and carrier proteins in a mouse model, achieving effects similar to conventional vaccines [Citation120].
Some glycoconjugate vaccines based on PGCT production have entered clinical trials for review, including O-antigen conjugates of type I Shigella dysenteriae, Shigella flexneri 2a, and uropathogenic pathogenic E. coli [Citation121–123]. However, the efficient expression of capsule polysaccharides relies on specific bacterial strains due to their diversity and complexity. The coupling efficiency of complex polysaccharides with oligosaccharyltransferase is significantly influenced by their compatibility. Additionally, only one structure of OST (PglB) has been reported, and the relationship between its structure and glycosylation ability remains only partially understood [Citation124]. Factors such as the protein carrier, the site of glycan coupling, and polymer length directly impact vaccine efficacy. These concerns pose challenges to the development of PGCT-based vaccines, emphasizing the need for further research to address these limitations [Citation118].
4. Adjuvant engineering
Subunit vaccines offer a simplified approach to vaccine formulation and enhanced safety by utilizing the immunogenic protective components of pathogens as the primary source of antigens. This strategy helps to avoid potential toxicities, allergens, and self-immune-triggering components of pathogens [Citation125]. Recombinant subunit vaccines have achieved remarkable success in the development of vaccines for diseases such as SARS-CoV-2, varicella-zoster, and cervical cancer [Citation126–128]. However, the main challenge with subunit vaccines is their limited immunogenicity [Citation129]. Adjuvants are substances or molecules that are combined with antigens to stimulate a stronger immune response (), and nearly all approved subunit vaccines have been developed with the help of adjuvants [Citation130,Citation131].
Figure 3. The schematic diagram illustrates several widely used vaccine research tools in recent years. (a) the active ingredients of adjuvants and their approved use in humans are depicted. (b) Viral vectors serve as a popular tool for delivering antigens to the immune system, with various viral vectors developed and in use. (c) Lipid nanoparticles (LNPs) have emerged as the most commonly used tool for delivering mRNA-based vaccines. Additionally, the use of cell-penetrating peptides has been explored to improve the cellular uptake of various molecules, including antigens and adjuvants. These vaccine research tools offer unique advantages for vaccine development, and their continued development holds great promise for enhancing the efficacy of vaccines against various diseases. The schematic illustration was drawn using Figdraw tools (www.figdraw.com).
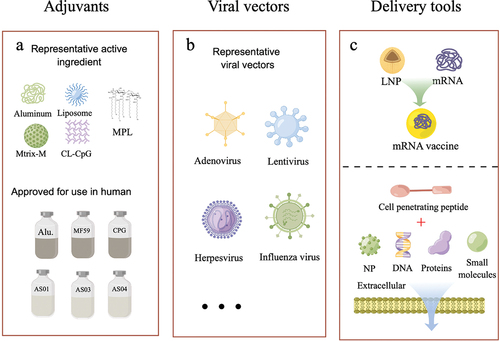
Aluminum adjuvants, widely recognized as excellent immunological adjuvants used in numerous vaccines, have a limited capacity to induce Th2 humoral immunity [Citation132,Citation133]. Fortunately, advancements in the field have led to the development of novel adjuvants that address this limitation and offer improved outcomes. The AS01 adjuvant system, utilized in the herpes zoster (Shingrix®), malaria vaccine RTS,S (Mosquirix), and RSV vaccine, has demonstrated efficacy in clinical trials [Citation134]. This system contains two immunostimulants, 3-O-desacyl-4’-monophosphoryl lipid A (MPL) and Quillaja Saponaria-derived QS-21(TLR4 agonist), that synergize to activate specific T cell responses and promote potent adaptive immunity [Citation135]. For example, Shingrix, a recombinant subunit vaccine comprising VZV glycoprotein gE and the robust AS01B adjuvant, has been approved by the FDA to prevent herpes zoster in adults aged 50 and older. The vaccine has an overall efficacy of 97.2% in the elderly (50 years and above) [Citation136]. Matrix-M is another saponin-based adjuvant formulated with cholesterol and phospholipids into particles. NVX-CoV2373 (Nuvaxovid®), an adjuvanted (Matrix-M) recombinant protein vaccine, conferred 89.7% protection against SARS-CoV-2 infection during the pandemic of the Alpha variant [Citation137]. These plant-derived adjuvants enhance the magnitude and durability of the immune response in the presence of antigens, making them attractive tools for vaccine development against complex infectious diseases. Additionally, GLA-SE, a novel oil-in-water nanoadjuvant formulation incorporating a crucial TLR4 agonist (Glucopyranosyl Lipid A, GLA), is another promising development. In a randomized, double-blind clinical trial, the combination of GLA-SE and the ID93 antigen led to elevated levels of specific antibodies against ID93 in the human body. Moreover, it significantly augmented the population of CD4+ T cells and the diversity of polyfunctional cytokines. Clinical data demonstrated its safety, suggesting that GLA-SE is a secure and efficacious novel adjuvant that holds promise for future vaccine development [Citation138].
Adjuvants are essential components of many vaccines that can enhance and modulate the immune response to a particular antigen. However, the safety considerations involved in their use have resulted in strict review standards for their inclusion in vaccine products. Despite effective immunomodulatory effects demonstrated in preclinical studies, only six adjuvants have been globally approved for use to date, namely aluminum salt adjuvant, MF-59 (Fluad®), AS01 (Shingrix®), AS03 (Pandemrix® and Arepanrix®), AS04 (Fendrix® and Cervarix®), and CpG1018(TLR9 agonist.)(HEPLISAV-B). Recent research has indicated that oil emulsion adjuvants or liposomes alone may not meet the requirements of the next generation of vaccines, and the trend in future adjuvant development is to incorporate specific immunomodulators into aluminum adjuvants, emulsions, and liposomes [Citation139]. However, as vaccines are administered to healthy people, including the elderly and infants, the feasibility of these next-generation molecular adjuvants must be fully evaluated. The benefits of their use in vaccine efficacy must be weighed against their potential inflammatory side effects, and adjuvant components and production processes must be optimized. We noted that although the AS01B adjuvant exhibits good overall tolerance, Shingrix showed more adverse reactions compared to Zostavax in clinical trials [Citation140,Citation141]. Additionally, in-depth research into the mechanism of action of adjuvants is necessary to lay the foundation for developing safer and more efficient molecular adjuvants [Citation142].
5. Viral vectors
The utilization of viral vectors for gene therapy dates back to the early days of virology [Citation143]. However, due to the continuous evolution of virology, viral vectors have now become important engineering tools that facilitate the creation of a diverse array of innovative vector vaccines () [Citation144]. By introducing target antigen sequences at appropriate positions in the viral vector, the vector can deliver the antigen to host cells, thereby triggering a comprehensive immune response [Citation145]. Among the various vector tools available, replication-defective viruses such as adenovirus, lentivirus, and poxvirus are particularly attractive for vaccine development due to their safety, wide range of host cells, low vector immunogenicity, and ability to express exogenous genes for prolonged periods in vivo [Citation146].
Notably, adenovirus vector vaccines have emerged as a popular choice during the COVID-19 pandemic due to their faster clinical conversion speed, as seen in AstraZeneca’s ChAdOx1Ncov-19, Johnson & Johnson’s Ad26.COV.S, and Russia’s Sputnik V [Citation147–149]. Another type of viral vector with the ability to self-replicate, such as herpesviruses, retroviruses, and influenza viruses, offers both safety and efficacy and is an area of focus in new vaccine research [Citation150]. In 2022, Chen and colleagues utilized an influenza virus vector to develop a new COVID-19 vaccine that, when administered nasally, was found to be effective in inducing mucosal immune responses, including resident cell and humoral immunity, as well as mucosal IgA, ultimately resulting in the blockage of viral transmission [Citation151].
While viral vector-based vaccine design has gained widespread attention in the field of vaccine research, concerns remain regarding safety and preexisting immunity in humans. For example, the first chimpanzee Ad-based COVID-19 vaccine, approved for use in multiple countries, has been suspended due to the risk of blood clots [Citation147]. To mitigate the impact of preexisting immunity on vaccine efficacy, Zabdeno/Mvabea employs two distinct viral vectors (Ad26 and MVA) in their Ebola vaccine research [Citation152]. The search for more diverse types of viral vectors may potentially expand their range of applications.
