ABSTRACT
Introduction
Antimicrobial resistance (AMR) is responsible for the death of millions worldwide and stands as a major threat to our healthcare systems, which are heavily reliant on antibiotics to fight bacterial infections. The development of vaccines against the main pathogens involved is urgently required as prevention remains essential against the rise of AMR.
Areas covered
A systematic research review was conducted on MEDLINE database focusing on the six AMR pathogens defined as ESKAPE (Enterococcus faecium, Staphylococcus aureus, Klebsiella pneumoniae, Acinetobacter baumannii, Pseudomonas aeruginosa, and Escherichia coli), which are considered critical or high priority pathogens by the World Health Organization (WHO) and the Centers for Disease Control and Prevention (CDC). The analysis was intersecated with the terms carbohydrate, glycoconjugate, bioconjugate, glyconanoparticle, and multiple presenting antigen system vaccines.
Expert opinion
Glycoconjugate vaccines have been successful in preventing meningitis and pneumoniae, and there are high expectations that they will play a key role in fighting AMR. We herein discuss the recent technological, preclinical, and clinical advances, as well as the challenges associated with the development of carbohydrate-based vaccines against leading AMR bacteria, with focus on the ESKAPE pathogens. The need of innovative clinical and regulatory approaches to tackle these targets is also highlighted.
1. Introduction
Bacterial antimicrobial resistance (AMR), a phenomenon resulting in ineffective drugs and persistent infections, is a growing threat and a major global health issue. Associated with around 5 million deaths in 2019, infections by AMR bacteria represent a leading cause of death worldwide, ahead of both human immunodeficiency virus (HIV) and malaria, and is particularly problematic for some of the globe’s poorest regions [Citation1]. Antibiotic resistance is a natural process characterized by mechanisms essentially limiting drug uptake, modifying drug targets, or inactivating drugs. Its occurrence has been greatly increased by massive antibiotic (mis)use [Citation2]. High AMR bacterial burdens are prevalently found in sub-Saharan Africa and south Asia, partly due to a lack of or an excessive use of antibiotics respectively, reflecting the need for nationally tailored actions against this global problem [Citation1].
AMR undermines medical care as a whole, by compromising surgeries, organ transplants, as well as treatments for physical trauma and conditions such as cancer, HIV and diabetes [Citation3]. Six of the leading AMR pathogens (Enterococcus faecium, Staphylococcus aureus, Klebsiella pneumoniae, Acinetobacter baumannii, Pseudomonas aeruginosa, and Enterobacteriacae including Escherichia coli), collectively termed ESKAPE pathogens, along with others, such as Streptococcus pneumoniae, have been identified as critical or high priority pathogens by the WHO and the CDC [Citation1,Citation4].
The global AMR challenge is currently tackled with the development of several complementary approaches, amongst which infection prophylaxis through vaccination remains a crucial one [Citation2]. Vaccination can diminish the spread of AMR pathogens by reducing secondary infections and decrease the need for antibiotics, thereby combating resistance expansion [Citation2]. Existing vaccination programmes against S. pneumoniae, Hib, S. typhi, malaria, rotavirus, seasonal flu, and the recently approved RSV already play an important role in containing antibiotic use and AMR [Citation1,Citation5]. For some of those vaccines, such as the pneumococcal conjugate vaccines (PCV), a continuous update of the vaccine formulation to increase valency is requested to counteract serotype replacement [Citation6,Citation7]. It is also worth noting that the development of veterinary vaccines, particularly against livestock pathogens, represents a desirable approach to reduce antibiotic use in animal and fish farming [Citation8].
Vaccination has the potential to avert antibiotic use impacting on public health and economic burden [Citation9]. Nevertheless, for most of the ESKAPE pathogens [Citation2] vaccination remains an unmet need.
Extracellular polysaccharides (PS) of bacterial pathogens significantly contribute to their virulence mechanisms, while the surface localization and unique structures make these PS ideal candidates for the development of vaccines. Most microbial PS are, however, poorly immunogenic and do not induce a memory response [Citation10]. This challenge has been overcome by coupling carbohydrates to a carrier protein to generate glycoconjugate vaccines [Citation11,Citation12]. The first glycoconjugate vaccines were licensed in the 1980s and since then have become one of the safest and most successful vaccine categories to prevent bacterial infection and reduce carriage [Citation12].
In this review, we will present the latest data and developments on carbohydrate-based vaccines against leading AMR bacterial pathogens and discuss strategies to overcome the hurdles impeding their success in the clinics.
2. From polysaccharides to glycoconjugate vaccines
Most bacteria are coated with a PS network, termed glycocalyx, which includes i) lipid-linked structures, such as lipopolysaccharides (LPS) for Gram-negative and teichoic acids (TA) for Gram-positive species; ii) surface peptidoglycans for Gram-positive species; and iii) capsular polysaccharides (CPS) for Gram-positive and -negative encapsulated bacteria. These surface PS contribute to biofilm formation and fuel virulence by increasing host attachment and supporting immune evasion [Citation12,Citation13].
Plain PS-based vaccines have shown limited clinical efficacy due to their T cell (or thymus)-independent (TI) antigenic character [Citation12,Citation14]. PS are built up with highly repetitive epitopes, and can activate specific B cells in a polyvalent fashion by crosslinking membrane-bound pentameric immunoglobulin M (IgM) B cell receptor (BCR) clusters [Citation14]. However, they typically induce B cells differentiation into plasma cells, without formation of memory B cells (MBC) [Citation12,Citation15–18], resulting in secretion of low-affinity IgM, and to a lesser extent IgG antibodies (mainly IgG2) [Citation17,Citation19]. The mechanism of B cell activation, based on the crosslinking of multiple BCR, explains why the immunogenicity of these vaccines is size-dependent, with only high molecular weight (MW) antigens being able to induce an effective immune response [Citation12]. PS antigens thus activate the immune system without involving major histocompatibility complex class 2 (MHCII) molecules, and consequently without T cell help [Citation17,Citation19].
Adult individuals who have acquired PS-specific MBC prior to vaccination, likely through exposure to relevant pathogens [Citation11,Citation14] or to cross-reacting PS from commensal bacteria or ingested food, can produce PS-specific antibodies after vaccination [Citation11,Citation20]. However, most of these antibodies are IgM and IgG2, which are poor complement activators and therefore less effective [Citation12,Citation17,Citation19]. Additionally, repeated immunization does not lead to increased antibody titers, but can rather trigger hyporesponsiveness [Citation21–23].
PS-based vaccines are not effective in immunologically naïve populations, such as children younger than 2 years, because of the lack of preexisting MBC and the immaturity of their splenic marginal zone (MZ), which is the primary site for B cell stimulation [Citation17,Citation19,Citation24]. In the elderly, limited production of naïve B cells in the splenic MZ prevents induction of a robust antibody production toward PS vaccines [Citation17,Citation19,Citation25].
Of note, not all PS are TI antigens: zwitterionic polysaccharides (ZPS) such as S. aureus CPS type 5 and 8, S. pneumoniae Sp1 and B. fragilis PSA are able to bind MHCII molecule and trigger T cell help[Citation17].
Eliciting a T cell-dependent (TD) immune response against PS can be generally accomplished by coupling carbohydrates to a carrier protein to generate a glycoconjugate vaccine, which contains both B and T cell epitopes (). Glycoconjugate vaccines induce a PS-specific adaptive immune response, a normal B cell affinity maturation in germinal centers, IgM to IgG class-switch recombination, B and T cell memory, and significantly higher protection compared to plain PS vaccines [Citation11,Citation26].
Figure 1. Immunological pathways for processing glycoconjugates. 1) Glycoconjugates vaccines are taken up by antigen-presenting cells (APC) and presented to B cells, which recognize glycan epitopes through the B cell receptor (BCR). 2) Upon BCR recognition, glycoconjugates are engulfed into the B cell via endocytosis. 3) In the cell, glycoconjugates are exposed to acidic conditions and further degraded to fragments by enzymes and reactive oxygen/nitrogen (ROS/RNS) species. 4) The resulting peptides and glycopeptides are loaded onto peptide-binding MHCII complexes, responsible for trafficking and exposing antigens to the cell surface. 5) As MHCII molecules can only bind peptides (and ZPS), only processed peptides were thought to be displayed at the surface for T cell recognition, however, studies have shown that T cells can also recognize carbohydrates, suggesting glycopeptides can also bind MHCII. 6) Primed T helper cells can recognize the presented antigen through their T cell receptor (TCR), and can, upon co-stimulation, initiate B cell maturation and production of memory cells and glycan-specific antibodies. In the absence of peptides, B cells cannot recruit T cell help and do not mount an efficient immune response.
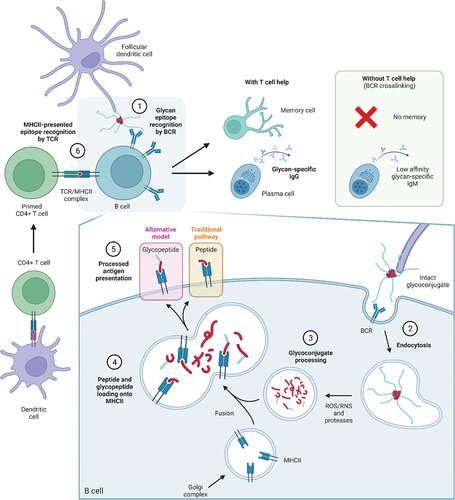
Glycoconjugates are proposed to bind to carbohydrate-specific B cells through BCR or pattern recognition receptors and, upon internalization, the protein component is digested by proteases in the endosomal compartment, forming peptides which interact with MHCII molecules. The resulting complex is then transported to the B cell surface and presented to T cells. Activation of T cells induces B cell maturation, resulting in IgM to IgG class-switching and production of high-affinity carbohydrate-specific antibodies. The generated antigen-specific memory B and T cells can be activated upon exposure to pathogens and consequently provide efficient protection [Citation17,Citation26]. Critical events for mounting an appropriate humoral response, particularly T and B cell interactions in the germinal center, are outside the scope of this review but have been detailed elsewhere [Citation11].
More recently, it has been shown that glycan-peptide fragments can be loaded onto MHCII molecules via the peptide and displayed at the B cell surface resulting in the generation of so-called Tcarb cells (carbohydrate-recognizing CD4+ T helper cells) [Citation27–29]. Carbohydrate-specific T cell-mediated humoral responses have been found for a variety of glycoconjugates (S. pneumoniae type 3 CPS, S. Typhi Vi, S. agalactiae type Ib and H. influenzae type b), although it has not been seen for others, such as meningococcal group C CPS conjugate [Citation30] which suggests coexistence of different processing mechanisms depending on the type of PS [Citation31]. Tcarb activation may be an important element of the anti-carbohydrate immune response and should be taken into account in the design of new glycoconjugates.
3. Carbohydrate-based vaccine technologies
3.1. Glycan antigen production
Licensed glycoconjugate vaccines are commonly produced by chemical conjugation of extracted bacterial PS to a carrier protein. PS are generally purified from bacteria and covalently linked randomly along the saccharide chain to a carrier with the formation of high MW, cross-linked and heterogeneous structures () [Citation32,Citation33]. The production of well-defined and uniform PS populations with a lower MW is sometimes preferred for consistency, as the elicited immune response can then better be correlated with the oligosaccharide’s chemical structure [Citation33,Citation34]. Recently, alternative approaches have been developed to produce oligosaccharides through organic synthesis or chemoenzymatic approaches () [Citation32,Citation33].
Figure 2. Simplified pathways for production of glycoconjugate vaccines. Oligosaccharides can be obtained from isolated PS through hydrolysis or oxidation. Various conjugation chemistries can be employed, resulting in site-selective or random conjugation on the carrier and/or the saccharide. Classical random conjugation strategies (a) usually involve lysine or Cysteine residues (e.g. N-hydroxysuccinimide (NHS) esters or thiol-maleimide reactions) for the protein, and hydroxyl groups for the saccharide (e.g. 1-cyano-4-dimethylaminopyridine (CDAP) activation and conjugation). Oligosaccharides obtained from PS depolymerization or chemoenzymatic methods can be conjugated at the reducing end through reductive amindation or through a linker providing a radial structure. Site-selective conjugation (b) can be achieved by glycoengineering (protein-glycan coupling techonology) or through incorporation of uAA.
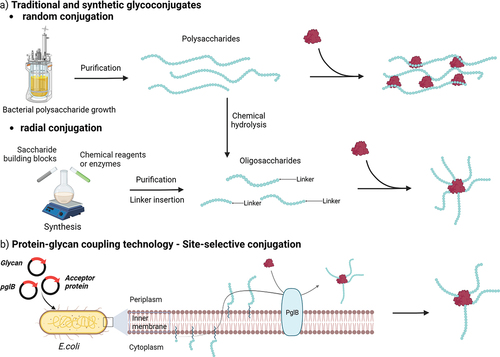
Specifically, organic synthesis has the advantages of producing well-defined and highly pure structures, which are more easily characterizable and devoid of any potential bacterial contaminants [Citation33,Citation34]. Fully synthetic antigens have reached the clinical development phase, notably with a synthetic H. influenzae type b (Hib) vaccine licensed in Cuba [Citation35], a PNAG conjugate that completed Phase I (NCT02853617), and more recently a vaccine against shigellosis that progressed to Phase II [Citation36]. Novel synthetic transformations and deepened insight into glycosylation reaction mechanisms, combined with swifter synthetic protocols, based on one-pot reactions and automated synthesis, are allowing complex bacterial glycans to be more readily available [Citation37]. Achievements in the production of synthetic glycans from PS expressed by AMR pathogens have recently been reviewed [Citation33]. Despite the cost reduction provided by these modern methodologies, the manufacturing of complex multivalent vaccines remains a challenge.