6. Molecular delivery tools
Molecular delivery vehicles are crucial agents that enable the transport of genetically altered pathogens or their associated antigens to the host’s immune system, sustaining their antigenic effects for an extended period of time () [Citation153]. Lipid-based nanocarriers (LNPs) are currently the most commonly employed delivery system in clinical practice. These artificial membrane mimetics are composed of one or multiple lipid groups, including neutral lipids, cationic lipids, cholesterol, and PEG-lipids [Citation154]. Due to their slow-release and superior biocompatibility, LNPs are favored in the field of nucleic acid delivery and have been utilized in the mRNA-based COVID-19 vaccines, BNT162b2 developed by BioNTech and Pfizer and mRNA-1273 developed by Moderna, both of which have demonstrated excellent immunogenic effects in clinical trials [Citation155,Citation156]. LNPs have been developed as a molecular engineering tool for delivering mRNA and have been involved in clinical research on various prophylactic and therapeutic cancer vaccines. However, the preparation process of LNPs can result in oxidation, degradation, and low reproducibility, and some severe allergic reactions have been observed in clinical trials [Citation157,Citation158]. Thus, further optimization of the LNP components and preparation process is still required.
In addition to LNPs, cell-penetrating peptides (CPPs) are widely used molecular delivery tools composed of short cationic or hydrophobic peptide fragments. CPPs can deliver various cargoes into cells, including small molecule-based drugs, peptides, proteins, and DNA/RNA [Citation159]. By enhancing the uptake of antigen molecules by antigen-presenting cells (APCs), CPPs can activate efficient cellular and humoral immune responses [Citation160,Citation161]. Backlund and colleagues tested the enhancing effects of eight previously reported CPPs on peptide vaccines and found that the linkage to CPPs increased the efficacy of peptide vaccines by 25-fold and significantly enhanced antigen accumulation in draining lymph nodes, thereby promoting cross-presentation of CPP peptides [Citation162].
Despite their usefulness, the delivery efficiency of CPP peptides in vivo is still limited by their binding to serum proteins and degradation in lysosomes. To address this, Yu et al. designed an improved chimeric molecule called eTAT (enhanced TAT), which integrates three additional modules onto the TAT sequence, including pH-dependent membrane active peptides (PMAP), endoprotease cleavage sites, and leucine zippers with dimerization ability. In vitro and in vivo studies demonstrated that eTAT overcame the limitations of traditional TAT and exhibited enhanced delivery efficiency. In a mouse model, the therapeutic drug eTAT-Ppm1b reduced the mortality rate of acute liver injury induced by Acetaminophen from 100% to 40%, showing promising therapeutic effects [Citation163]. Moreover, chitosan has garnered significant attention as a promising vaccine delivery system due to its excellent safety profile and capacity to enhance mucosal absorption [Citation164]. With specific modifications, chitosan exhibits favorable compatibility and stability, along with cell-targeting abilities [Citation165–167]. These properties augment cellular uptake, positioning chitosan as a potential tool for vaccine delivery. Chitosan can be formulated into nano-sized particles or microspheres through diverse processes. Numerous studies have shown that chitosan-based nanoparticles effectively deliver various pathogenic antigens, eliciting robust responses from the mucosal, humoral, and cellular immune systems [Citation168,Citation169]. Furthermore, self-assembling nanoparticles (SAPN) and silica particles have demonstrated remarkable efficacy in vaccine delivery [Citation170,Citation171]. Consequently, the rapid development of these delivery tools is expected to foster more successful vaccine research in the future.
Overall, multiple delivery tools have demonstrated effectiveness in the field of vaccine delivery. However, ensuring the safety of delivery systems is of paramount importance and should not be disregarded. Several preclinical studies have shown high inflammatory reactions associated with lipid nanoparticles (LNPs) [Citation172,Citation173]. The Pfizer and Moderna vaccines, for example, have been associated with common adverse reactions, including pain and fever after muscle injection [Citation174,Citation175]. It is plausible that these symptoms are strongly linked to the chemical composition of LNPs. Optimizing this composition could potentially enhance the safety of LNPs. Additionally, the stability of LNPs is closely tied to their chemical composition and delivery substance [Citation176]. These issues represent the primary limitations in the development of LNPs, but it is anticipated that newer molecular techniques will address these concerns in the future.
7. Expert opinion
Vaccines are an essential tool for maintaining global health. Traditional vaccine technology has been widely used in infectious pathogen vaccine research, despite numerous unsuccessful cases. In the development of the next generation of vaccine technology, LNP-based mRNA vaccines may become a new type of vaccine technology that could change vaccine development in the coming years [Citation177]. Currently, further research is needed on mRNA sequence design, LNP formulation and preparation technology, as well as the tolerability and immunogenicity of mRNA vaccines in the human body.
In addition, other aspects deserve closer attention.Viral-vector vaccines targeting multiple pathogens have shown flexibility, efficiency, and great potential for vaccine applications. Conducting thorough safety assessments of viral vectors and implementing effective measures to reduce preexisting immunity are of paramount importance for expanding the range of applications of viral vectors in biomedicine. With the continuous increase in the elderly population, it is necessary to pay extra attention to improving the safety and efficacy of protective vaccines for immunocompromised individuals and the elderly in future vaccine development. Moreover, adjuvants require particular attention. Since established adjuvant tools are still relatively limited, the development of new effective adjuvants is of great significance for the development of new vaccines. Additionally, attention should also be directed toward vaccine strategies capable of simultaneously combating multiple pathogen infections. Chahal et al. developed an mRNA vaccine incorporating replicons from multiple pathogens. A single dose of this vaccine effectively protects against Ebola, H1N1 influenza, and Toxoplasma simultaneously [Citation178]. Therefore, further attention and investment are warranted in exploring and investigating combination vaccine strategies to optimize vaccine development and implementation.
Moreover, enhancing the immune response through the identification of specific antigenic regions/epitopes represents an advanced vaccination strategy. Vaccines designed based on B cell epitopes or T cell epitopes, as well as their combinations, have the potential to induce long-term immunological protection while minimizing adverse reactions to specific structures [Citation179,Citation180]. Numerous studies have demonstrated the efficacy of epitope-based vaccines and have highlighted the significant potential of this vaccination approach [Citation181,Citation182]. However, the design of epitope-based vaccines presents several challenges. The first hurdle involves identifying suitable epitope molecules and their MHC restrictions from complex pathogens or large antigen libraries [Citation183]. This is particularly challenging when developing conservative epitope or multi-epitope vaccines against pathogens that easily mutate [Citation184]. The design of epitope molecules should also carefully consider potential autoimmunity risks, especially regarding CD4+ T cell epitopes [Citation185]. Furthermore, B cell epitope vaccines need to consider antigen conformation and glycosylation patterns, as these factors are crucial for inducing highly effective neutralizing antibodies. Additionally, the rational combination of epitope vaccines with adjuvants can greatly influence their efficacy. While bioinformatics technology provides valuable research tools, further effort and support are necessary for epitope-based vaccine research. Another challenge encountered in vaccine research is addressing diseases with varying cycles. For instance, the varicella-zoster virus (VZV) initially leads to a self-limiting disease known as chickenpox. However, this astute virus can remain dormant in the body and cause a secondary infection with more severe symptoms during periods of immunosenescence [Citation186]. Enhancing the prevention of this pathogen can be achieved through the optimization of vaccination schedules and increased vaccine dosage. Enhancing the prevention of this pathogen can be achieved through the optimization of vaccination schedules and increased vaccine dosage [Citation187]. Another effective strategy involves improving vaccine efficacy by optimizing new adjuvants and T cell epitopes. Shingrix, developed by GSK for varicella-zoster, serves as an excellent example of this strategy [Citation188].
Vaccination undoubtedly plays a crucial role in preventing numerous infectious diseases, with approximately 25 diseases being preventable through WHO-approved vaccines. However, the high costs associated with research and development often translate into expensive vaccine prices, impeding vaccination compliance, particularly in developing nations [Citation189,Citation190]. The decision to pursue vaccine development hinges upon multiple factors, including disease severity, public health impact, existing preventive strategies, and vaccine feasibility. In the case of mild or self-limiting diseases like the common cold, the expenses and resources required for vaccination render vaccine development a lower priority. Some pathogens already have effective interventions, such as antibiotics and antiviral drugs, which can effectively control and alleviate disease impacts, obviating the need for vaccine development. Additionally, certain pathogens can be adequately managed through improvements in sanitation and vector control. In contrast, developing vaccines for formidable pathogens like HIV remains scientifically challenging, necessitating advanced vaccine technologies to achieve effective prevention. To maximize benefits, a comprehensive approach that considers various prevention and treatment measures is essential for effective disease intervention.