Enzyme-catalyzed oligosaccharide assembly is an environment-friendly alternative to the chemical synthesis of bacterial carbohydrates. which could be attractive for industrialization [Citation34]. Development of enzyme-based approaches has been shown feasible, particularly for meningococcal CPS synthesis [Citation38]. Oligomers with a defined length could be obtained through solid-supported enzyme catalysis with simplified manufacturing and purification [Citation39,Citation40]. The enzymatic approach, however, is limited by the availability of glycosyl donors, due to the variability of bacterial monosaccharides and the potential presence of rare sugars which would need ad hoc synthetic protocols for their preparation. No example of chemoenzymatic glycoconjugate vaccine has so far reached the clinical stage.
3.2. Approaches for glycoconjugation
For long PS, conjugation to the carrier protein is carried out randomly along the carbohydrate chain, taking advantage of the abundant lysine residues and the high reactivity of activated saccharide residues. Because there is no or little control in the regiochemistry of this conjugation chemistry, the random approach results in cross-linked glycoproteins with variable saccharide loading and antigen positioning () [Citation34]. In contrast, conjugation of oligosaccharides to the protein can take place directly through the reducing end of the sugar or via a spacer, to reduce steric hindrance, with the formation of more defined and easy to characterize radial structures () [Citation32]. More selective approaches are required for proteins that serve a dual role as antigen and glycan carrier. Two main strategies for site-selective protein engineering are applied for glycoconjugation: the incorporation of unnatural amino acids (uAA) and the in vivo bioconjugation referred to as protein-glycan coupling technology (PGCT) () [Citation41].
Recent examples of glycoconjugates relying on uAA include the development of a malarial vaccine, in which a glycosylphosphatidylinositol antigen was incorporated at the C-terminal p-azidomethyl phenylalanine of a Malaria protein Pfs25, that was expressed E. coli [Citation42]. A conjugate obtained through a similar approach, based on the polyrhamnose backbone of the universally conserved cell wall carbohydrate from Group A Streptococcus (GAS) and the toxin antigen streptolysin O, generated in mice functional antibodies against both virulence factors and provided protection against systemic GAS challenges [Citation43]. A 24–valent pneumococcal vaccine where PS were site-selectively coupled to engineered CRM197, bearing multiple uAA for conjugation away from the most relevant T cell epitopes, induced opsonophagocytic antibodies in rabbit, raising IgG levels comparable to PCV13 for the same serotypes [Citation44]. The vaccine completed Phase II clinical studies, while a 31–valent vaccine is under preclinical development.
PGCT is a single-step in vivo bioconjugation of the saccharide antigen and the carrier protein, which are both expressed in E. coli, thus avoiding the need for separate expression and purification of each component [Citation45]. The technology is based on the N-linked glycosylation system from Campylobacter jejuni that can be functionally expressed in E. coli [Citation46]. More specifically, PglB, the oligosaccharyltransferase enzyme, couples the PS synthesized on a undecaprenol pyrophosphate lipid anchor within the cytoplasm to the acceptor carrier protein having the acceptor sequon (D/E – X–N – X–S/T) to generate the bioconjugate [Citation32]. Generally, up to 4–5 consensus sequences are inserted within the protein, although full occupancy can be difficult to achieve. PGCT is not limited to the use of PglB, and new enzymes are continuously being discovered to further extend bioconjugation toolbox [Citation47,Citation48].
A variety of bioconjugate vaccines based on O-antigens (A. baumannii, B. pseudomallei, E. coli, F. tularensis, K. pneumoniae, S. dysenteriae, and S. flexneri) and CPS (S. aureus, S. pneumoniae) have been generated and found immunogenic in animal preclinical models [Citation47,Citation49–51]. Some of these bioconjugates are currently undergoing clinical evaluation: a Shigella 2a vaccine [Citation52] was shown to induce anti-lipooligosaccharide antibodies associated with a reduction of disease in a Phase II study [Citation53]; a 9–valent vaccine against extraintestinal pathogenic E. coli (ExPEC9V) to prevent invasive infection in older adults with a history of UTI has advanced to Phase III (NCT04899336) [Citation54] while a S. pneumoniae (NCT03303976) and a 4–valent K. pneumoniae vaccine have entered Phase I studies (NCT04959344) () [Citation32]. The limited amount of saccharides that can be incorporated using site-selective technologies might pose some challenges for less immunogenic PS, and clinical studies will be relevant to inform on this.
3.3. Nanotechnologies for glycoconjugates
Several new technologies to allow the generation of complex multivalent glycoconjugate vaccines are under development. In the last decade, alternative delivery systems have been receiving attention to improve vaccine efficacy and immunogenicity, and a variety of platforms for the multiple presentation of saccharide antigens on pre-arranged and well-ordered matrices have been developed, among which (self-assembling) nanoparticles (NP), generalized modules for membrane antigens (GMMA), and more recently multiple antigen-presenting system (MAPS) technology [Citation64,Citation65]. summarizes the pros and cons associated with each technology.
Table 1. Main advantages and drawbacks for each carbohydrate-based vaccine platform reviewed. We refer the readers to sections 3.1 and 3.2 for carbohydrate production and conjugation details.
3.3.1. Glycoconjugated (self-assembling) nanoparticles
Glyconanoparticles can display antigens with high density in a high order-structure arrangement, as naturally presented by a pathogen, enabling multiple binding events between the nanoparticle (NP) and the host cell BCR. The resulting high avidity can induce a more potent immune response than the single soluble recombinant antigens [Citation64].
In addition, particles in the 50–200 nm size range can be actively transported by migratory cells, such as dendritic cells or macrophages, to lymph nodes which are the primary location of B cell maturation [Citation66]. NP carriers could thus enhance antigen uptake by APC and BCR cross-linking as compared to classic protein carriers [Citation67].
Self-assembling protein NP are protein oligomers assembling in three-dimensional structures which recently attracted interest in the field of vaccine development because of their unique properties, such as biocompatibility, enhanced stability and molecular specificity, leading to efficient delivery and antigen display at their surface [Citation68,Citation69]. Virus-like particle (VLP)-based vaccines deriving from self-assembling viral structural proteins are already used in commercial vaccines () [Citation70]. Examples of VLP-based licensed vaccines include the hepatitis B virus (HBV) vaccine Recombivax HB [Citation71], the human papillomavirus (HPV) vaccines, Gardasil (Merck) and Cervarix (GSK), and the hepatitis E virus vaccine Hecolin [Citation72].
Recently, VLP have been tested as carrier for carbohydrates. An efficacious pneumoccocal glycoconjugate vaccine based on Qβ VLP has been recently shown feasible [Citation73]. VLP from the HBV core antigen have been exploited as carrier for the meningococcal group C CPS, generating a strong immune response in mice [Citation74]. A conjugate vaccine developed by linking purified O-antigen from V. cholerae O1 to Qβ VLP using squarate chemistry generated in mice prominent and long-lasting anti O-antigen IgG antibodies that recognized native LPS from V. cholerae [Citation75].
New self-assembling NP scaffolds computationally designed with precisely control of morphology and characteristics are emerging as tools to assemble different immunological components (i.e. antigens and adjuvants) in order to amplify their immune responses [Citation68,Citation69,Citation76,Citation77]. Vaccine candidates designed through this approach and exposing glycoprotein antigens from respiratory syncytial virus (RSV), influenza and SARS-CoV-2 have been advanced in clinical trials [Citation69,Citation78–81].
3.3.2. Generalized modules for membrane antigens
Gram-negative bacteria generate low amounts of outer membrane vesicles (OMV) through a blebbing process, which can be stimulated through strain engineering to destabilize the membrane and effectively produce generalized modules for membrane antigens (GMMA) (). GMMA faithfully resemble the bacterial outer membrane and possess self-adjuvanting properties through the display of several pathogen-associated molecular patterns, which are conserved small molecular motifs in bacteria that are recognized by pattern recognition receptors on mammalian cells. For example, GMMA expose surface O-polysaccharide (OPS) chains in their natural environment and conformation, thus promoting uptake by immune cells and inducing strong immune responses. GMMA in which the lipid A portion of its LPS has been modified to modulate endotoxicity have, therefore, been proposed as vaccine candidates [Citation82,Citation83]. The safety of S. sonnei GMMA was demonstrated in a Phase I study [Citation34,Citation84,Citation85]. A number of PS from different pathogens (N. meningitidis serogroup A, H. influenzae type b, GAS, and S. Typhi Vi) have been successfully conjugated to GMMA and shown to be immunogenic in animal models [Citation86–88]. The chemical linkage was directed either to proteins or to the LPS expressed on the vesicle surface [Citation87,Citation89,Citation90].
Alternatively, strategies to engineer E. coli to express heterologous PS on GMMA and OMV have been developed [Citation91]. GlycoOMV have been generated by exploiting the PS biosynthesis on the native undecaprenyl pyrophosphate carrier in the cytoplasmic side of the inner membrane and subsequent translocation to the periplasm side through the endogenous flippase Wzx, to enable the final transfer on the lipid A by the endogenous O-antigen ligase WaaL [Citation91]. Glycans can also be assembled from the terminal sugars of the truncated lipid A-core expressed on the cytoplasmic side of the inner membrane and then flipped to the periplasm by the Lpt protein complex for final transfer onto the outer membrane of the vesicles [Citation92]. GlycoOMV expressing OPS from various pathogenic bacteria, including the highly virulent F. tularensis ssp. tularensis type A strain Schu S4 (generating Ft-glycOMV) have been produced through this approach [Citation93]. The glycoOMV induced antibodies protecting mice from a lethal challenge with F. tularensis after passive transfer and elicited a protective IgA-mediated mucosal immune response when subcutaneously administered. GlycoOMV decorated with pneumococcal CPS were also generated and induced serum IgG opsonophagocytic titers comparable to the corresponding chemical conjugates in PCV13 [Citation94]. Finally, the hypervesiculating JC8031 strain of E. coli was engineered for expression of S. aureus PNAG, both in acetylated and deacetylated form (dPNAG) [Citation95]. After mice immunization, the dPNAG-OMV-elicited antibody mediated in vitro killing of PNAG-positive S. aureus and F. tularensis holarctica and protected mice from challenges with the two bacterial species.
3.3.3. Multiple antigen-presenting system technology
The multiple antigen-presenting system (MAPS) enables the creation of a macromolecular complex by integrating glycan and protein antigens to reproduce the antigenic and immunologic strengths of whole-cell vaccines (). In the MAPS, differently from classic conjugate vaccines, the PS and protein are not covalently linked, but affinity binding is exploited to generate multivalent immune complexes, thus mimicking chemical and physical features of a whole-cell constructs for B and T cell activation [Citation96,Citation97]. The MAPS complex is obtained by mixing a target protein antigen, genetically fused to an avidin moiety (termed rhavi), and a biotinylated PS [Citation96]. The immunogenicity of the formed macromolecular construct depends on the size of the PS scaffold, as the protein becomes exposed along its chain. As the MAPS approach allows for the combination of a variety of protein and carbohydrate components, it appears advantageous in the design of vaccines against challenging pathogens, such as S. aureus, a bacterium known to have complex host–pathogen interactions [Citation96,Citation98]. Depending on the size of the glycan-protein complex, the MAPS also allows to achieve Th1/Th17–biased immune responses [Citation96].
MAPS technology appears also to streamline the manufacturing process and this feature has been exploited for the development of 24–valent pneumococcal vaccine that was successful in Phase II clinical studies [Citation99] A multivalent vaccine to fight invasive K. pneumoniae and P. aeruginosa infections is also in progress (Patent no. US11612647B2) [Citation100].
4. Carbohydrates as antigens to target ESKAPE pathogens
Bacterial surface polysaccharides are a very diverse class of biomolecules, that includes capsular polysaccharides (CPS), lipopolysaccharides (LPS), teichoic acids (TA) and exopolysaccharides (). These extracellular carbohydrates are usually crucial to the survival of bacteria and heavily contribute to their virulence. Consequently, they represent a rich source of protective antigens. Notably, some structural overlap in glycans between bacterial strains and even species makes it possible to generate broad-spectrum glycoconjugate vaccines, while passive immunotherapy may be a suitable strategy for treating patients unable to mount an effective immune response during or after an infection. The most recent advances and trends in active and passive immunizations against the ESKAPE pathogens presented in Box 1 are described here and summarized in . Although S. pneumoniae is not part of the ESKAPE group, it is considered a major AMR driver. For an overview of the state of art of pneumococcal conjugate vaccine we refer the readers to other recent reviews [Citation146,Citation147].
Figure 4. a) Overview of the main surface carbohydrate classes and their surface localization on Gram-negative and -positive bacteria. b) Selected examples of polysaccharide RU and relevant oligosaccharides from ESKAPE pathogens. LPS and O-antigens structures are not shown for clarity. For WTA and LTA enterococcal structures see reference [Citation148]. Cross-reactive trisaccharide highlighted for A. baumannii CPS K3. Pel is a partially deacetylated polymer of α-1,4–GlcNAc made up predominantly of the dimeric repeat here shown [Citation149]. Alginate is composed of ManA, that can bear O-acetyls at the C2’ and/or C3’ positions, and interspersed with L-GlcA, resulting in random structures [Citation150]. Common S. aureus WTA is shown with all three possible linkages for GlcNac residues, catalyzed by glycosyltransferases TarS (β-1,4), TarM (α-1,4) and TarP (β-1,3) [Citation151]. dPNAG can be fully or partially deacetylated. All carbohydrates are represented in pyranose form, except when noted f for furanose. All carbohydrates are in D configuration (save Fuc naturally occurring in L configuration), except when stated otherwise (D/L within the symbol). At physiological pH, GlcN, GalN, ManN and Ala residues carry positive charges, while GlcA, ManA and phosphate residues carry negative charges.
![Figure 4. a) Overview of the main surface carbohydrate classes and their surface localization on Gram-negative and -positive bacteria. b) Selected examples of polysaccharide RU and relevant oligosaccharides from ESKAPE pathogens. LPS and O-antigens structures are not shown for clarity. For WTA and LTA enterococcal structures see reference [Citation148]. Cross-reactive trisaccharide highlighted for A. baumannii CPS K3. Pel is a partially deacetylated polymer of α-1,4–GlcNAc made up predominantly of the dimeric repeat here shown [Citation149]. Alginate is composed of ManA, that can bear O-acetyls at the C2’ and/or C3’ positions, and interspersed with L-GlcA, resulting in random structures [Citation150]. Common S. aureus WTA is shown with all three possible linkages for GlcNac residues, catalyzed by glycosyltransferases TarS (β-1,4), TarM (α-1,4) and TarP (β-1,3) [Citation151]. dPNAG can be fully or partially deacetylated. All carbohydrates are represented in pyranose form, except when noted f for furanose. All carbohydrates are in D configuration (save Fuc naturally occurring in L configuration), except when stated otherwise (D/L within the symbol). At physiological pH, GlcN, GalN, ManN and Ala residues carry positive charges, while GlcA, ManA and phosphate residues carry negative charges.](/cms/asset/107c52f8-5ab6-457e-a498-931321bae64a/ierv_a_2274955_f0004_oc.jpg)
Table 2. Current key preclinical carbohydrate-based approaches against ESKAPE pathogens. Use of synthetic glycan is meant by the term ‘semi-synthetic chemical conjugate’.
Table 3. Recent clinical trials for vaccines and passive immunizations against ESKAPE.
4.1. Capsular polysaccharides and lipopolysaccharides
Capsular and bacterial lipopolysaccharides represent the best-known classes of bacterial PS and have consequently been in focus for the development of carbohydrate-based vaccines (). Bacterial capsular polysaccharides (CPS), or K-antigens, are long and incredibly diverse PS chains, produced by both Gram-positive and -negative bacteria. Capsules are covalently linked to the cell surface, compose the outermost layer and have variable functions that can enhance bacterial fitness for survival and alter immune responses to pathogens [Citation152]. Lipopolysaccharides (LPS) are endotoxins found in the outer membrane of Gram-negative bacteria. LPS contain three distinct regions: the lipid A, a glycophospholipid moiety anchoring LPS into the bacterial membrane (); a core oligosaccharide, commonly composed of keto-deoxyoctulosonate (KDO) attached to the lipid A; and the O-antigen, or O-specific polysaccharide (OPS), a repetitive outer glycopolymer unique to each bacterial strain [Citation153]. LPS have long been studied as immunogens and LPS-based conjugates could soon emerge as potent vaccines against Gram-negative bacteria using approaches described in section 4 [Citation154].
4.1.1. A. baumannii
There are more than 90 expected serotypes for the CPS of A. baumannii. Although CPS are the most explored carbohydrate vaccine antigen because of their surface accessibility [Citation155], only 20 CPS structures have been elucidated to date [Citation156]. Monoclonal antibodies (mAb) against A. baumannii K1 CPS bound 13 out of 100 isolates, while showing neutrophil-mediated killing activity in vitro and decreasing bacterial loads in various rat organs after infection [Citation157]. Specific antibodies obtained after immunization with the A. baumannii SK44 CPS reacted to 62% of the 554 tested clinical isolates, suggesting its potential as protective antigen against heterologous strains [Citation118]. The A. baumannii K3 CPS type () consists of a branched pentasaccharide, with a core trisaccharide RU found in the CPS of several strains, including SK44, explaining this cross-reactivity. Oligosaccharides resembling that pentasaccharide were synthesized and screened against sera from infected patients to identify potential vaccine candidates [Citation158]. Li et al. demonstrated that K3 CPS could be combined with recombinant cholera toxin B subunit into a bioconjugate with significant protective effect [Citation159]. Rudenko et al. also recently reported CPS-based glycoconjugates with conserved K9 CPS fragments and several classical carrier proteins (bovine serum albumin (BSA), ovalbumin (OVA) and keyhole limpet hemocyanin (KLH)), which improved carbohydrate immunogenicity and mice survival upon bacterial challenge [Citation119].
Along with the capsules, several O-antigen structures have been identified for A. baumannii and methodologies for serotyping are currently under investigation [Citation160]. The O-antigen diversity and lack of understanding of highly expressed structures in clinical isolates remain a challenge for the development of OPS-based vaccines.
4.1.2. E. coli
E. coli produces a remarkable variety of CPS, 80 of which have been isolated [Citation161]. However, studies on the O-antigen distribution in some European countries and in the United States (US) suggest that 10 serotypes would cover the majority of UTI or bacteremia [Citation54,Citation108], which explains the focus on vaccines against these O-antigens in clinical development. ExPEC4V, a bioconjugate containing O-antigens from four E. coli serotypes (O1A, O2, O6A, and O25B, conjugated to exotoxin protein A (EPA) from P. aeruginosa), was shown to be well tolerated and immunogenic in a Phase II study [Citation58,Citation59]. However, ExPEC4V did not meet the expected endpoint in terms of UTI recurrence rate reduction, although a post hoc analysis showed an impact on UTI episodes regardless of serotype [Citation162]. The failure was attributed to the limited vaccine coverage (30–35% of serovars). Therefore, a 10–valent bioconjugate candidate vaccine (ExPEC10V) was tested in Phase I/II and a combination of 9 bioconjugates (ExPEC9V) was selected based on immunogenicity data, to advance in Phase III for prevention of invasive disease in older adults with a history of recurrent UTI.
The main challenge in the development of multivalent vaccines has been the low immunogenicity observed for some O-antigen serotypes. In particular, the glycans of serotypes O25A and O25B were demonstrated to be poor immunogens in healthy volunteers, which is unfortunate as serotype O25B has emerged as the leading cause of invasive multidrug-resistant ExPEC infections [Citation108]. Chorro et al. demonstrated that a linkage switch, from radial (as in bioconjugates) to lattice-like, in an elongated O25B PS glycoconjugate to CRM197 provided superior immunogenicity in mice. The conjugate was also shown to protect the animals from a lethal challenge with invasive MDR ST131 E. coli and to induce a robust functional antibody response in immunized nonhuman primates [Citation108]. In this context, a set of oligosaccharides from O25B serotype differing in RU frameshift and length were chemically synthesized and conjugated to CRM197 [Citation130]. Among them, three conjugates of glycan antigens induced antibody titers comparable to a conventional conjugate and enabling identification of relevant PS glycoepitopes.
Cell-free extracts of nonpathogenic E. coli were used for PglB mediated production of a bioconjugate vaccine candidate against two of the most prevalent ETEC O serogroups, O148 and O78. The two bioconjugates stimulated in mice induction of IgG antibodies with bactericidal activity against the cognate pathogens. Also, the bioconjugate containing O148 O-PS reduced ETEC colonization in mice by oral administration providing evidence of vaccine-induced mucosal protection [Citation131].
The glycosylation pattern of the protein SslE or YghJ, a metalloprotease known as potential E. coli vaccine candidate [Citation163], has been shown to occur in numerous ETEC strains has been shown to be recognized by patients infected with the pathogen suggesting potential use of glycopeptides as vaccine antigens. Glycopeptide [Citation164]-specific antibodies have been also identified in patients with UTI [Citation165].
4.1.3. E. faecalis and E. faecium
After the initial discovery of an LTA-like structure composed of α-Glc-(1,2)-α-Glc-(1,2)-glycerol-3-phosphate, different classes of polysaccharides have been isolated from E. faecalis [Citation166,Citation167]. Based on the analysis of the biosynthetic CPS locus, four serotypes A-D have been established. A recent analysis of clinical isolates indicated that most pathogenic strains belong to serotype C, although another study pointed at a broader serotype diversity [Citation168]. Early studies showed that purified CPS depleted the OPK activity of immune rabbit sera, and elicited high titers of antibodies in rabbit mediating OPK of bacteria [Citation166,Citation169]. Diheteroglycan (DHG) () has been recently characterized as an immunogenic component of CPS-C and -D, and a target to generate antibodies with OPK activity [Citation170]. An anti-DHG opsonic mAb was isolated and demonstrated to have efficacy in passive immunotherapy [Citation121]. DHG-SagA or DHG-PpiC proved to be valid glycoconjugate vaccines against several enterococcal species, with SagA and PpiC (E. faecium proteins) having a dual role as antigen and carrier [Citation121,Citation122]. Further studies are needed to inform on the best glycoconjugate design for maximal immunogenicity while maintaining control over the production. In that direction, synthetic oligomers mimicking DHG from E. faecalis type 2 conjugated to BSA have recently been evaluated in mice [Citation123]. Interestingly, cell wall-associated antigens of E. faecium U0317 were recognized by anti-DHG antibodies [Citation171].
By contrast, E. faecium CPS are far less known and though putative CPS biosynthetic genes have been predicted [Citation172,Citation173], primarily teichoic acids have been structurally elucidated [Citation166,Citation174]. Four distinct extracellular PS from E. faecium were isolated by Kodali et al. and conjugated to CRM197 for rabbit immunization. Of these vaccines, CRM197-Pf2, a conjugate bearing altruronic acid-containing heteroglycans, proved to be the most effective. Anti-Pf2-CRM197 antibodies had strong OPK activity and significantly reduced bacterial loads in mouse liver and kidney tissue. Altruronic acid, a glucuronic acid derivative, is a rare carbohydrate that can be found in the LPS or CPS of other pathogens, and could become part of multivalent formulations [Citation142].
4.1.4. K. pneumoniae
Among the most studied virulence factors for K. pneumoniae are CPS, or K antigens, 79 serotypes of which have been identified to date, with only 25 representing about 70% of clinical isolates [Citation100,Citation175]. A 24–valent PS K-antigen vaccine (administered in addition to 8 P. aeruginosa O-antigens conjugated to EPA as carrier protein) initially moved forward through multiple clinical trials demonstrating safety, immunogenicity and opsonic activity [Citation176–178]. However, the vaccine was never authorized on the market because of the advanced formulation needed for its development [Citation100]. Because the K1 and K2 serotypes () are responsible for an important part of hypervirulent infections, glycoconjugate vaccines targeting these two serotypes have been explored. In a recent study [Citation124], a glycoengineered E. coli strain was used to produce a bioconjugate vaccine expressing K1 and K2 antigens, which afforded protection to mice against a lethal challenge with two K. pneumoniae strains. Another promising example in preclinical studies is a synthetic K2 CPS conjugated with diphtheria toxin (DT) that induced the production of specific capsular antibodies in mice [Citation125].
As opposed to CPS, which are highly variable, K. pneumoniae O-antigens could represent alternative targets thanks to their limited structural range. Four serotypes (O1, O2, O3 and O5) are responsible for around 80% of clinically relevant K. pneumoniae strains [Citation179]. In mid-2021, a tetravalent bioconjugate candidate vaccine (Kleb4V), produced by LimmaTech Biologics AG in collaboration with GSK, completed clinical Phase I/II and proved to be safe and immunogenic in humans. The tetravalent bioconjugate vaccine covers OPS of the most predominant K. pneumoniae serotypes (NCT04959344) [Citation32]. Recently, Hegerle et al. combined two P. aeruginosa flagellins (FlaA, FlaB) to O1, O2, O3, O5 Klebsiella O-serotypes to obtain a 4–valent chemical conjugate that induced antibody titers against all antigens in rabbits [Citation128]. Similarly, Cross et al. reported functional in vitro and in vivo activity of antibodies produced by a 12–valent vaccine comprised of four K. pneumoniae (O1, O2, O3 and O5) and eight P. aeruginosa core and O-polysaccharides (COPS), and interesting protein antigens such as MrkA (K. pneumoniae virulence factor), combined through the MAPS platform. The vaccine induced a robust immune response against the carbohydrate antigens of both bacteria as well as against the proteins [Citation129]. Synthetic glycans resembling the PS coating K. pneumoniae surface have been also targeted by Idorsia (former Vaxxilon) to develop a multivalent semisynthetic conjugate vaccine (Patent no. US20230122752A1).