Recently, advancements in structural biology technology have enabled the atomic-level structure resolution of most proteins, providing a blueprint for researchers to remodel antigens at the atomic level. Using machine learning and computational analysis, researchers can better understand and analyze the structure and immunogenic components of pathogens. Molecular engineering tools, such as AlphaFold2 and RosettaFold, are now capable of accurately predicting the three-dimensional structure of proteins, which can greatly aid in the development of protein drugs [Citation191,Citation192]. One notable achievement was made in 2020 by David Baker’s team, which successfully designed an inhibitor for the coronavirus protein. This inhibitor can bind to the spike protein of SARS-CoV-2 virus with high affinity at the picomolar level, effectively preventing viral infection of cells [Citation193]. Another remarkable accomplishment was achieved in 2022 by Longxing Cao et al. They developed a new technology for designing binding proteins that can target specific binding sites. This technology can be used by researchers to design drugs for any site of interest [Citation194]. These molecular engineering tools and predictive techniques may open up new fields of protein drug design and help with the precise and rational design of novel vaccine immunogens. Therefore, the rapid advancement of protein redesign technology is of great significance to the development of medicine and technology in the 21st century.
Although the rational use of various molecular engineering tools has led to the development of new vaccine products, it is often challenging to achieve optimal antigen design using a single tool. Therefore, fully exploring the potential of different tools and combining them may help to overcome the limitations of individual tools. For instance, the combined use of CRISPR and Cre-LoxP has been shown to improve gene editing efficiency. Additionally, using the I53–50 particle carrier with a novel adjuvant has greatly enhanced the immunogenicity of subunit vaccines. Consequently, the judicious use of existing tools and the continuous exploration of new ones will undoubtedly facilitate the development of novel vaccines. In summary, the innovation of molecular engineering tools has the potential to overcome challenges that cannot be resolved using traditional vaccine technologies and may serve as a crucial element in the development of vaccines for both current and emerging pathogens in the future.
Article highlights
Vaccination is a highly effective strategy for preventing infectious diseases, and the advancement of new vaccines enhances the ability to control complex pathogens.
Conventional vaccine technologies face limitations when addressing new and intricate pathogens, underscoring the need for diverse vaccine platforms.
Molecular engineering tools expedite the development of innovative vaccine platforms, providing increased flexibility and precision in vaccine design, leading to superior disease protection and potential improvements in global health outcomes.
Gene editing tools refine virus attenuation accuracy, enable new attenuation strategies, and support the development of safer and more efficient attenuated vaccines.
Protein engineering tools offer powerful means to modify proteins, enhancing natural protein functions and unlocking limitless possibilities for creating novel immunogens, especially virus-like particles. These tools can effectively design improved immunogenic proteins, presenting promising prospects in immunology research.
The development of safe and effective subunit vaccines largely depends on molecular adjuvants. The limited availability of clinically approved molecular adjuvants highlights the need to develop diverse, safe, and efficient alternatives.
Virology research has enabled the development of viral-vectored vaccines, which can induce robust and comprehensive immune responses. These vaccines offer potential solutions to challenges posed by complex pathogens.
The success of mRNA vaccines has stimulated extensive research in vaccine development, primarily due to the implementation of advanced delivery systems. The exploration of more efficient delivery technologies could lead to further breakthroughs in vaccine development.
Declaration of interest
The authors have no relevant affiliations or financial involvement with any organization or entity with a financial interest in or financial conflict with the subject matter or material discussed in the manuscript. This includes employment, consultancies, honoraria, stock ownership or options, expert testimony, grants or patents received or mending, or royalties.
Reviewer disclosures
Peer reviewers on this manuscript have no relevant financial or other relationships to disclose.
Author contributions
S.L. and N.S. designed and funded the study. W.X. and T.L. wrote the original draft, reviewed and edited the manuscript, Y.G., N.X., and S.L. participated in discussion and interpretation of the manuscript.
Acknowledgments
We sincerely thank the drawing support provided by the Figdraw platform (www.figdraw.com).
Additional information
Funding
References
- Bloom DE, Cadarette D. Infectious disease threats in the Twenty-First century: strengthening the global response [Review]. Front Immunol. 2019;10:10. doi: 10.3389/fimmu.2019.00549
- Alqutob R, Al Nsour M, Tarawneh MR, et al. COVID-19 Crisis in Jordan: response, Scenarios, Strategies, and Recommendations. JMIR Public Health Surveill. 2020;6(3):e19332. doi: 10.2196/19332
- Stawicki SP, Jeanmonod R, Miller AC, et al. The 2019-2020 novel coronavirus (severe acute respiratory syndrome coronavirus 2) pandemic: a joint American College of Academic International Medicine-World Academic Council of Emergency Medicine Multidisciplinary COVID-19 Working Group Consensus Paper. J Glob Infect Dis. 2020 Apr;12(2):47–93.
- Rémy V, Zöllner Y, Heckmann U. Vaccination: the cornerstone of an efficient healthcare system. J Mark Access Health Policy. 2015;3(1):3. doi: 10.3402/jmahp.v3.27041
- Mühlemann B, Vinner L, Margaryan A, et al. Diverse variola virus (smallpox) strains were widespread in northern Europe in the Viking age. Science. 2020;369(6502):eaaw8977. doi: 10.1126/science.aaw8977
- Trevelyan B, Smallman-Raynor M, Cliff AD. The spatial dynamics of poliomyelitis in the United States: from epidemic emergence to vaccine-induced retreat, 1910–1971. Ann Assoc Am Geogr. 2005;95(2):269–293. doi: 10.1111/j.1467-8306.2005.00460.x
- Bányai K, László B, Duque J, et al. Systematic review of regional and temporal trends in global rotavirus strain diversity in the pre rotavirus vaccine era: insights for understanding the impact of rotavirus vaccination programs. Vaccine. 2012;30:A122–A130. doi: 10.1016/j.vaccine.2011.09.111
- Chang M-H, Chen C-J, Lai M-S, et al. Universal hepatitis B vaccination in Taiwan and the incidence of hepatocellular carcinoma in children. N Engl J Med. 1997;336(26):1855–1859. doi: 10.1056/NEJM199706263362602
- Okada K, Miyazaki C, Kino Y, et al. Phase II and III clinical studies of diphtheria-tetanus-acellular pertussis vaccine containing inactivated polio vaccine derived from sabin strains (DTaP-Sipv)). J Infect Dis. 2013;208(2):275–283. doi: 10.1093/infdis/jit155
- Lehtinen M, Dillner J. Clinical trials of human papillomavirus vaccines and beyond. Nat Rev Clin Oncol. 2013;10(7):400–410. doi: 10.1038/nrclinonc.2013.84
- Sallam M. COVID-19 vaccine hesitancy worldwide: a concise systematic review of vaccine acceptance rates. Vaccines. 2021;9(2):160. doi: 10.3390/vaccines9020160
- Baker RE, Mahmud AS, Miller IF, et al. Infectious disease in an era of global change. Nature Rev Microbiol. 2022;20(4):193–205. doi: 10.1038/s41579-021-00639-z
- Lazarus JV, Romero D, Kopka CJ, et al. A multinational Delphi consensus to end the COVID-19 public health threat. Nature. 2022;611(7935):332–345. doi: 10.1038/s41586-022-05398-2
- Burton DR. Advancing an HIV vaccine; advancing vaccinology. Nat Rev Immunol. 2019;19(2):77–78. doi: 10.1038/s41577-018-0103-6
- Diamond MS, Ledgerwood JE, Pierson TC. Zika virus vaccine development: progress in the face of new challenges. Annu Rev Med. 2019;70(1):121–135. doi: 10.1146/annurev-med-040717-051127
- Enquist LW, DiMaio D, Dermody TS. Recurring revolutions in virology. Annu Rev Virol. 2021;8(1):v–vii. doi: 10.1146/annurev-vi-08-032921-100002
- Baay M, Lina B, Fontanet A, et al. Virology, epidemiology, immunology and vaccine development of SARS-CoV-2, update after nine months of pandemic. Biologicals. 2021;69:76–82. doi: 10.1016/j.biologicals.2020.11.003
- Teng M, Yao Y, Nair V, et al. Latest advances of virology research using CRISPR/Cas9-based gene-editing technology and its application to vaccine development. Viruses. 2021;13(5):779. doi: 10.3390/v13050779
- Debelouchina GT, Muir TW. A molecular engineering toolbox for the structural biologist. Q Rev Biophys. 2017 Jan;50:e7. doi: 10.1017/S0033583517000051
- Liang Z, Zhu H, Wang X, et al. Adjuvants for coronavirus vaccines. Front Immunol. 2020;11:589833. doi: 10.3389/fimmu.2020.589833
- Chang J. Adenovirus vectors: excellent tools for vaccine development. Immune Netw. 2021 Feb;21(1):e6. doi: 10.4110/in.2021.21.e6
- Tenchov R, Bird R, Curtze AE, et al. Lipid nanoparticles─From liposomes to mRNA vaccine delivery, a landscape of research diversity and advancement. ACS Nano. 2021;15(11):16982–17015. doi: 10.1021/acsnano.1c04996
- Ho W, Gao M, Li F, et al. Next-generation vaccines: nanoparticle-mediated DNA and mRNA delivery. Adv Healthcare Mater. 2021;10(8):2001812. doi: 10.1002/adhm.202001812
- Nguyen B, Tolia NH. Protein-based antigen presentation platforms for nanoparticle vaccines. NPJ Vaccines. 2021;6(1):70. doi: 10.1038/s41541-021-00330-7
- Kühn R, Torres RM. Cre/loxP recombination system and gene targeting. Methods Mol Biol. 2002;180:175–204.