4.1.5. P. aeruginosa
For P. aeruginosa, more than 20 different O-antigen structures can be distinguished, but only 11 of these are expressed in the majority of pathogenic serotypes. LPS-based vaccines against P. aeruginosa, initially included 7 (Pseudogen) and subsequently 16 different serotypes, and these have been tested in the clinics since the 1970s, ultimately failing to show efficacy [Citation180–184]. In one study in patients with cystic fibrosis (CF), Pseudogen exacerbated the infection possibly because of the presence of lipid A [Citation185]. Increasing the coverage of these LPS-based vaccines did not provide significant improvements in terms of reduction of bacterial colonization [Citation114,Citation186–188].
A conjugate vaccine, Aerugen, composed of EPA and O-antigens from eight serotypes, was proven safe and immunogenic in diverse populations, including plasma donors, bone marrow transplant and non-colonized CF patients [Citation189–191]. The vaccine was also shown to induce opsonophagocytic antibodies with a three year persistency in a small cohort of non-colonized CF patients [Citation192]. However, in a larger trial in CF patients, Aerugen did not show efficacy compared to the placebo and it was the last carbohydrate-based vaccine against P. aeruginosa reported in the clinics [Citation193]. Despite these failures, O-antigens remain promising targets and new approaches have been recently investigated at a preclinical level, including conjugation to poly(lactic-co-glycolic acid) (PGLA)- [Citation126] or gold-based (AuNP) [Citation127] nanoparticles.
P. aeruginosa also produces a surface PS antigen termed A-band polysaccharide (A-PS) (), that can be attached to the LPS, which is then referred to as A-band LPS, in contrast to the LPS O-antigens, which are sometimes termed B-band LPS. Notably, A-band LPS is found to be conserved in clinical isolates, while serotype-specific B-band LPS disappears over time. A-PS is composed of a rhamnan RU, and several other non-rhamnose components that remain to be confirmed. Preliminary studies, however, determined the rhamnan chain to be non-immunogenic. Recently, Cairns et al. established that the A-PS is capped with a 3-O-methyl-D-rhamnose pentasaccharide linked through a methyl mannose unit to a neutral mannose trisaccharide. Further study on an antibody that was broadly cross-reactive to LPS hinted at the potential of A-PS as immunogen for vaccine or therapeutic development against P. aeruginosa [Citation194]. The same group reported the synthesis of the terminal methylated oligosaccharide and confirmed the immunogenicity of the A-PS tip structure through conjugation to human serum albumin (HSA) [Citation133].
4.1.6. S. aureus
CPS 5 and 8 (CP5 and CP8, respectively) () are the most prevalent capsular serotypes in clinical isolates of S. aureus and are associated with approximately 80% of infections [Citation195]. CP5 and CP8 conjugates have been evaluated as vaccine candidates since the 1990s, most notably in Nabi’s StaphVax (CP5/CP8 glycoconjugates to EPA), and more recently in Pfizer’s SA4Ag (containing CP5/8 glycoconjugates to CRM197, with two additional protein antigens) as well as in GSK’s trivalent vaccines (composed of CP5/8–tetanus toxoid (TT) conjugates) [Citation55,Citation196]. However, no vaccine has been licensed so far.
While the unadjuvanted CP5 and CP8 EPA conjugates initially showed efficacy [Citation197,Citation198], no benefit compared to placebo was shown in further clinical studies involving end-stage renal disease patients as target population [Citation199]. In the GSK vaccine, the two CP5–and 8–TT conjugates were mixed with the detoxified α-toxin (Hla H35L) and clumping factor A (ClfA) and tested with and without adjuvant AS03B in a Phase I study in healthy volunteers. The vaccine was safe and induced a strong humoral response [Citation200]. CP5–and CP8–CRM197 conjugates combined with ClfA and manganese transporter C, without adjuvant, were well tolerated and immunogenic in a Phase I study conducted by Pfizer [Citation201,Citation202]. Challenges associated with the development of these vaccines include the lability of the linker used for conjugation, specifically considering the disulfide chemistry conjugation exploited by Nabi’s approach [Citation196,Citation203]. More generally, the lack of established correlates of protection with studies in humans and the complexity of staphylococcal pathogenesis have hampered the success of vaccination [Citation204,Citation205].
Preclinical research is still ongoing on CP5 and CP8 [Citation117], through emerging technologies including bioconjugate vaccines, MAPS and semi-synthetic glycoconjugates [Citation116] which could improve the manufacturing and stability issues associated with classical conjugates.
4.1.7. Conserved LPS epitopes
An LPS mimic of a conserved core tetrasaccharide, Hep2Kdo2 (), was synthesized and evaluated as protective antigen against multiple Gram-negative pathogens. Sera raised against the Hep2Kdo2 glycoconjugate were cross-reactive to carbohydrate extracts from various bacteria, including P. aeruginosa. Interestingly, this vaccine proved efficacious against N. meningitidis and E. coli when administered with an inhibitor of the CPS transporter to allow access to the inner LPS core. Besides solving the lack of antigen exposure, this ‘vaccination + block’ approach could bring a level of control and minimize off-target damage to other commensal bacteria, as either element is not sufficient for bacterial killing [Citation132]. However, this method and other similar approaches could prove too complicated for clinical trials.
Epitope mapping of a mAb targeting the LPS inner core revealed binding to a heavily phosphorylated heptose, characteristic of Hep2Kdo2 in P. aeruginosa strains. The mAb was suggested to be serotype-independent but exclusive to P. aeruginosa over other Gram-negative bacteria [Citation206]. The strain specificity of conserved antigens could prove useful in limiting negative effects on the normal flora, at the cost of reduced antibody binding breadth against other Gram-negative pathogens.
4.2. Teichoic acids
Teichoic acids (TA) are important adhesive macromolecules from Gram-positive bacteria. They are phosphodiester-based glycopolymers, built up from a ribitol phosphate or glycerolphosphate backbone and include the membrane-anchored lipoteichoic acid (LTA) and the peptidoglycan-bound wall teichoic acid (WTA) (). Both polymers are associated with biofilm formation and immune evasion [Citation151]. Because TA antigens are highly expressed, easily accessible, and with limited structural variation, passive immunization against TA could be a particularly suitable strategy against S. aureus [Citation151], especially for immunocompromised patients for whom active immunization could prove too taxing. Pagibaximab, a chimeric monoclonal antibody against LTA, was developed by Biosynexus Inc. to prevent sepsis in neonates, unfortunately to no avail (NCT00646399) [Citation61].
Besides passive immunotherapy, TA remain interesting targets for vaccine development [Citation207], and various efforts have been made toward their synthesis and immunological evaluation [Citation148,Citation208–210]. Importantly, cross-reactivity of LTA was reported, pointing out LTA as a potential single vaccine antigen to target multiple Gram-positive pathogens, including S. aureus, E. faecalis and E. faecium [Citation144,Citation211].
Despite their inherent low immunogenicity as stand-alone antigens, it was reported that mouse immunization with purified PNAG and/or TA PS could have an inhibitory effect on biofilm formation in S. aureus [Citation143]. Along with DHG, LTA is responsible for the strain diversity of E. faecalis. LTA was shown to be a viable vaccine candidate for CPS-A and -B serotypes, which lack DHG that masks LTA in CPS-C and -D strains (see previous section) and prevent opsonization by anti-LTA antibodies [Citation170]. The genetically similar pathogen E. faecium also expresses TA, several glycoforms of which have already been successfully synthesized [Citation148,Citation210]. Pf3 is a glucosylated LTA and one of the four E. faecium surface PS identified by Kodali and colleagues. Nonlipidated Pf3 was conjugated to CRM197 and used for rabbit immunization, where the raised antibodies showed significantly enhanced OPK activity compared to those raised with unconjugated and lipidated Pf3 [Citation142]. Zhou et al. conjugated a synthetic E. faecium U0317 WTA monomer to KLH for mouse immunization, eliciting high IgG titers against the conjugate. However the raised sera were not tested against naturally occurring WTA and their functionality was not investigated [Citation145]. Finally, an LTA-mimicking peptide was recently reported to protect mice from S. aureus infection, which could represent another clever use of carbohydrate antigens [Citation212].
4.3. Pseudomonas extracellular or exopolysaccharides
Bacterial extracellular polymeric substances (EPS) are biofilm components and include extracellular or exopolysaccharides. These PS represent a major proportion of the EPS matrix for both Gram-positive and -negative bacteria and are involved in most biofilm-related functions [Citation213]. P. aeruginosa can produce three different exopolysaccharides, alginate (or mucoid exopolysaccharide, MEP), Psl and Pel, each of which is associated with different biofilm development stages () [Citation213].
Alginate is a promising immunogen that could be used to produce opsonizing antibodies [Citation193], but it failed in Phase I due to its poor immunogenicity as a stand-alone antigen. However, these vaccine preparations contained no protein component nor adjuvant which could have amplified the immune response. Preclinical research and immunological evaluation of alginate is still ongoing [Citation134–137], with several NP approaches in development. PGLA [Citation135], solid lipid nanoparticles (SLN) [Citation136] and OMV [Citation137] conjugates decorated with P. aeruginosa-extracted alginate are being developed. Recently, Zhang et al. synthesized alginate fragments up to the 24–mer and assessed their immunogenicity in mice through HSA conjugates [Citation134]. Interestingly, the data seems to indicate that an alginate tetrasaccharide can serve as an optimal antigen to elicit a sufficient immune response. Passive immunization against alginate is currently also being explored. Aridis Pharmaceutical’s AR-105 (Aerucin), a human IgG1 mAb targeting P. aeruginosa alginate, with a broad recognition of several clinical isolates, demonstrated safety in a Phase I clinical trial in 16 healthy volunteers [Citation63] and recently completed a Phase II study in ventilator-associated pneumonia patients (NCT03027609), though no report has yet been published.
Pel and Psl are the two other exopolysaccharides produced by P. aeruginosa [Citation214,Citation215]. Presence of Psl has been ascertained in 76% of analyzed clinical isolates, and its expression has been shown to favor persistent infection. Psl is involved in the formation and maintenance of biofilms and recently has been shown to protect P. aeruginosa from host defenses within the CF lung by inhibiting opsonization and phagocyte killing, prior to the conversion to the mucoid phenotype [Citation216]. Anti-Psl mAb have demonstrated to be protective in various animal models with an OPK ability against several bacterial strains [Citation217]. One of these mAb, MEDI3902, binding in a bivalent mode to Psl and PcrV, a protein involved in host cell cytotoxicity, was initially shown safe in a dose escalation Phase I study in healthy subjects [Citation218]. It failed however to reduce P. aeruginosa nosocomial pneumonia incidence in Pseudomonas-colonized mechanically ventilated subjects [Citation62]. Recently, three mAb targeting three distinct Psl epitopes exhibited stratified binding in mature in vitro biofilms and bound Psl within the context of a chronic biofilm infection. The mAb also inhibited biofilm formation to a varying extent but all favored biomass reduction in the presence of neutrophils [Citation219].
Polyhydroxyalkanoates (PHA) are natural polymeric intracellular inclusions often produced by bacteria to store energy and materials. These NP can be engineered to express vaccine antigens at their surface, a feature recently harnessed by Rehm’s group for a particulate vaccine against P. aeruginosa [Citation138,Citation139]. While only protein antigens were expressed at the surface of PHA particles, the authors observed that by deliberately co-purifying PHA with other host cell proteins and Psl, they could broaden the immune response induced by the PHA vaccine. Indeed, a significant anti-Psl response was shown in the sera from PHA-immunized mice [Citation138].
4.4. PNAG
Poly-N-acetyl glucosamine (PNAG), also known as the polysaccharide intercellular adhesin (PIA), capsular polysaccharide/adhesin (PS/A) or slime-associated antigen (SAA), is a β-(1–6)-linked surface polymer, sometimes categorized as a CPS or as an exopolysaccharide (). PNAG plays an essential role in the biofilm production and immune evasion mechanisms of different bacterial species and has been identified as a virulence factor in most ESKAPE pathogens. This prevalence makes for a particularly interesting protective antigen with a broad vaccination potential [Citation213,Citation220]. The deacetylated form of PNAG has been shown to induce protective antibodies against S. aureus [Citation221,Citation222], as opposed to the acetylated form that generates poorly opsonic unprotective antibodies [Citation223]. A synthetic dPNAG composed of nine monosaccharides, conjugated to staphylococcal Hla H35L-induced antibodies capable of reducing staphylococcal skin abscesses, pneumonia, and nasal colonization in mice [Citation224]. A synthetic PNAG pentamer TT-conjugate vaccine candidate (AV0328) was tested to offer protection against S. pneumoniae, methicillin-resistant S. aureus and N. meningitidis, and progressed in Phase I and II (NCT02853617) [Citation225]. The comparability of antibodies raised against synthetic nonamer and pentamer-GlcN-TT conjugates and X-ray studies of a highly opsonic monoclonal antibody (F598) backed the hypothesis that a pentasaccharide is the shortest repeat for effective immunization against PNAG. The data also suggested that a single acetyl group is required for binding, explaining how protective antibodies are able to bind both highly and poorly acetylated PNAG forms [Citation226]. Alopexx reported the in vitro OPK activity of AV0328–derived antibodies against a wide range of pathogens, including E. coli, A. baumannii, and K. pneumoniae. Notably, rabbit antibodies induced against the synthetic oligosaccharide conjugated to TT induced complement-mediated killing of A. baumannii S1, a high PNAG-producing strain, but not its PNAG-negative mutant [Citation227]. Immunization significantly reduced post infection levels of A. baumannii in the lungs and blood, demonstrating that the PNAG conjugate could prevent pneumonia and pathogen caused bacteremia. Recently, different modalities to present PNAG have been explored. GlycoOMV, where PNAG was expressed in OMV, were recently described by Stevenson et al. to increase OPK activity against S. aureus in comparison to the pentameric GlcN-TT conjugate, while avoiding the chemical conjugation step [Citation95].
Active and passive immunization against PNAG has been described by Hülsdünker et al., who observed a decrease in neutrophil activation and in the severity of graft-versus-host disease in mice [Citation60]. Anti-PNAG mAb F598 has completed Phase I clinical studies, and combination with other anti-PNAG mAb, such as TG10 may have synergistic effects.
4.5. Prokaryotic nonulosonic acids
Nonulosonic acids (NulO) are a superfamily of carbohydrates which includes the well-known sialic acids and several other structures unique to prokaryotes that decorate cell surface glycans (e.g. CPS and LPS) and glycoproteins (e.g. flagellin, pili proteins). Much like hypersialylated cancer cells, prokaryotic NulO help pathogens evade the host’s immune defenses, but also have roles in bacterial motility and biofilm formation [Citation228]. Targeting selected repeats rather than whole PS can be more effective [Citation229], and these signature glycans can therefore become privileged antigens.
Lee et al. recently isolated pseudaminic acid (Pse)-containing oligosaccharides from the exopolysaccharide of A. baumannii through phage-assisted digestion. A. baumannii strain 54,149 exopolysaccharide was essentially depolymerized to two RU and conjugated to CRM197. Rabbit antibodies raised against the glycoconjugate displayed in vitro bactericidal activity and identified Pse as a strong immunogen and main epitope [Citation140]. Subsequently, Wei et al. synthesized Pse and evaluated the immunogenicity of synthetic Pse-based conjugates with different glycosylation degrees. All Pse-vaccines provided protection against a lethal challenge with A. baumannii, with the conjugate bearing 8 Pse units affording 100% survival (as compared to the 80% of survival afforded by the lower and higher glycosylated CRM197, carrying 4 or 14 Pse units respectively) [Citation141]. Acinetaminic acid is another prokaryotic NulO, isolated from A. baumannii K13 and K73 CPS, which differ in their forms [Citation230].
Enterococcal glycans can also be decorated with NulO. Of the four PS isolated from E. faecium by Kodali et al., Pf4, a legionaminic acid-containing heteroglycan, conjugated to CRM197 generated opsonic antibodies and reduced bacterial loads in mouse tissue. While the immunological impact of legionaminic acid in Pf4 is unknown, a similar branched pentamer has been shown to form the core repeat of O-antigen from C. tuicensis [Citation142]. Legionaminic acid can also be found in the capsules of E. coli and P. aeruginosa [Citation228].
These studies highlight how targeting single immunodominant carbohydrate moieties or designed repeats could be effective and lead to more rationally designed multivalent cross-reactive formulations where well-defined synthetic oligosaccharides would play a central role. Potential cross-reactivity with mammalian sialic acids should however be taken into consideration.
Box 1. Key AMR pathogens and associated challenges for vaccine development.
Escherichia coli (WHO critical priority [Citation4], CDC urgent/serious threat [Citation101])
E. coli is a Gram-negative bacterium commonly found in the human gut. Typically, strains are classified into commensal microbiota, enterovirulent or extraintestinal pathogenic E. coli (ExPEC) on the basis of their genetic features and clinical outcomes [Citation102]. Strains causing gastroenteritis are in turn subdivided in enterotoxigenic (ETEC), enteropathogenic (EPEC), enterohemorrhagic (EHEC), enteroaggregative (EAEC), enteroinvasive (EIEC), and lastly diffusely adhering E. coli (DAEC). ExPEC can cause severe (extra)intestinal infections and diseases, urinary tract infections (UTI), bacteremia and meningitis. ETEC is a major cause of traveler’s diarrhea and death from diarrheal disease worldwide, especially in infants and young children [Citation103]. Antibiotic resistance has become a major concern in the treatment of infections, and the production of an effective E. coli vaccine is challenging due to i) the heterogeneity of E. coli strains, ii) the high probability of side effects on the intestinal flora, iii) the dependence of the disease to the expression of multiple virulence factors [Citation104], especially since an effective vaccine should provide protection against the bacteria at different stages of pathogenesis [Citation105–107]. Most carbohydrate-based vaccines under development are targeting urinary and invasive infections due to the well-known capacity of anti O-antigen antibodies to induce bacterial killing in animal models [Citation108].
Staphylococcus aureus (WHO high priority [Citation4], CDC serious threat [Citation101])
S. aureus is a commensal Gram-positive bacterium increasingly resistant to the first-line antibiotic vancomycin [Citation109]. It is the most common microbial cause of nosocomial and community-acquired skin and soft tissue infections (SSTI) [Citation110], and of surgical site infections (SSI) [Citation111]. Around 50% of all staphylococcal infections are estimated to be methicillin-resistant [Citation112]. Given the high infection frequency, contagiousness and the prevalence of resistant strains, SSTI highly contribute to AMR spread [Citation2]. Although a few vaccines are in the pipeline, a successful development will likely depend on a better understanding of pathogen-host interactions.
Klebsiella pneumoniae
K. pneumoniae is an opportunistic Gram-negative bacterium found in the human mouth and gut. K. pneumoniae is associated with hospital-acquired persistent UTI, liver abscess, SSTI, pneumonia and sepsis, particularly affecting the elderly and immunocompromised patients. Infections are also widespread in communal settings. Resistance to cephalosporins and last-line carbapenems through beta-lactamase production generates invasive infections associated with high mortality [Citation101]. K. pneumoniae is considered a reservoir and propagator of AMR-related genes [Citation2]. K. pneumoniae is also a leading cause of neonatal sepsis in low and middle-income countries which has been associated with spread of multi-resistant lineages [Citation113].
Acinetobacter baumannii (WHO critical priority [Citation4], CDC urgent threat [Citation101])
A. baumannii is a commensal Gram-negative bacterium commonly found in hospitals, where it causes pneumonia, SSI, UTI, as well as bloodstream and wound infections. A. baumannii is intrinsically resistant to antibiotics and can acquire the ability to produce carbapenemases through gene transfer. Carbapenem-resistant A. baumannii ranks as the most critical pathogen in the WHO priority list for research and development of new antibiotics and as a serious threat for the CDC [Citation4,Citation101]. It is however not considered a strong candidate for vaccine development, due to its low incidence and difficulties to identify key target populations, therefore developing alternative strategies such as passive immunization is currently recommended [Citation112].
Pseudomonas aeruginosa (WHO critical priority [Citation4] CDC serious threat [Citation101])
P. aeruginosa is an opportunistic Gram-negative bacterium causing life-threatening infections in immunocompromised individuals and patients with cystic fibrosis (CF). It is increasingly recognized as a nosocomial pathogen in the elderly and in combat-related wounds, suggesting an appropriate target population for vaccination [Citation114]. P. aeruginosa exhibits most of the known resistance mechanisms to antibiotics, that are both intrinsic chromosomally encoded or originate from genetically imported plasmids. Some strains are resistant to more than three classes of antibiotics and pan-drug resistant strains have been reported [Citation115]. P. aeruginosa is also able to form a biofilm in the lungs of infected patients thus impeding the access of antibiotics to bacterial cells. Despite years of academic and industrial research efforts, no commercial vaccine is available, and no potential candidates are currently in clinical trials [Citation2,Citation112].
Enterococcus faecium (CDC serious threat [Citation101])
E. faecium is a Gram-positive commensal bacterium commonly found in the gastrointestinal tract and causing nosocomial infections, such as UTI, endocarditis and meningitis. Immunocompromised individuals and patients in intensive care units are particularly at risk. Up to 30% of infections are currently resistant to vancomycin, and additional resistance is on the rise [Citation101]. Due to the low incidence, morbidity and mortality associated with enteroccocal infections, vaccine development is currently not recommended and other approaches are explored [Citation112]. Infections from E. faecium and faecalis are often undistinguishable.
5. Conclusion
Glycoconjugate vaccines are widely recognized tools for preventing bacterial infections. This class of vaccines has demonstrated the ability to generate opsonic or bactericidal antibodies in humans, effectively thwarting invasive infections such as pneumoniae and impacting bacterial carriage. Consequently, carbohydrate-based vaccines could play a crucial role in combating infections caused by the so-called ESKAPE pathogens, which are primary drivers of AMR. In addition to traditional methods, emerging technologies such as chemically synthesized carbohydrates, bioconjugation, glyconanoparticles/GMMA and MAPS are enabling the development of purpose-designed novel glycoconjugate vaccines.
6. Expert opinion
The global AMR burden seems primarily associated with the ESKAPE pathogens. As such, identifying syndromes where future vaccines can be clinically tested will be key to successfully develop a preventive strategy against AMR. Lower respiratory and thorax infections, bloodstream infections, and intra-abdominal infections have been estimated to account for almost 80% of deaths attributable to AMR pathogens in 2019, with lower respiratory infections alone accounting for more than 400,000 attributable deaths and 1.5 million associated deaths [Citation1]. These infections are often associated with large consumptions of antibiotics driving the emergence of resistant strains. Nosocomial infections have been a significant source of AMR. Central line-associated bloodstream infections (CLABSI), ventilatory-associated infection, catheter-associated UTI (CAUTI) and surgical site infections (SSI) represent the most relevant syndromes [Citation231], with four pathogens accounting as primary causative agents (S. aureus, E. coli, P. aeruginosa, and K. pneumoniae) () [Citation232]. This suggests that developing tools to prevent infections caused by these targets will not only help alleviate these diseases but also fight the development of AMR.
Figure 5. Incidence of AMR pathogens in adult healthcare-associated infections (US data 2015–2017). Among the ESKAPE pathogens, E. coli, S. aureus, klebsiella spp. and P. aeruginosa emerge as the ones with higher incidence across different syndromes. Vaccines against these pathogens remains a priority. Figure adapted from reference [Citation232]. Abbreviations: CAUTI, catheter-associated urinary tract infections; CLABSI, central line-associated bloodstream infections; PVAP, possible ventilator-associated pneumoniae; SSI, surgical site infections.
![Figure 5. Incidence of AMR pathogens in adult healthcare-associated infections (US data 2015–2017). Among the ESKAPE pathogens, E. coli, S. aureus, klebsiella spp. and P. aeruginosa emerge as the ones with higher incidence across different syndromes. Vaccines against these pathogens remains a priority. Figure adapted from reference [Citation232]. Abbreviations: CAUTI, catheter-associated urinary tract infections; CLABSI, central line-associated bloodstream infections; PVAP, possible ventilator-associated pneumoniae; SSI, surgical site infections.](/cms/asset/3c6f1804-c9f7-4e15-94c3-c2961299ec83/ierv_a_2274955_f0005_oc.jpg)
rRemarkably, AMR is not exclusively linked to nosocomial infections. UTI accounts for 150,000 episodes globally per year and is responsible for 11% of antibiotic prescriptions. The consequence of community-acquired infections should not be underestimated: for example, E. coli bacteremia [Citation233] seems to be primarily linked to UTI in both nosocomial and community settings. Therefore, impacting community-acquired infections could also reduce the burden of bacteremia.
It is worth underscoring that E coli and K. pneumoniae are also major causes of neonatal sepsis and a vaccine has been advocated by the WHO [Citation234–236].
Carbohydrate-based vaccines have the potential to play a relevant role to counteract AMR. However, in order to design effective glycoconjugate vaccines against AMR pathogens some challenges will need to be addressed.
Considering that hospital-acquired infections associated with AMR are often polymicrobial, complex multivalent formulations will be needed to ensure disease prevention and reduce the AMR burden. In some of these infections (e.g. CAUTI or CF), therapy is not very effective because of the formation of biofilms in which pathogens are hidden and poorly accessible to therapeutics as well as antibodies. Targeting biofilm-associated glycans (e.g. PNAG, Psl) and proteins along with disrupting biofilm agents could be key to succeeding in the development of novel therapeutic approaches.
Also, currently approved glycoconjugate vaccines are all made from CPS, while it remains to be seen whether OPS can be successful. For example it has been pointed out that the capsule might obstruct O-antigen specific antibody binding in E. coli [Citation237] and K. pneumoniae [Citation238]. In some cases, as in bronchiectasis patients, high titers of IgG2 specific for the O-antigen resulted in impaired Pseudomonas serum-mediated killing [Citation239], which could explain the inconsistent results of LPS-based approaches thus far explored for P. aeruginosa vaccines. Considering that patients with P. aeruginosa-associated bloodstream infections presented non-protective antibodies against Psl, boosting the immune response against this PS might be challenging [Citation240]. For other biofilm-associated PS like PNAG, which are ubiquitous among different species, safety concerns need to be addressed. The difficult path for a S. aureus vaccines well exemplifies challenges associated with AMR vaccine development as various glycoconjugate formulations have been tested in clinical trials with no success. While the chemical features of some conjugates tested in early clinical trials could not ensure adequate stability to the vaccine [Citation203], challenges are also associated with the existence of multiple evasion mechanisms. Consequently, current vaccine formulations under development are based on either non-capsular carbohydrate antigens (e.g. teichoic acids) or on protein antigens combined to glycoconjugates. Also, training the immune system for the production of specific antibody subclasses could be relevant for an appropriate immune response against S. aureus [Citation241].