- McLellan MA, Rosenthal NA, Pinto AR. Cre-loxP-Mediated recombination: general principles and experimental considerations. Curr Protoc Mouse Biol. 2017;7(1):1–12. doi: 10.1002/cpmo.22
- Minor PD. Live attenuated vaccines: historical successes and current challenges. Virology. 2015;479:379–392. doi: 10.1016/j.virol.2015.03.032
- Lauer KB, Borrow R, Blanchard TJ, et al. Multivalent and multipathogen viral vector vaccines. Clin Vaccin Immunol. 2017;24(1):e00298–16. doi: 10.1128/CVI.00298-16
- Hall SL, Sarris CM, Tierney EL, et al. A cold-adapted mutant of parainfluenza virus type 3 is attenuated and protective in chimpanzees. J Infect Dis. 1993;167(4):958–962. doi: 10.1093/infdis/167.4.958
- Pliaka V, Kyriakopoulou Z, Markoulatos P. Risks associated with the use of live-attenuated vaccine poliovirus strains and the strategies for control and eradication of paralytic poliomyelitis. Expert Rev Vaccines. 2012;11(5):609–628. doi: 10.1586/erv.12.28
- Lauring AS, Jones JO, Andino R. Rationalizing the development of live attenuated virus vaccines. Nat Biotechnol. 2010;28(6):573–579. doi: 10.1038/nbt.1635
- Borca MV, Holinka LG, Berggren KA, et al. CRISPR-Cas9, a tool to efficiently increase the development of recombinant African swine fever viruses. Sci Rep. 2018;8(1):3154. doi: 10.1038/s41598-018-21575-8
- Tang N, Zhang Y, Pedrera M, et al. A simple and rapid approach to develop recombinant avian herpesvirus vectored vaccines using CRISPR/Cas9 system. Vaccine. 2018;36(5):716–722. doi: 10.1016/j.vaccine.2017.12.025
- Wang W, Pan D, Fu W, et al. Development of a skin- and neuro-attenuated live vaccine for varicella. Nat Commun. 2022;13(1):824. doi: 10.1038/s41467-022-28329-1
- Mo Z-J, Huang S-J, Qiu L-X, et al. Safety and immunogenicity of a skin- and neuro-attenuated live vaccine for varicella: a randomized, double-blind, controlled, dose-escalation and age de-escalation phase 1 clinical trial. Lancet Reg Health West Pac. 2023;34:100707. doi: 10.1016/j.lanwpc.2023.100707
- Liang X, Sun L, Yu T, et al. A CRISPR/Cas9 and Cre/Lox system-based express vaccine development strategy against re-emerging Pseudorabies virus. Sci Rep. 2016;6(1):19176. doi: 10.1038/srep19176
- Song AJ, Palmiter RD. Detecting and avoiding problems when using the Cre-lox system. Trends Genet. 2018 May;34(5):333–340. doi: 10.1016/j.tig.2017.12.008
- Harno E, Cottrell EC, White A. Metabolic pitfalls of CNS Cre-based technology. Cell Metab. 2013 Jul 2;18(1):21–28. doi: 10.1016/j.cmet.2013.05.019
- Mosberg JA, Lajoie MJ, Church GM. Lambda red recombineering in Escherichia coli occurs through a fully single-stranded intermediate. Genetics. 2010 Nov;186(3):791–799. doi: 10.1534/genetics.110.120782
- Pyne ME, Moo-Young M, Chung DA, et al. Coupling the CRISPR/Cas9 system with lambda red recombineering enables simplified chromosomal gene replacement in Escherichia coli. Appl Environ Microbiol. 2015 Aug;81(15):5103–5114.
- Huang X, Wang X, Zhang J, et al. Escherichia coli-derived virus-like particles in vaccine development. NPJ Vaccines. 2017;2(1):3. doi: 10.1038/s41541-017-0006-8
- Batista P, Lopes AM, Mazzola PG, et al. Methods of endotoxin removal from biological preparations: a review. 2007.
- Silva F, Queiroz JA, Domingues FC. Evaluating metabolic stress and plasmid stability in plasmid DNA production by Escherichia coli. Biotechnol Adv. 2012;30(3):691–708. doi: 10.1016/j.biotechadv.2011.12.005
- Wang K, Zhou L, Chen T, et al. Engineering for an HPV 9-valent vaccine candidate using genomic constitutive over-expression and low lipopolysaccharide levels in Escherichia coli cells. Microb Cell Fact. 2021;20(1):227. doi: 10.1186/s12934-021-01719-8
- Mosberg JAW. Studying and improving Lambda Red recombination for genome engineering in Escherichia coli. Harvard University; 2013.
- Kozovska Z, Rajcaniova S, Munteanu P, et al. CRISPR: history and perspectives to the future. Biomed Pharmacother. 2021;141:111917. doi: 10.1016/j.biopha.2021.111917
- Adli M. The CRISPR tool kit for genome editing and beyond. Nat Commun. 2018;9(1):1911. doi: 10.1038/s41467-018-04252-2
- Zhang Y, Showalter AM. CRISPR/Cas9 genome editing technology: a valuable tool for understanding plant cell wall biosynthesis and function. Front Plant Sci. 2020;11:589517. doi: 10.3389/fpls.2020.589517
- Sharma G, Sharma AR, Bhattacharya M, et al. CRISPR-Cas9: a preclinical and clinical perspective for the treatment of human diseases. Mol Ther. 2021;29(2):571–586. doi: 10.1016/j.ymthe.2020.09.028
- Wang D, Wang X-W, Peng X-C, et al. CRISPR/Cas9 genome editing technology significantly accelerated herpes simplex virus research. Cancer Genet Ther. 2018;25(5–6):93–105. doi: 10.1038/s41417-018-0016-3
- Maggio I, Zittersteijn HA, Wang Q, et al. Integrating gene delivery and gene-editing technologies by adenoviral vector transfer of optimized CRISPR-Cas9 components. Genet Ther. 2020;27(5):209–225. doi: 10.1038/s41434-019-0119-y
- Xu A, Qin C, Lang Y, et al. A simple and rapid approach to manipulate pseudorabies virus genome by CRISPR/Cas9 system. Biotechnol Lett. 2015;37(6):1265–1272. doi: 10.1007/s10529-015-1796-2
- Chen Y-C, Sheng J, Trang P, et al. Potential application of the CRISPR/Cas9 system against herpesvirus infections. Viruses. 2018;10(6):291. doi: 10.3390/v10060291
- Giuliano CJ, Lin A, Girish V, et al. Generating single cell–derived knockout clones in mammalian cells with CRISPR/Cas9. Curr Protoc Mol Biol. 2019;128(1):e100. doi: 10.1002/cpmb.100
- Gowripalan A, Smith S, Stefanovic T, et al. Rapid poxvirus engineering using CRISPR/Cas9 as a selection tool. Commun Biol. 2020;3(1):643. doi: 10.1038/s42003-020-01374-6
- Zhang W-W, Karmakar S, Gannavaram S, et al. A second generation leishmanization vaccine with a markerless attenuated Leishmania major strain using CRISPR gene editing. Nat Commun. 2020;11(1):3461. doi: 10.1038/s41467-020-17154-z
- Wu W, Orr-Burks N, Karpilow J, et al. Development of improved vaccine cell lines against rotavirus. Sci Data. 2017;4(1):170021. doi: 10.1038/sdata.2017.21
- Sanden S, Wu W, Dybdahl-Sissoko N, et al. Engineering enhanced vaccine cell lines to eradicate vaccine-preventable diseases: the polio end game. J Virol. 2016;90(4):1694–1704. doi: 10.1128/JVI.01464-15
- Karimian A, Azizian K, Parsian H, et al. CRISPR/Cas9 technology as a potent molecular tool for gene therapy. J Cell Physiol. 2019;234(8):12267–12277. doi: 10.1002/jcp.27972
- Li L, Hu S, Chen X. Non-viral delivery systems for CRISPR/Cas9-based genome editing: challenges and opportunities. Biomaterials. 2018;171:207–218. doi: 10.1016/j.biomaterials.2018.04.031
- Nishimasu H, Yamano T, Gao L, et al. Structural basis for the altered PAM recognition by engineered CRISPR-Cpf1. Molecular Cell. 2017;67(1):139–147.e2. doi: 10.1016/j.molcel.2017.04.019
- Suzuki K, Tsunekawa Y, Hernandez-Benitez R, et al. In vivo genome editing via CRISPR/Cas9 mediated homology-independent targeted integration. Nature. 2016;540(7631):144–149. doi: 10.1038/nature20565
- Segel M, Lash B, Song J, et al. Mammalian retrovirus-like protein PEG10 packages its own mRNA and can be pseudotyped for mRNA delivery. Science. 2021;373(6557):882–889. doi: 10.1126/science.abg6155
- Bi Y, Hua Z, Liu X, et al. Isozygous and selectable marker-free MSTN knockout cloned pigs generated by the combined use of CRISPR/Cas9 and Cre/LoxP. Sci Rep. 2016;6(1):31729. doi: 10.1038/srep31729
- Wang L, Schultz PG. Expanding the genetic code. Angewandte Chemie. 2005;44(1):34–66. doi: 10.1002/anie.200460627
- Xie J, Schultz PG. A chemical toolkit for proteins—an expanded genetic code. Nat Rev Mol Cell Biol. 2006;7(10):775–782. doi: 10.1038/nrm2005
- Davis L, Chin JW. Designer proteins: applications of genetic code expansion in cell biology. Nat Rev Mol Cell Biol. 2012;13(3):168–182. doi: 10.1038/nrm3286
- Koehler C, Sauter PF, Wawryszyn M, et al. Genetic code expansion for multiprotein complex engineering. Nat Methods. 2016;13(12):997–1000. doi: 10.1038/nmeth.4032
- Chin JW. Expanding and reprogramming the genetic code. Nature. 2017;550(7674):53–60. doi: 10.1038/nature24031
- Si L, Xu H, Zhou X, et al. Generation of influenza a viruses as live but replication-incompetent virus vaccines. Science. 2016;354(6316):1170–1173. doi: 10.1126/science.aah5869
- Wang N, Li Y, Niu W, et al. Construction of a live-attenuated HIV-1 vaccine through genetic code expansion. Angewandte Chemie. 2014;53(19):4867–4871. doi: 10.1002/anie.201402092
- Wang T-Y, Sang G-J, Wang Q, et al. Generation of premature termination codon (PTC)-harboring pseudorabies virus (PRV) via genetic code expansion technology. Viruses. 2022;14(3):572. doi: 10.3390/v14030572
- Zhang RR, Ye Q, Li XF, et al. Construction and characterization of UAA-controlled recombinant Zika virus by genetic code expansion. Sci China Life Sci. 2021 Jan;64(1):171–173.
- Fok JA, Mayer C. Genetic-code-expansion strategies for vaccine development. Chembiochem. 2020;21(23):3291–3300. doi: 10.1002/cbic.202000343
- Shandell MA, Tan Z, Cornish VW. Genetic code expansion: a brief history and perspective. Biochemistry. 2021;60(46):3455–3469. doi: 10.1021/acs.biochem.1c00286
- Quax TE, Claassens NJ, Söll D, et al. Codon bias as a means to fine-tune gene expression. Molecular Cell. 2015;59(2):149–161. doi: 10.1016/j.molcel.2015.05.035
- Broadbent AJ, Santos CP, Anafu A, et al. Evaluation of the attenuation, immunogenicity, and efficacy of a live virus vaccine generated by codon-pair bias de-optimization of the 2009 pandemic H1N1 influenza virus, in ferrets. Vaccine. 2016;34(4):563–570. doi: 10.1016/j.vaccine.2015.11.054
- Groenke N, Trimpert J, Merz S, et al. Mechanism of virus attenuation by codon pair deoptimization. Cell Rep. 2020;31(4):107586. doi: 10.1016/j.celrep.2020.107586
- Konopka-Anstadt JL, Campagnoli R, Vincent A, et al. Development of a new oral poliovirus vaccine for the eradication end game using codon deoptimization. NPJ Vaccines. 2020;5(1):26. doi: 10.1038/s41541-020-0176-7
- Coleman JR, Papamichail D, Skiena S, et al. Virus attenuation by genome-scale changes in codon pair bias. Science. 2008;320(5884):1784–1787. doi: 10.1126/science.1155761
- Mueller S, Coleman JR, Papamichail D, et al. Live attenuated influenza virus vaccines by computer-aided rational design. Nat Biotechnol. 2010;28(7):723–726. doi: 10.1038/nbt.1636
- Le Nouën C, Brock LG, Luongo C, et al. Attenuation of human respiratory syncytial virus by genome-scale codon-pair deoptimization. Proc Nat Acad Sci. 2014;111(36):13169–13174. doi: 10.1073/pnas.1411290111
- Jordan-Paiz A, Franco S, Martinez MA. Synonymous codon pair recoding of the HIV-1 env gene affects virus replication capacity. Cells. 2021;10(7):1636. doi: 10.3390/cells10071636
- Li P, Ke X, Wang T, et al. Zika virus attenuation by codon pair deoptimization induces sterilizing immunity in mouse models. J Virol. 2018;92(17):e00701–18. doi: 10.1128/JVI.00701-18
- Nouën CL, Luongo CL, Yang L, et al. Optimization of the codon pair usage of human respiratory syncytial virus paradoxically resulted in reduced viral replication in vivo and reduced immunogenicity. J Virol. 2020;94(2):e01296–19. doi: 10.1128/JVI.01296-19
- Zakeri B, Fierer JO, Celik E, et al. Peptide tag forming a rapid covalent bond to a protein, through engineering a bacterial adhesin. Proc Natl Acad Sci U S A. 2012;109(12):E690–E697. doi: 10.1073/pnas.1115485109