Glycoconjugate vaccines typically elicit suboptimal immune response in individuals with underlying comorbidities, immunocompromised and aging populations, who are often exposed to antibiotic use [Citation11,Citation12]. Current vaccination schedules include several booster immunizations [Citation242], without reaching the glycoconjugates’ full protection potential [Citation11] and fail to quickly mount a strong immune response [Citation243]. In today’s AMR context, identifying novel glycoconjugate designs combined with potent adjuvants to enhance immunogenicity is pivotal. For instance, potent single-dose vaccines are highly desirable to counteract nosocomial infections and expand access where supply issues could occur.
A more profound understanding of immunological mechanisms behind glycoconjugate vaccines would be beneficial in this regard [Citation244]. Carrier-induced epitope suppression (CIES) is one of such immune phenomena which has not yet been fully unraveled and is directly relevant to the choice of carrier for safe and efficient conjugate vaccines. CIES involves the dampening of the immune response against PS epitopes by prior or simultaneous immunization with the same protein carrier as used in the glycoconjugate, and has been observed in several conjugate combination vaccines [Citation244,Citation245]. CIES has been proposed to be caused by the clonal expansion of B cells specific for carrier protein epitopes following intramolecular antigenic competition. Nonetheless, clinical data assessment [Citation246] has determined that CIES is not the only underlying interference mechanism and is not observed for all regimens. As additional antigens are added to routine vaccinations, further research is required to confirm the impact of CIES and understand the patterns involved in either the enhancement or hindrance of the immune response [Citation244,Citation245].
All these challenges call for technologies enabling access to complex multi-antigen and even multi-pathogen nanosized vaccines. In this regard, the development of innovative platforms such as MAPS, NP and GMMA, as well as advances in glycoengineering is likely critical. These emerging technologies are currently under evaluation at the clinical level and the coming years will be an exciting time to learn which ones will be validated by licensure of new vaccines.
Article highlights
Developing vaccines against ESKAPE pathogens is a critical priority, as these pathogens are major contributors to AMR, posing a severe threat to public health.
Carbohydrate-based vaccines harnessing bacterial glycans could be instrumental in the fight against drug-resistant pathogens.
A deeper understanding of glycoimmunology is crucial and would help comprehend how glycoconjugates work, paving the way for more effective vaccine development.
The development of effective bacterial vaccines faces multiple challenges, including the need for new technologies and analytical tools to ensure vaccine quality and deliverability.
The challenging identification of target populations to assess clinical efficacy and impact on AMR is calling for new clinical design and regulatory approaches.
Declaration of interests
C Sorieul is a PhD student at Leiden University and a former employee of GSK in the context of the industrial EU program Horizon 2020 PAVax, grant no 861194. M Dolce is a PhD student and participated in a post graduate studentship program at GSK in collaboration with the University of Siena. M Rosaria Romano and R Adamo are employees of the GSK group of companies. The authors have no other relevant affiliations or financial involvement with any organization or entity with a financial interest in or financial conflict with the subject matter or material discussed in the manuscript apart from those disclosed.
Reviewer disclosures
Peer reviewers on this manuscript have no relevant financial or other relationships to disclose.
Author contributions
All authors have substantially contributed to the conception and design of the review article and interpreting the relevant literature, and have been involved in writing the review article or revised it for intellectual content.
Acknowledgments
to were created with BioRender.com. Carbohydrate structures from were generated with the CSDB/SNFG structure editor.
Additional information
Funding
References
- Murray CJL, Ikuta KS, Sharara F, et al. Global burden of bacterial antimicrobial resistance in 2019: a systematic analysis. Lancet. 2022;399(10325):629–655. doi: 10.1016/S0140-6736(21)02724-0
- Micoli F, Bagnoli F, Rappuoli R, et al. The role of vaccines in combatting antimicrobial resistance. Nat Rev Microbiol. 2021 May;19(5):287–302. doi: 10.1038/s41579-020-00506-3
- RAND E. How drug resistant infections are undermining modern medicine and why more research is needed now. London: Wellcome Trust; 2021.
- Tacconelli E, Carrara E, Savoldi A, et al. Discovery, research, and development of new antibiotics: the WHO priority list of antibiotic-resistant bacteria and tuberculosis. Lancet Infect Dis. 2018;18(3):318–327. doi: 10.1016/S1473-3099(17)30753-3
- Vekemans J, Hasso-Agopsowicz M, Kang G, et al. Leveraging vaccines to reduce antibiotic use and prevent antimicrobial resistance: a World health organization action framework. Clin Infect Dis. 1017 [2021 Aug 16];73(4):e1011–e. doi: 10.1093/cid/ciab062
- Ladhani SN, Collins S, Djennad A, et al. Rapid increase in non-vaccine serotypes causing invasive pneumococcal disease in England and wales, 2000-17: a prospective national observational cohort study. Lancet Infect Dis. 2018;18(4):441–451. doi: 10.1016/S1473-3099(18)30052-5
- Chandler T, Furmanek S, Carrico R, et al. 23-Valent Pneumococcal Polysaccharide Vaccination Does Not Prevent Community-Acquired Pneumonia Hospitalizations Due to Vaccine-Type Streptococcus pneumoniae. Microorganisms. 2022 Mar 4;10(3):560. doi: 10.3390/microorganisms10030560
- Hoelzer K, Bielke L, Blake DP, et al. Vaccines as alternatives to antibiotics for food producing animals. Part 1: challenges and needs. Vet Res. 2018 Jul 31;49(1):64. doi: 10.1186/s13567-018-0560-8
- Frost I, Sati H, Garcia-Vello P, et al. The role of bacterial vaccines in the fight against antimicrobial resistance: an analysis of the preclinical and clinical development pipeline. Lancet Microbe. 2022;4(2):e113–e125. doi: 10.1016/S2666-5247(22)00303-2
- Micoli F, Costantino P, Adamo R. Potential targets for next generation antimicrobial glycoconjugate vaccines. FEMS Microbiol Rev. 2018;42(3):388–423. doi: 10.1093/femsre/fuy011
- Rappuoli R. Glycoconjugate vaccines: Principles and mechanisms. Sci Transl Med. 2018 Aug 29;10(456). doi: 10.1126/scitranslmed.aat4615
- Stefanetti G, Borriello F, Richichi B, et al. Immunobiology of carbohydrates: implications for novel vaccine and adjuvant design against infectious diseases [review]. Front Cell Infect Microbiol. 2022 Jan 18;11:11.
- Luong P, Dube DH. Dismantling the bacterial glycocalyx: chemical tools to probe, perturb, and image bacterial glycans. Bioorg Med Chem. 2021 Jul 15;42:116268. doi: 10.1016/j.bmc.2021.116268
- Jha V, Janoff EN. Complementary role of CD4+ T cells in response to pneumococcal polysaccharide vaccines in humans. Vaccines (Basel). 2019 Feb 11;7(1):18. doi: 10.3390/vaccines7010018
- Vos Q, Lees A, Wu ZQ, et al. B-cell activation by T-cell-independent type 2 antigens as an integral part of the humoral immune response to pathogenic microorganisms. Immunol Rev. 2000 Aug;176:154–170.
- Craxton A, Magaletti D, Ryan EJ, et al. Macrophage- and dendritic cell–dependent regulation of human B-cell proliferation requires the TNF family ligand BAFF. Blood. 2003 Jun 1;101(11):4464–4471. doi: 10.1182/blood-2002-10-3123
- Khatun F, Toth I, Stephenson RJ. Immunology of carbohydrate-based vaccines. Adv Drug Deliv Rev. 2020;165-166:117–126. doi: 10.1016/j.addr.2020.04.006
- Snapper CM, Mond JJ. A model for induction of T cell-independent humoral immunity in response to polysaccharide antigens. J Immunol. 1996;157(6):2229–2233. doi: 10.4049/jimmunol.157.6.2229
- Pace D. Glycoconjugate vaccines.Expert Opin Biol Ther. 2013 Jan 1;13(1):11–33. doi: 10.1517/14712598.2012.725718
- Pishesha N, Harmand TJ, Ploegh HL. A guide to antigen processing and presentation. Nat Rev Immunol. 2022 Dec;22(12):751–764. doi: 10.1038/s41577-022-00707-2
- Richmond P, Kaczmarski E, Borrow R, et al. Meningococcal C polysaccharide vaccine induces immunologic hyporesponsiveness in adults that is overcome by meningococcal C conjugate vaccine. J Infect Dis. 2000 Feb;181(2):761–764. doi: 10.1086/315284
- Brynjolfsson SF, Henneken M, Bjarnarson SP, et al. Hyporesponsiveness following booster immunization with bacterial polysaccharides is caused by apoptosis of memory B cells. J Infect Dis. 2011;205(3):422–430. doi: 10.1093/infdis/jir750
- Bjarnarson SP, Benonisson H, Del Giudice G, et al. Pneumococcal polysaccharide abrogates conjugate-induced germinal center reaction and depletes antibody secreting cell pool, causing hyporesponsiveness. PLoS One. 2013;8(9):e72588. doi: 10.1371/journal.pone.0072588
- Zandvoort A, Timens W. The dual function of the splenic marginal zone: essential for initiation of anti-TI-2 responses but also vital in the general first-line defense against blood-borne antigens. Clin Exp Immunol. 2002 Oct;130(1):4–11. doi: 10.1046/j.1365-2249.2002.01953.x
- Siegrist CA, Aspinall R. B-cell responses to vaccination at the extremes of age. Nat Rev Immunol. 2009 Mar;9(3):185–194. doi: 10.1038/nri2508
- Sun L, Middleton DR, Wantuch PL, et al. Carbohydrates as T-cell antigens with implications in health and disease. Glycobiology. 2016 Oct;26(10):1029–1040. doi: 10.1093/glycob/cww062
- Avci FY, Li X, Tsuji M, et al. A mechanism for glycoconjugate vaccine activation of the adaptive immune system and its implications for vaccine design. Nat Med. 2011 Nov 20;17(12):1602–1609. doi: 10.1038/nm.2535
- Koj S, Lugowski C, Niedziela T. In-cell depolymerization of polysaccharide antigens. Exploring the processing pathways of glycans and why some glycoconjugate vaccines are less effective than expected: a review. Carbohydr Polym. 2023 Sep 1;315:120969. doi: 10.1016/j.carbpol.2023.120969
- Micoli F, Stefanetti G, MacLennan CA. Exploring the variables influencing the immune response of traditional and innovative glycoconjugate vaccines [review]. Front Mol Biosci. 2023 May 16;10:10. doi: 10.3389/fmolb.2023.1201693
- Middleton DR, Sun L, Paschall AV, et al. T Cell-Mediated Humoral Immune Responses to type 3 capsular polysaccharide of streptococcus pneumoniae. J Immunol. 2017 Jul 15;199(2):598–603. doi: 10.4049/jimmunol.1700026
- Sun X, Stefanetti G, Berti F, et al. Polysaccharide structure dictates mechanism of adaptive immune response to glycoconjugate vaccines. Proc Natl Acad Sci, USA. 2019 Jan 2;116(1):193–198. doi: 10.1073/pnas.1816401115
- Romano MR, Berti F, Rappuoli R. Classical- and bioconjugate vaccines: comparison of the structural properties and immunological response. Curr Opin Immunol. 2022 Oct 01;78:102235. doi: 10.1016/j.coi.2022.102235
- Del Bino L, Østerlid KE, Wu D-Y, et al. Synthetic glycans to improve Current glycoconjugate vaccines and fight antimicrobial resistance. Chem Rev. 2022 Oct 26;122(20):15672–15716. doi: 10.1021/acs.chemrev.2c00021
- Micoli F, Del Bino L, Alfini R, et al. Glycoconjugate vaccines: current approaches towards faster vaccine design. Expert Rev Vaccines. 2019 Sep 2;18(9):881–895. doi: 10.1080/14760584.2019.1657012
- Verez-Bencomo V, Fernández-Santana V, Hardy E, et al. A synthetic conjugate polysaccharide vaccine against haemophilus influenzae type b. Science. 2004 Jul 23;305(5683):522–525. doi: 10.1126/science.1095209
- Cohen D, Atsmon J, Artaud C, et al. Safety and immunogenicity of a synthetic carbohydrate conjugate vaccine against shigella flexneri 2a in healthy adult volunteers: a phase 1, dose-escalating, single-blind, randomised, placebo-controlled study. Lancet Infect Dis. 2021 Apr;21(4):546–558. doi: 10.1016/S1473-3099(20)30488-6