- Schoene C, Fierer JO, Bennett SP, et al. SpyTag/SpyCatcher cyclization confers resilience to boiling on a mesophilic enzyme. Angewandte Chemie. 2014;53(24):6101–6104. doi: 10.1002/anie.201402519
- Ma X, Zou F, Yu F, et al. Nanoparticle vaccines based on the receptor binding domain (RBD) and heptad repeat (HR) of SARS-CoV-2 elicit robust protective immune responses. Immunity. 2020;53(6):1315–1330. e9. doi: 10.1016/j.immuni.2020.11.015
- Cohen AA, Gnanapragasam PNP, Lee YE, et al. Mosaic nanoparticles elicit cross-reactive immune responses to zoonotic coronaviruses in mice. Science. 2021;371(6530):735–741. doi: 10.1126/science.abf6840
- Reddington SC, Howarth M. Secrets of a covalent interaction for biomaterials and biotechnology: SpyTag and SpyCatcher. Curr Opin Chem Biol. 2015;29:94–99. doi: 10.1016/j.cbpa.2015.10.002
- Paterson GK, Mitchell TJ. The biology of Gram-positive sortase enzymes. Trends Microbiol. 2004;12(2):89–95. doi: 10.1016/j.tim.2003.12.007
- Spirig T, Weiner EM, Clubb RT. Sortase enzymes in Gram-positive bacteria. Mol Microbiol. 2011;82(5):1044–1059. doi: 10.1111/j.1365-2958.2011.07887.x
- Obeng EM, Fulcher AJ, Wagstaff KM. Harnessing sortase a transpeptidation for advanced targeted therapeutics and vaccine engineering. Biotechnol Adv. 2023;64:108108. doi: 10.1016/j.biotechadv.2023.108108
- Tang S, Xuan B, Ye X, et al. A modular vaccine development platform based on sortase-mediated site-specific tagging of antigens onto virus-like particles. Sci Rep. 2016;6(1):25741. doi: 10.1038/srep25741
- Saunders KO, Lee E, Parks R, et al. Neutralizing antibody vaccine for pandemic and pre-emergent coronaviruses. Nature. 2021;594(7864):553–559. doi: 10.1038/s41586-021-03594-0
- Xu Z, Rivera-Hernandez T, Chatterjee O, et al. Semisynthetic, self-adjuvanting vaccine development: efficient, site-specific sortase A-mediated conjugation of Toll-like receptor 2 ligand FSL-1 to recombinant protein antigens under native conditions and application to a model group a streptococcal vaccine. JControlled Release. 2020;317:96–108. doi: 10.1016/j.jconrel.2019.11.018
- Duarte JN, Cragnolini JJ, Swee LK, et al. Generation of immunity against pathogens via single-domain antibody–antigen constructs. J Immunol. 2016;197(12):4838–4847. doi: 10.4049/jimmunol.1600692
- Morgan HE, Turnbull WB, Webb ME. Challenges in the use of sortase and other peptide ligases for site-specific protein modification. Chem Soc Rev. 2022;51(10):4121–4145. doi: 10.1039/D0CS01148G
- Békés M, Langley DR, Crews CM. PROTAC targeted protein degraders: the past is prologue. Nat Rev Drug Discov. 2022;21(3):181–200. doi: 10.1038/s41573-021-00371-6
- Paiva S-L, Crews CM. Targeted protein degradation: elements of PROTAC design. Curr Opin Chem Biol. 2019;50:111–119. doi: 10.1016/j.cbpa.2019.02.022
- Si L, Shen Q, Li J, et al. Generation of a live attenuated influenza a vaccine by proteolysis targeting. Nat Biotechnol. 2022;40(9):1370–1377. doi: 10.1038/s41587-022-01381-4
- Chatterjee P, Ponnapati M, Kramme C, et al. Targeted intracellular degradation of SARS-CoV-2 via computationally optimized peptide fusions. Commun Biol. 2020;3(1):715. doi: 10.1038/s42003-020-01470-7
- Li K, Crews CM. Protacs: past, present and future. Chem Soc Rev. 2022;51(12):5214–5236. doi: 10.1039/D2CS00193D
- Gadd MS, Testa A, Lucas X, et al. Structural basis of PROTAC cooperative recognition for selective protein degradation. Nat Chem Biol. 2017;13(5):514–521. doi: 10.1038/nchembio.2329
- Ahmad H, Zia B, Husain H, et al. Recent advances in PROTAC-based antiviral strategies. Vaccines. 2023;11(2):270. doi: 10.3390/vaccines11020270
- Zeng S, Huang W, Zheng X, et al. Proteolysis targeting chimera (PROTAC) in drug discovery paradigm: recent progress and future challenges. Eur J Med Chem. 2021;210:112981. doi: 10.1016/j.ejmech.2020.112981
- Gao H, Sun X, Rao Y. PROTAC Technology: opportunities and challenges. ACS Med Chem Lett. 2020;11(3):237–240. doi: 10.1021/acsmedchemlett.9b00597
- Gainza-Cirauqui P, Correia BE. Computational protein design—the next generation tool to expand synthetic biology applications. Curr Opin Biotechnol. 2018;52:145–152. doi: 10.1016/j.copbio.2018.04.001
- Bale JB, Gonen S, Liu Y, et al. Accurate design of megadalton-scale two-component icosahedral protein complexes. Science. 2016;353(6297):389–394. doi: 10.1126/science.aaf8818
- Arunachalam PS, Walls AC, Golden N, et al. Adjuvanting a subunit COVID-19 vaccine to induce protective immunity. Nature. 2021;594(7862):253–258. doi: 10.1038/s41586-021-03530-2
- Boyoglu-Barnum S, Ellis D, Gillespie RA, et al. Quadrivalent influenza nanoparticle vaccines induce broad protection. Nature. 2021;592(7855):623–628. doi: 10.1038/s41586-021-03365-x
- Marcandalli J, Fiala B, Ols S, et al. Induction of potent neutralizing antibody responses by a designed protein nanoparticle vaccine for respiratory syncytial virus. Cell. 2019;176(6):1420–1431.e17. doi: 10.1016/j.cell.2019.01.046
- Sliepen K, Radić L, Capella-Pujol J, et al. Induction of cross-neutralizing antibodies by a permuted hepatitis C virus glycoprotein nanoparticle vaccine candidate. Nat Commun. 2022;13(1):7271. doi: 10.1038/s41467-022-34961-8
- Kay E, Cuccui J, Wren BW. Recent advances in the production of recombinant glycoconjugate vaccines. NPJ Vaccines. 2019;4(1):16. doi: 10.1038/s41541-019-0110-z
- Perrett KP, Nolan TM, McVernon J. A licensed combined haemophilus influenzae type b-serogroups C and Y meningococcal conjugate vaccine. Infect Dis Ther. 2013;2(1):1–13. doi: 10.1007/s40121-013-0007-5
- McCarthy PC, Sharyan A, Sheikhi Moghaddam L. Meningococcal vaccines: current status and emerging strategies. Vaccines. 2018;6(1):12. doi: 10.3390/vaccines6010012
- Grijalva CG, Nuorti JP, Arbogast PG, et al. Decline in pneumonia admissions after routine childhood immunisation with pneumococcal conjugate vaccine in the USA: a time-series analysis. Lancet. 2007;369(9568):1179–1186. doi: 10.1016/S0140-6736(07)60564-9
- Dow JM, Mauri M, Scott TA, et al. Improving protein glycan coupling technology (PGCT) for glycoconjugate vaccine production. Expert Rev Vaccines. 2020;19(6):507–527. doi: 10.1080/14760584.2020.1775077
- Berti F, Micoli F. Improving efficacy of glycoconjugate vaccines: from chemical conjugates to next generation constructs. Curr Opin Immunol. 2020;65:42–49. doi: 10.1016/j.coi.2020.03.015
- Reglinski M, Ercoli G, Plumptre C, et al. A recombinant conjugated pneumococcal vaccine that protects against murine infections with a similar efficacy to Prevnar-13. NPJ Vaccines. 2018;3(1):53. doi: 10.1038/s41541-018-0090-4
- Hatz CFR, Bally B, Rohrer S, et al. Safety and immunogenicity of a candidate bioconjugate vaccine against Shigella dysenteriae type 1 administered to healthy adults: a single blind, partially randomized Phase I study. Vaccine. 2015;33(36):4594–4601. doi: 10.1016/j.vaccine.2015.06.102
- Riddle MS, Kaminski RW, Paolo CD, et al. Safety and immunogenicity of a candidate bioconjugate vaccine against shigella flexneri 2a administered to healthy adults: a single-blind, randomized phase I study. Clin Vaccin Immunol. 2016;23(12):908–917. doi: 10.1128/CVI.00224-16
- Huttner A, Hatz C, van den Dobbelsteen G, et al. Safety, immunogenicity, and preliminary clinical efficacy of a vaccine against extraintestinal pathogenic Escherichia coli in women with a history of recurrent urinary tract infection: a randomised, single-blind, placebo-controlled phase 1b trial. Lancet Infect Dis. 2017;17(5):528–537. doi: 10.1016/S1473-3099(17)30108-1