- Seeberger PH. Discovery of semi- and fully-synthetic carbohydrate vaccines against bacterial infections using a medicinal chemistry approach.Chem Rev. 2021;121(7):3598–3626. doi: 10.1021/acs.chemrev.0c01210
- Fiebig T, Freiberger F, Pinto V, et al. Molecular cloning and functional characterization of components of the capsule biosynthesis complex of Neisseria meningitidis serogroup A: toward in vitro vaccine production. J Biol Chem. 2014;289(28):19395–19407. doi: 10.1074/jbc.M114.575142
- Fiebig T, Romano MR, Oldrini D, et al. An efficient cell free enzyme-based total synthesis of a meningococcal vaccine candidate. NPJ Vaccines. 2016;1(1):16017. doi: 10.1038/npjvaccines.2016.17
- Oldrini D, Fiebig T, Romano MR, et al. Combined chemical synthesis and tailored enzymatic elongation provide fully synthetic and conjugation-ready Neisseria meningitidis serogroup X vaccine antigens. ACS Chem Biol. 2018 Apr 20;13(4):984–994. doi: 10.1021/acschembio.7b01057
- Berti F, Adamo R. Antimicrobial glycoconjugate vaccines: an overview of classic and modern approaches for protein modification [10.1039/C8CS00495A]. Chem Soc Rev. 2018;47(24):9015–9025. doi: 10.1039/C8CS00495A
- Kapoor N, Vanjak I, Rozzelle J, et al. Malaria derived glycosylphosphatidylinositol anchor enhances anti-Pfs25 functional antibodies that block malaria transmission. Biochem. 2018;57(5):516–519. doi: 10.1021/acs.biochem.7b01099
- Kapoor N, Uchiyama S, Pill L, et al. Non-native amino acid click chemistry-based technology for site-specific polysaccharide conjugation to a bacterial protein serving as both carrier and vaccine antigen. ACS Omega. 2022 Jul 19;7(28):24111–24120. doi: 10.1021/acsomega.1c07360
- Fairman J, Agarwal P, Barbanel S, et al. Non-clinical immunological comparison of a next-generation 24-valent pneumococcal conjugate vaccine (VAX-24) using site-specific carrier protein conjugation to the current standard of care (PCV13 and PPV23). Vaccine. 2021 May 27;39(23):3197–3206. doi: 10.1016/j.vaccine.2021.03.070
- Dow JM, Mauri M, Scott TA, et al. Improving protein glycan coupling technology (PGCT) for glycoconjugate vaccine production. Expert Rev Vaccines. 2020 June 2;19(6):507–527. doi: 10.1080/14760584.2020.1775077
- Wacker M, Linton D, Hitchen PG, et al. N-linked glycosylation in Campylobacter jejuni and its functional transfer into E. coli. Science. 2002 Nov 29;298(5599):1790–1793. doi: 10.1126/science.298.5599.1790
- Kay E, Cuccui J, Wren BW. Recent advances in the production of recombinant glycoconjugate vaccines.NPJ Vaccines. 2019 May 1;4(1):16. doi: 10.1038/s41541-019-0110-z
- Kowarik M, Young NM, Numao S, et al. Definition of the bacterial N-glycosylation site consensus sequence. EMBO J. 2006 May 3;25(9):1957–1966. doi: 10.1038/sj.emboj.7601087
- Kowarik M, Wetter M, Haeuptle MA, et al. The development and characterization of an E. coli O25B bioconjugate vaccine. Glycoconj J. 2021 Aug;38(4):421–435. doi: 10.1007/s10719-021-09985-9
- Herbert JA, Kay EJ, Faustini SE, et al. Production and efficacy of a low-cost recombinant pneumococcal protein polysaccharide conjugate vaccine. Vaccine. 2018 Jun 18;36(26):3809–3819. doi: 10.1016/j.vaccine.2018.05.036
- Harding CM, Feldman MF. Glycoengineering bioconjugate vaccines, therapeutics, and diagnostics in E. coli. Glycobiology. 2019 Jul 1;29(7):519–529. doi: 10.1093/glycob/cwz031
- Riddle MS, Kaminski RW, Di Paolo C, et al. Safety and immunogenicity of a candidate bioconjugate vaccine against shigella flexneri 2a administered to healthy adults: a single-blind, randomized phase I study. Clin Vaccine Immunol. 2016 Dec;23(12):908–917. doi: 10.1128/CVI.00224-16
- Talaat KR, Alaimo C, Martin P, et al. Human challenge study with a shigella bioconjugate vaccine: analyses of clinical efficacy and correlate of protection. EBioMedicine. 2021;66:66. doi: 10.1016/j.ebiom.2021.103310
- Saade E, Gravenstein S, Donskey CJ, et al. Characterization of Escherichia coli isolates potentially covered by ExPEC4V and ExPEC10V, that were collected from post-transrectal ultrasound-guided prostate needle biopsy invasive urinary tract and bloodstream infections. Vaccine. 2020 Jul 14;38(33):5100–5104. doi: 10.1016/j.vaccine.2020.06.024
- Scully IL, Timofeyeva Y, Illenberger A, et al. Performance of a Four-Antigen Staphylococcus aureus vaccine in preclinical models of invasive S. aureus disease. Microorganisms. 2021 Jan 15;9(1):177. doi: 10.3390/microorganisms9010177
- Gurtman A, Begier E, Mohamed N, et al. The development of a staphylococcus aureus four antigen vaccine for use prior to elective orthopedic surgery. Hum Vaccin Immunother. 2019;15(2):358–370. doi: 10.1080/21645515.2018.1523093
- Creech CB, Frenck RW, Fiquet A, et al. Persistence of immune responses through 36 months in healthy adults after vaccination with a novel staphylococcus aureus 4-antigen vaccine (SA4Ag). Open Forum Infect Dis. 2019;7(1). doi: 10.1093/ofid/ofz532
- Smith W B, Abbanat D, Spiessens B, et al. 2712. Safety and immunogenicity of two doses of ExPEC4V vaccine against extraintestinal pathogenic Escherichia coli disease in healthy adult participants. Open Forum Infect Dis. 2019;6(Supplement_2):S954–S954. doi: 10.1093/ofid/ofz360.2389
- Frenck RW Jr., Ervin J, Chu L, et al. Safety and immunogenicity of a vaccine for extra-intestinal pathogenic Escherichia coli (ESTELLA): a phase 2 randomised controlled trial. Lancet Infect Dis. 2019 Jun;19(6):631–640. doi: 10.1016/S1473-3099(18)30803-X
- Hülsdünker J, Thomas OS, Haring E, et al. Immunization against poly-N-acetylglucosamine reduces neutrophil activation and GVHD while sparing microbial diversity. Proc Natl Acad Sci, USA. 2019;116(41):20700–20706. doi: 10.1073/pnas.1908549116
- Patel M, Kaufman DA. Anti-lipoteichoic acid monoclonal antibody (pagibaximab) studies for the prevention of staphylococcal bloodstream infections in preterm infants. Expert Opin Biol Ther. 2015 Apr;15(4):595–600. doi: 10.1517/14712598.2015.1019857
- Chastre J, François B, Bourgeois M, et al. Safety, efficacy, and pharmacokinetics of gremubamab (MEDI3902), an anti-Pseudomonas aeruginosa bispecific human monoclonal antibody, in P. aeruginosa-colonised, mechanically ventilated intensive care unit patients: a randomised controlled trial. Crit Care. 2022 Nov 15;26(1):355. doi: 10.1186/s13054-022-04204-9
- Loos A, Weich N, Woo J, et al. 674. Pre-Clinical and Phase I Safety Data for Anti-Pseudomonas aeruginosa Human Monoclonal Antibody AR-105. Open Forum Infect Dis. 2019;6(Supplement_2):S307–S308. doi: 10.1093/ofid/ofz360.742
- López-Sagaseta J, Malito E, Rappuoli R, et al. Self-assembling protein nanoparticles in the design of vaccines. Comput Struct Biotechnol J. 2016;14:58–68. doi: 10.1016/j.csbj.2015.11.001
- Morelli L, Polito L, Richichi B, et al. Glyconanoparticles as tools to prevent antimicrobial resistance. Glycoconj J. 2021;38(4):475–490. doi: 10.1007/s10719-021-09988-6
- Doan TA, Forward T, Tamburini BAJ. Trafficking and retention of protein antigens across systems and immune cell types.Cell Mol Life Sci. 2022;79(5):275. doi: 10.1007/s00018-022-04303-4
- van der Put RMF, Metz B, Pieters RJ. Carriers and Antigens: new developments in glycoconjugate vaccines. Vaccines. 2023;11(2):219. doi: 10.3390/vaccines11020219
- Zottig X, Côté-Cyr M, Arpin D, et al. Protein supramolecular structures: from self-assembly to nanovaccine design. Nanomaterials. 2020;10(5):1008. doi: 10.3390/nano10051008
- Olshefsky A, Richardson C, Pun SH, et al. Engineering self-assembling protein nanoparticles for therapeutic delivery. Bioconjug Chem. 2022 Mar 16;33(11):2018–2034. doi: 10.1021/acs.bioconjchem.2c00030
- Fuenmayor J, Gòdia F, Cervera L. Production of virus-like particles for vaccines. N Biotechnol. 2017 Oct 25;39(Pt B):174–180. doi: 10.1016/j.nbt.2017.07.010
- Venters C, Graham W, Cassidy W. Recombivax-HB: perspectives past, present and future.Expert Rev Vaccines. 2004 June 1;3(2):119–129. doi: 10.1586/14760584.3.2.119
- Kheirollahpour M, Mehrabi M, Dounighi NM, et al. Nanoparticles and Vaccine Development. Pharm Nanotechnol. 2020;8(1):6–21. doi: 10.2174/2211738507666191024162042
- Polonskaya Z, Deng S, Sarkar A, et al. T cells control the generation of nanomolar-affinity anti-glycan antibodies. J Clin Invest. 2017 Apr 3;127(4):1491–1504. doi: 10.1172/JCI91192
- Xu L, Li Z, Su Z, et al. Development of meningococcal polysaccharide conjugate vaccine that can elicit long-lasting and strong cellular immune response with hepatitis B core antigen virus-like particles as a novel carrier protein. Vaccine. 2019 Aug 8;37(7):956–964. doi: 10.1016/j.vaccine.2018.12.073
- Rashidijahanabad Z, Kelly M, Kamruzzaman M, et al. Virus-like particle display of vibrio choleraeO-Specific polysaccharide as a potential vaccine against cholera. ACS Infect Dis. 2022 Mar 11;8(3):574–583. doi: 10.1021/acsinfecdis.1c00585
- King NP, Sheffler W, Sawaya MR, et al. Computational design of self-assembling protein nanomaterials with atomic level accuracy. Science. 2012 Jun 1;336(6085):1171–1174. doi: 10.1126/science.1219364
- Correnti CE, Hallinan JP, Doyle LA, et al. Engineering and functionalization of large circular tandem repeat protein nanoparticles. Nat Struct Mol Biol. 2020;27(4):342–350. doi: 10.1038/s41594-020-0397-5
- Marcandalli J, Fiala B, Ols S, et al. Induction of potent neutralizing antibody responses by a designed protein nanoparticle vaccine for respiratory syncytial virus. Cell. 2019 Mar 7;176(6):1420–1431.e17. doi: 10.1016/j.cell.2019.01.046
- Walls AC, Fiala B, Schäfer A, et al. Elicitation of potent neutralizing antibody responses by designed protein nanoparticle vaccines for SARS-CoV-2. Cell. 2020 Nov 25;183(5):1367–1382.e17. doi: 10.1016/j.cell.2020.10.043
- Arunachalam PS, Walls AC, Golden N, et al. Adjuvanting a subunit COVID-19 vaccine to induce protective immunity. Nature. 2021 June 1;594(7862):253–258. doi: 10.1038/s41586-021-03530-2
- Boyoglu-Barnum S, Ellis D, Gillespie RA, et al. Quadrivalent influenza nanoparticle vaccines induce broad protection. Nature. 2021 Apr;592(7855):623–628. doi: 10.1038/s41586-021-03365-x
- Bernadac A, Gavioli M, Lazzaroni JC, et al. Escherichia coli tol-pal mutants form outer membrane vesicles. J Bacteriol. 1998 Sep;180(18):4872–4878. doi: 10.1128/JB.180.18.4872-4878.1998
- Turner L, Praszkier J, Hutton ML, et al. Increased Outer Membrane Vesicle Formation in a Helicobacter pylori tolB Mutant. Helicobacter. 2015 Aug;20(4):269–283. doi: 10.1111/hel.12196
- Mancini F, Micoli F, Necchi F, et al. GMMA-Based vaccines: the known and the unknown. Front Immunol. 2021;12:715393. doi: 10.3389/fimmu.2021.715393
- Micoli F, Rossi O, Conti V, et al. Antibodies elicited by the shigella sonnei GMMA vaccine in adults trigger complement-mediated serum bactericidal activity: results from a phase 1 dose escalation trial followed by a booster extension. Front Immunol. 2021;12:671325. doi: 10.3389/fimmu.2021.671325
- Di Benedetto R, Alfini R, Carducci M, et al. Novel simple conjugation chemistries for decoration of GMMA with Heterologous Antigens. Int J Mol Sci. 2021 Sep 22;22(19):10180. doi: 10.3390/ijms221910180
- Palmieri E, Kis Z, Ozanne J, et al. GMMA as an alternative carrier for a glycoconjugate vaccine against group a streptococcus. Vaccines (Basel). 2022 Jun 28;10(7):1034. doi: 10.3390/vaccines10071034
- Gasperini G, Alfini R, Arato V, et al. Salmonella Paratyphi A Outer Membrane Vesicles Displaying Vi Polysaccharide as a Multivalent Vaccine against Enteric Fever. Infect Immun. 2021 Mar 17;89(4). doi: 10.1128/IAI.00699-20
- Micoli F, Alfini R, Di Benedetto R, et al. Generalized modules for membrane antigens as carrier for polysaccharides: impact of sugar length, density, and attachment site on the immune response elicited in animal models. Front Immunol. 2021;12:719315. doi: 10.3389/fimmu.2021.719315
- Raso MM, Gasperini G, Alfini R, et al. GMMA and glycoconjugate approaches compared in mice for the development of a vaccine against shigella flexneri serotype 6. Vaccines (Basel). 2020 Apr 3;8(2):160. doi: 10.3390/vaccines8020160
- Valguarnera E, Feldman MF. Glycoengineered outer membrane vesicles as a platform for vaccine development. Methods Enzymol. 2017;597:285–310.