- Napiórkowska M, Boilevin J, Darbre T, et al. Structure of bacterial oligosaccharyltransferase PglB bound to a reactive LLO and an inhibitory peptide. Sci Rep. 2018;8(1):16297. doi: 10.1038/s41598-018-34534-0
- Moyle PM, Toth I. Modern subunit vaccines: development, components, and research opportunities. ChemMedchem. 2013;8(3):360–376. doi: 10.1002/cmdc.201200487
- Yang S, Li Y, Dai L, et al. Safety and immunogenicity of a recombinant tandem-repeat dimeric RBD-based protein subunit vaccine (ZF2001) against COVID-19 in adults: two randomised, double-blind, placebo-controlled, phase 1 and 2 trials. Lancet Infect Dis. 2021;21(8):1107–1119. doi: 10.1016/S1473-3099(21)00127-4
- Dagnew AF, Ilhan O, Lee W-S, et al. Immunogenicity and safety of the adjuvanted recombinant zoster vaccine in adults with haematological malignancies: a phase 3, randomised, clinical trial and post-hoc efficacy analysis. Lancet Infect Dis. 2019;19(9):988–1000. doi: 10.1016/S1473-3099(19)30163-X
- Li Z, Song S, He M, et al. Rational design of a triple-type human papillomavirus vaccine by compromising viral-type specificity. Nat Commun. 2018;9(1):5360. doi: 10.1038/s41467-018-07199-6
- Vartak A, Sucheck SJ. Recent advances in subunit vaccine carriers. Vaccines. 2016;4(2):12. doi: 10.3390/vaccines4020012
- Perrie Y, Mohammed AR, Kirby DJ, et al. Vaccine adjuvant systems: enhancing the efficacy of sub-unit protein antigens. Int J Pharmaceut. 2008;364(2):272–280. doi: 10.1016/j.ijpharm.2008.04.036
- Tandrup Schmidt S, Foged C, Smith Korsholm K, et al. Liposome-based adjuvants for subunit vaccines: formulation strategies for subunit antigens and immunostimulators. Pharmaceutics. 2016;8(1):7. doi: 10.3390/pharmaceutics8010007
- Tomljenovic L, Shaw CA. Aluminum vaccine adjuvants: are they safe? Curr Med Chem. 2011;18(17):2630–2637. doi: 10.2174/092986711795933740
- Aimanianda V, Haensler J, Lacroix-Desmazes S, et al. Novel cellular and molecular mechanisms of induction of immune responses by aluminum adjuvants. Trends Pharmacol Sci. 2009;30(6):287–295. doi: 10.1016/j.tips.2009.03.005
- Laurens MB. RTS,S/AS01 vaccine (Mosquirix™): an overview. Human Vaccines & Immunotherapeutics. 2020 Mar 3;16(3):480–489. doi: 10.1080/21645515.2019.1669415
- Didierlaurent AM, Laupèze B, Di Pasquale A, et al. Adjuvant system AS01: helping to overcome the challenges of modern vaccines. Expert Rev Vaccines. 2017 Jan;16(1):55–63.
- Maltz F, Fidler B. Shingrix: a new herpes zoster vaccine. P T. 2019;44(7):406–433.
- Dunkle LM, Kotloff KL, Gay CL, et al. Efficacy and safety of NVX-CoV2373 in adults in the United States and Mexico. N Engl J Med. 2021;386(6):531–543. doi: 10.1056/NEJMoa2116185
- Coler RN, Day TA, Ellis R, et al. The TLR-4 agonist adjuvant, GLA-SE, improves magnitude and quality of immune responses elicited by the ID93 tuberculosis vaccine: first-in-human trial. NPJ Vaccines. 2018;3(1):34.
- Pulendran B, Arunachalam PS, O’Hagan DT. Emerging concepts in the science of vaccine adjuvants. Nat Rev Drug Discov. 2021;20(6):454–475. doi: 10.1038/s41573-021-00163-y
- Hesse EM, Shimabukuro TT, Su JR, et al. Postlicensure safety surveillance of recombinant zoster vaccine (Shingrix) - United States, October 2017-June 2018. MMWR Morb Mortal Wkly Rep. 2019 Feb 1;68(4):91–94. doi: 10.15585/mmwr.mm6804a4
- Miller ER, Lewis P, Shimabukuro TT, et al. Post-licensure safety surveillance of zoster vaccine live (Zostavax®) in the United States, Vaccine Adverse Event Reporting System (VAERS), 2006–2015. Human Vaccines Immunother. 2018;14(8):1963–1969. doi: 10.1080/21645515.2018.1456598
- Shi S, Zhu H, Xia X, et al. Vaccine adjuvants: understanding the structure and mechanism of adjuvanticity. Vaccine. 2019;37(24):3167–3178. doi: 10.1016/j.vaccine.2019.04.055
- Thomas CE, Ehrhardt A, Kay MA. Progress and problems with the use of viral vectors for gene therapy. Nat Rev Genet. 2003;4(5):346–358. doi: 10.1038/nrg1066
- Travieso T, Li J, Mahesh S, et al. The use of viral vectors in vaccine development. NPJ Vaccines. 2022;7(1):75. doi: 10.1038/s41541-022-00503-y
- Ura T, Okuda K, Shimada M. Developments in viral vector-based vaccines. Vaccines. 2014;2(3):624–641. doi: 10.3390/vaccines2030624
- Robert-Guroff M. Replicating and non-replicating viral vectors for vaccine development. Curr Opin Biotechnol. 2007;18(6):546–556. doi: 10.1016/j.copbio.2007.10.010
- Voysey M, Clemens SAC, Madhi SA, et al. Safety and efficacy of the ChAdOx1 nCoV-19 vaccine (AZD1222) against SARS-CoV-2: an interim analysis of four randomised controlled trials in Brazil, South Africa, and the UK. Lancet. 2021 Jan 9;397(10269):99–111. doi: 10.1016/S0140-6736(20)32661-1
- Sadoff J, Gray G, Vandebosch A, et al. Safety and efficacy of single-dose Ad26.COV2.S vaccine against Covid-19. N Engl J Med. 2021;384(23):2187–2201. doi: 10.1056/NEJMoa2101544
- Jones I, Roy P. Sputnik V COVID-19 vaccine candidate appears safe and effective. Lancet. 2021;397(10275):642–643. doi: 10.1016/S0140-6736(21)00191-4
- Kamel M, El-Sayed A. Utilization of herpesviridae as recombinant viral vectors in vaccine development against animal pathogens. Virus res. 2019;270:197648. doi: 10.1016/j.virusres.2019.197648
- Chen J, Wang P, Yuan L, et al. A live attenuated virus-based intranasal COVID-19 vaccine provides rapid, prolonged, and broad protection against SARS-CoV-2. Sci Bull. 2022;67(13):1372–1387.
- Tomori O, Kolawole MO. Ebola virus disease: current vaccine solutions. Curr Opin Immunol. 2021;71:27–33. doi: 10.1016/j.coi.2021.03.008
- Jain KK. An overview of drug delivery systems. In: Jain K, editor. Drug delivery systems. New York (NY): Springer New York; 2020. p. 1–54. doi: 10.1007/978-1-4939-9798-5_1.
- Mitchell MJ, Billingsley MM, Haley RM, et al. Engineering precision nanoparticles for drug delivery. Nat Rev Drug Discov. 2021;20(2):101–124. doi: 10.1038/s41573-020-0090-8
- Polack FP, Thomas SJ, Kitchin N, et al. Safety and efficacy of the BNT162b2 mRNA Covid-19 vaccine. N Engl J Med. 2020;383(27):2603–2615. doi: 10.1056/NEJMoa2034577
- Baden LR, El Sahly HM, Essink B, et al. Efficacy and safety of the mRNA-1273 SARS-CoV-2 vaccine. N Engl J Med. 2021;384(5):403–416. doi: 10.1056/NEJMoa2035389
- McClements DJ. Edible lipid nanoparticles: digestion, absorption, and potential toxicity. Progress Lipid Res. 2013;52(4):409–423. doi: 10.1016/j.plipres.2013.04.008
- Moghimi SM. Allergic reactions and anaphylaxis to LNP-based COVID-19 vaccines. Mol Ther. 2021;29(3):898–900. doi: 10.1016/j.ymthe.2021.01.030
- Derakhshankhah H, Jafari S. Cell penetrating peptides: a concise review with emphasis on biomedical applications. Biomed Pharmacother. 2018;108:1090–1096. doi: 10.1016/j.biopha.2018.09.097
- Yang J, Luo Y, Shibu MA, et al. Cell-penetrating peptides: efficient vectors for vaccine delivery. Curr Drug Deliv. 2019;16(5):430–443. doi: 10.2174/1567201816666190123120915
- Skwarczynski M, Toth I. Cell-penetrating peptides in vaccine delivery: facts, challenges and perspectives. Ther Deliv. 2019;10(8):465–467. doi: 10.4155/tde-2019-0042
- Backlund CM, Holden RL, Moynihan KD, et al. Cell-penetrating peptides enhance peptide vaccine accumulation and persistence in lymph nodes to drive immunogenicity. Proc Nat Acad Sci. 2022;119(32):e2204078119. doi: 10.1073/pnas.2204078119
- Yu S, Yang H, Li T, et al. Efficient intracellular delivery of proteins by a multifunctional chimaeric peptide in vitro and in vivo. Nat Commun. 2021;12(1):5131. doi: 10.1038/s41467-021-25448-z
- Garg U, Chauhan S, Nagaich U, et al. Current advances in chitosan nanoparticles based drug delivery and targeting. Adv Pharm Bull. 2019 Jun;9(2):195–204.
- Pawar D, Jaganathan K. Mucoadhesive glycol chitosan nanoparticles for intranasal delivery of hepatitis B vaccine: enhancement of mucosal and systemic immune response. Drug Delivery. 2016;23(1):185–194. doi: 10.3109/10717544.2014.908427
- van der Maaden K, Sekerdag E, Schipper P, et al. Layer-by-layer assembly of inactivated poliovirus and N-Trimethyl chitosan on Ph-sensitive microneedles for dermal vaccination. Langmuir. 2015;31(31):8654–8660. doi: 10.1021/acs.langmuir.5b01262
- Hu Z, Chen J, Zhou S, et al. Mouse IP-10 gene delivered by folate-modified chitosan nanoparticles and dendritic/tumor cells fusion vaccine effectively inhibit the growth of hepatocellular carcinoma in mice. Theranostics. 2017;7(7):1942–1952. doi: 10.7150/thno.16236
- Jiang T, Singh B, Li H-S, et al. Targeted oral delivery of BmpB vaccine using porous PLGA microparticles coated with M cell homing peptide-coupled chitosan. Biomaterials. 2014;35(7):2365–2373. doi: 10.1016/j.biomaterials.2013.11.073
- Xu B, Zhang W, Chen Y, et al. Eudragit® L100-coated mannosylated chitosan nanoparticles for oral protein vaccine delivery. Int j biol macromol. 2018;113:534–542. doi: 10.1016/j.ijbiomac.2018.02.016
- Joyce MG, Chen W-H, Sankhala RS, et al. SARS-CoV-2 ferritin nanoparticle vaccines elicit broad SARS coronavirus immunogenicity. Cell Rep. 2021;37(12):110143. doi: 10.1016/j.celrep.2021.110143
- Hong X, Zhong X, Du G, et al. The pore size of mesoporous silica nanoparticles regulates their antigen delivery efficiency. Sci Adv. 2020;6(25):eaaz4462. doi: 10.1126/sciadv.aaz4462
- Freyn AW, Ramos da Silva J, Rosado VC, et al. A multi-targeting, nucleoside-modified mRNA influenza virus vaccine provides broad protection in mice. Mol Ther. 2020;28(7):1569–1584. doi: 10.1016/j.ymthe.2020.04.018
- Lederer K, Castaño D, Atria DG, et al. SARS-CoV-2 mRNA vaccines foster potent antigen-specific germinal center responses associated with neutralizing antibody generation. Immunity. 2020;53(6):1281–1295. e5. doi: 10.1016/j.immuni.2020.11.009
- Jackson LA, Anderson EJ, Rouphael NG, et al. An mRNA vaccine against SARS-CoV-2—preliminary report. N Engl J Med. 2020;383(20):1920–1931. doi: 10.1056/NEJMoa2022483
- Walsh EE, Frenck RW Jr, Falsey AR, et al. Safety and immunogenicity of two RNA-based Covid-19 vaccine candidates. N Engl J Med. 2020;383(25):2439–2450. doi: 10.1056/NEJMoa2027906
- Battaglia L, Gallarate M. Lipid nanoparticles: state of the art, new preparation methods and challenges in drug delivery. Expert Opin Drug Delivery. 2012;9:497–508. doi: 10.1517/17425247.2012.673278
- Maruggi G, Zhang C, Li J, et al. mRNA as a transformative technology for vaccine development to control infectious diseases. Mol Ther. 2019;27(4):757–772. doi: 10.1016/j.ymthe.2019.01.020
- Chahal JS, Khan OF, Cooper CL, et al. Dendrimer-RNA nanoparticles generate protective immunity against lethal Ebola, H1N1 influenza, and Toxoplasma gondii challenges with a single dose. Proc Nat Acad Sci. 2016;113(29):E4133–E4142. doi: 10.1073/pnas.1600299113
- Ahmad TA, Eweida AE, Sheweita SA. B-cell epitope mapping for the design of vaccines and effective diagnostics. Trials Vaccinol. 2016;5:71–83. doi: 10.1016/j.trivac.2016.04.003
- Malonis RJ, Lai JR, Vergnolle O. Peptide-based vaccines: current progress and future challenges. Chem Rev. 2019;120(6):3210–3229. doi: 10.1021/acs.chemrev.9b00472
- He R, Yang X, Liu C, et al. Efficient control of chronic LCMV infection by a CD4 T cell epitope-based heterologous prime-boost vaccination in a murine model. Cell Mol Immunol. 2018;15(9):815–826. doi: 10.1038/cmi.2017.3
- Xu K, Acharya P, Kong R, et al. Epitope-based vaccine design yields fusion peptide-directed antibodies that neutralize diverse strains of HIV-1. Nat Med. 2018;24(6):857–867. doi: 10.1038/s41591-018-0042-6
- Oyarzun P, Ellis JJ, Gonzalez-Galarza FF, et al. A bioinformatics tool for epitope-based vaccine design that accounts for human ethnic diversity: application to emerging infectious diseases. Vaccine. 2015;33(10):1267–1273. doi: 10.1016/j.vaccine.2015.01.040
- van Doorn E, Liu H, Ben-Yedidia T, et al. Evaluating the immunogenicity and safety of a BiondVax-developed universal influenza vaccine (Multimeric-001) either as a standalone vaccine or as a primer to H5N1 influenza vaccine: phase IIb study protocol. Medicine. 2017;96(11). doi: 10.1097/MD.0000000000006339
- Ishina IA, Zakharova MY, Kurbatskaia IN, et al. MHC class II presentation in autoimmunity. Cells. 2023;12(2):314. doi: 10.3390/cells12020314
- Gershon AA, Breuer J, Cohen JI, et al. Varicella zoster virus infection. Nat Rev Dis Primers. 2015;1(1):1–18. doi: 10.1038/nrdp.2015.16
- Keating GM. Shingles (Herpes Zoster) vaccine (Zostavax®): a review in the prevention of herpes zoster and postherpetic neuralgia. BioDrugs. 2016;30(3):243–254. doi: 10.1007/s40259-016-0180-7
- James SF, Chahine EB, Sucher AJ, et al. Shingrix: the new adjuvanted recombinant herpes zoster vaccine. Ann Pharmacother. 2018;52(7):673–680. doi: 10.1177/1060028018758431
- Plotkin S, Robinson JM, Cunningham G, et al. The complexity and cost of vaccine manufacturing – an overview. Vaccine. 2017;35(33):4064–4071. doi: 10.1016/j.vaccine.2017.06.003
- Loayza N. Costs and trade-offs in the fight against the COVID-19 pandemic: a developing country perspective. World Bank Res Policy Briefs. 2020;35:148535.
- Thornton JM, Laskowski RA, Borkakoti N. AlphaFold heralds a data-driven revolution in biology and medicine. Nat Med. 2021;27(10):1666–1669. doi: 10.1038/s41591-021-01533-0
- Schoeder CT, Schmitz S, Adolf-Bryfogle J, et al. Modeling Immunity with Rosetta: methods for antibody and antigen design. Biochemistry. 2021 Mar 23;60(11):825–846. doi: 10.1021/acs.biochem.0c00912
- Cao L, Goreshnik I, Coventry B, et al. De Novo design of picomolar SARS-CoV-2 miniprotein inhibitors. Science. 2020;370(6515):426–431. doi: 10.1126/science.abd9909
- Cao L, Coventry B, Goreshnik I, et al. Design of protein-binding proteins from the target structure alone. Nature. 2022;605(7910):551–560. doi: 10.1038/s41586-022-04654-9