- Gnopo YMD, Watkins HC, Stevenson TC, et al. Designer outer membrane vesicles as immunomodulatory systems - reprogramming bacteria for vaccine delivery. Adv Drug Deliv Rev. 2017 May 15;114:132–142. doi: 10.1016/j.addr.2017.05.003
- Chen L, Valentine JL, Huang CJ, et al. Outer membrane vesicles displaying engineered glycotopes elicit protective antibodies. Proc Natl Acad Sci, USA. 2016 Jun 28;113(26):E3609–18. doi: 10.1073/pnas.1518311113
- Price NL, Goyette-Desjardins G, Nothaft H, et al. Glycoengineered Outer Membrane Vesicles: A Novel Platform for Bacterial Vaccines. Sci Rep. 2016 Apr 22;6(1):24931. doi: 10.1038/srep24931
- Stevenson TC, Cywes-Bentley C, Moeller TD, et al. Immunization with outer membrane vesicles displaying conserved surface polysaccharide antigen elicits broadly antimicrobial antibodies. Proc Natl Acad Sci, USA. 2018;115(14):E3106–E3115. doi: 10.1073/pnas.1718341115
- Zhang F, Lu YJ, Malley R. Multiple antigen-presenting system (MAPS) to induce comprehensive B- and T-cell immunity. Proc Natl Acad Sci, USA. 2013 Aug 13;110(33):13564–13569. doi: 10.1073/pnas.1307228110
- Zhang F, Thompson C, Ma N, et al. Carrier proteins facilitate the generation of antipolysaccharide immunity via multiple mechanisms. mBio. MBio. 2022 Jun 28;13(3):e0379021. doi: 10.1128/mbio.03790-21
- Zhang F, Ledue O, Jun M, et al. Protection against staphylococcus aureus colonization and infection by B- and T-Cell-mediated mechanisms. MBio. 2018 Oct 16;9(5). doi: 10.1128/mBio.01949-18
- Chichili GR, Smulders R, Santos V, et al. Phase 1/2 study of a novel 24-valent pneumococcal vaccine in healthy adults aged 18 to 64 years and in older adults aged 65 to 85 years. Vaccine. 2022 Jul 29;40(31):4190–4198. doi: 10.1016/j.vaccine.2022.05.079
- Choi M, Tennant SM, Simon R, et al. Progress towards the development of klebsiella vaccines. Expert Rev Vaccines. 2019 Jul;18(7):681–691. doi: 10.1080/14760584.2019.1635460
- CDC. Antibiotic resistance threats in the United States, 2019. Atlanta GA: US Department of Health and Human Services; 2019.
- Kaper JB, Nataro JP, Mobley HLT. Pathogenic Escherichia coli.Nature Rev Microbiol. 2004 Feb 1;2(2):123–140. doi: 10.1038/nrmicro818
- Khalil IA, Troeger C, Blacker BF, et al. Morbidity and mortality due to shigella and enterotoxigenic Escherichia coli diarrhoea: the global burden of disease study 1990-2016. Lancet Infect Dis. 2018 Nov;18(11):1229–1240. doi: 10.1016/S1473-3099(18)30475-4
- O’Brien VP, Hannan TJ, Nielsen HV, et al. Drug and vaccine development for the treatment and prevention of urinary tract infections. Microbiol Spectr. 2016 Feb;4(1). doi: 10.1128/microbiolspec.UTI-0013-2012
- Brumbaugh AR, Mobley HL. Preventing urinary tract infection: progress toward an effective Escherichia coli vaccine. Expert Rev Vaccines. 2012 Jun;11(6):663–676. doi: 10.1586/erv.12.36
- McLellan LK, Hunstad DA. Urinary tract infection: pathogenesis and outlook.Trends Mol Med. 2016 Nov 1;22(11):946–957. doi: 10.1016/j.molmed.2016.09.003
- Pokharel P, Dhakal S, Dozois CM. The diversity of Escherichia coli Pathotypes and vaccination strategies against this versatile bacterial pathogen. Microorganisms. 2023;11(2):344. doi: 10.3390/microorganisms11020344
- Chorro L, Li Z, Chu L, et al. Preclinical immunogenicity and efficacy of optimized O25b O-Antigen glycoconjugates to prevent MDR ST131 E. coli infections. Infect Immun. 2022 Apr 21;90(4):e0002222. doi: 10.1128/iai.00022-22
- McGuinness WA, Malachowa N, DeLeo FR. Vancomycin resistance in staphylococcus aureus. Yale J Biol Med. 2017 Jun;90(2):269–281.
- Olaniyi R, Pozzi C, Grimaldi L, et al. Staphylococcus aureus-associated skin and soft tissue infections: anatomical localization, epidemiology, therapy and potential prophylaxis. Curr Top Microbiol Immunol. 2017;409:199–227.
- Humphreys H, Becker K, Dohmen PM, et al. Staphylococcus aureus and surgical site infections: benefits of screening and decolonization before surgery. J Hosp Infect. 2016;94(3):295–304. doi: 10.1016/j.jhin.2016.06.011
- Gooch JR, Serazin A, Schwarm E, et al. Vaccines to tackle drug resistant infections - an evaluation of R&D opportunities the boston consulting group/wellcome trust. 2018. https://vaccinesforamr.org/
- Sands K, Carvalho MJ, Portal E, et al. Characterization of antimicrobial-resistant Gram-negative bacteria that cause neonatal sepsis in seven low- and middle-income countries. Nat Microbiol. 2021 Apr;6(4):512–523. doi: 10.1038/s41564-021-00870-7
- Priebe GP, Goldberg JB. Vaccines for Pseudomonas aeruginosa: a long and winding road. Expert Rev Vaccines. 2014 Apr;13(4):507–519. doi: 10.1586/14760584.2014.890053
- El Zowalaty ME, Al Thani AA, Webster TJ, et al. Pseudomonas aeruginosa: arsenal of resistance mechanisms, decades of changing resistance profiles, and future antimicrobial therapies. Future Microbiol. 2015;10(10):1683–1706. doi: 10.2217/fmb.15.48
- Zhao M, Qin C, Li L, et al. Conjugation of synthetic trisaccharide of staphylococcus aureus type 8 capsular polysaccharide elicits antibodies recognizing intact bacterium. Front Chem. 2020;8:258. doi: 10.3389/fchem.2020.00258
- Cheng BL, Nielsen TB, Pantapalangkoor P, et al. Evaluation of serotypes 5 and 8 capsular polysaccharides in protection against staphylococcus aureus in murine models of infection. Hum Vaccin Immunother. 2017 Jul 3;13(7):1609–1614. doi: 10.1080/21645515.2017.1304334
- Yang FL, Lou TC, Kuo SC, et al. A medically relevant capsular polysaccharide in Acinetobacter baumannii is a potential vaccine candidate. Vaccine. 2017 Mar 7;35(10):1440–1447. doi: 10.1016/j.vaccine.2017.01.060
- Rudenko N, Karatovskaya A, Zamyatina A, et al. Immune response to conjugates of fragments of the type K9 capsular polysaccharide of acinetobacter baumannii with carrier proteins. Microbiol Spectr. 2022 Oct 26;10(5):e0167422. doi: 10.1128/spectrum.01674-22
- Kobayashi SD, Porter AR, Freedman B, et al. Antibody-mediated killing of carbapenem-resistant ST258 Klebsiella pneumoniae by human neutrophils. MBio. 2018 Mar 13;9(2). doi: 10.1128/mBio.00297-18
- Kalfopoulou E, Laverde D, Miklic K, et al. Development of Opsonic Mouse Monoclonal Antibodies against Multidrug-Resistant Enterococci. Infect Immun. 2019 Sep;87(9). doi: 10.1128/IAI.00276-19
- Romero-Saavedra F, Laverde D, Kalfopoulou E, et al. Conjugation of different immunogenic enterococcal vaccine target antigens leads to extended strain coverage. J Infect Dis. 2019 Oct 8;220(10):1589–1598. doi: 10.1093/infdis/jiz357
- Laverde D, Romero-Saavedra F, Argunov DA, et al. Synthetic oligomers mimicking capsular polysaccharide diheteroglycan are potential vaccine candidates against encapsulated enterococcal infections. ACS Infect Dis. 2020 Jul 10;6(7):1816–1826. doi: 10.1021/acsinfecdis.0c00063
- Feldman MF, Mayer Bridwell AE, Scott NE, et al. A promising bioconjugate vaccine against hypervirulent Klebsiella pneumoniae. Proc Natl Acad Sci, USA. 2019 Sep 10;116(37):18655–18663. doi: 10.1073/pnas.1907833116
- Ravinder M, Liao KS, Cheng YY, et al. A Synthetic Carbohydrate-Protein Conjugate Vaccine Candidate against Klebsiella pneumoniae Serotype K2. J Org Chem. 2020 Dec 18;85(24):15964–15997. doi: 10.1021/acs.joc.0c01404
- Maleki M, Azimi S, Salouti M. Protective effect of two new nanovaccines against Pseudomonas aeruginosa based on LPS and OPS: a comparison study. Immunobiol. 2022 Nov;227(6):152278. doi: 10.1016/j.imbio.2022.152278
- Najafzadeh F, Tanomand A, Hadadi A, et al. Immunological Properties of Exotoxin A Toxoid - Detoxified Lipopolysaccharide - Gold Nanoparticles Conjugate Against Pseudomonas aeruginosa Infection. Iran J Immunol. 2021;18(4):292–303. doi: 10.22034/IJI.2021.87816.1832
- Hegerle N, Choi M, Sinclair J, et al. Development of a broad spectrum glycoconjugate vaccine to prevent wound and disseminated infections with Klebsiella pneumoniae and Pseudomonas aeruginosa. PLoS One. 2018;13(9):e0203143. doi: 10.1371/journal.pone.0203143
- Cross AT, Michon F. Novel multivalent vaccine for Gram-negative bacterial pathogens including multiple antibiotic resistant strains. 18th Annual World Vaccine Congress; (WA) DC USA 2019.
- Naini A, Bartetzko MP, Sanapala SR, et al. Semisynthetic glycoconjugate vaccine candidates against Escherichia coli O25B induce functional IgG antibodies in mice. J Am Chem Soc Au. 2022;2(9):2135–2151. doi: 10.1021/jacsau.2c00401
- Williams AJ, Warfel KF, Desai P, et al. A low-cost recombinant glycoconjugate vaccine confers immunogenicity and protection against enterotoxigenic Escherichia coli infections in mice [original research]. Front Mol Biosci. 2023 Mar 2;10:10.
- Kong L, Vijayakrishnan B, Kowarik M, et al. An antibacterial vaccination strategy based on a glycoconjugate containing the core lipopolysaccharide tetrasaccharide Hep2Kdo2. Nat Chem. 2016 Mar 1;8(3):242–249. doi: 10.1038/nchem.2432
- Jamshidi MP, Cairns C, Chong S, et al. Synthesis and immunogenicity of a methyl rhamnan pentasaccharide conjugate from Pseudomonas aeruginosa A-Band polysaccharide. ACS Infect Dis. 2022 Jul 8;8(7):1347–1355. doi: 10.1021/acsinfecdis.2c00184
- Zhang L, Zhang Y, Hua Q, et al. Promoter-controlled synthesis and antigenic evaluation of mannuronic acid alginate glycans of Pseudomonas aeruginosa. Org Lett. 2022 Nov 18;24(45):8381–8386. doi: 10.1021/acs.orglett.2c03439
- Azimi S, Safari Zanjani L. Immunization against Pseudomonas aeruginosa using Alg-PLGA nano-vaccine. Iran J Basic Med Sci. 2021 Apr;24(4):476–482. doi: 10.22038/ijbms.2021.52217.11813
- Afshari H, Maleki M, Hakimian M, et al. Immunogenicity evaluating of the SLNs-alginate conjugate against Pseudomonas aeruginosa. J Immunol Methods. 2021 Jan;488:112938.
- Farjah A, Owlia P, Siadat SD, et al. Immunological evaluation of an alginate-based conjugate as a vaccine candidate against Pseudomonas aeruginosa. APMIS. 2015 Feb;123(2):175–183. doi: 10.1111/apm.12337
- Gonzaga ZJC, Merakou C, DiGiandomenico A, et al. A Pseudomonas aeruginosa-Derived Particulate Vaccine Protects against P. aeruginosa Infection. Vaccines (Basel). 2021 Jul 20;9(7):803. doi: 10.3390/vaccines9070803
- Lee JW, Parlane NA, Wedlock DN, et al. Bioengineering a bacterial pathogen to assemble its own particulate vaccine capable of inducing cellular immunity. Sci Rep. 2017;7(1):41607. doi: 10.1038/srep41607
- Lee IM, Yang F-L, Chen T-L, et al. Pseudaminic acid on exopolysaccharide of acinetobacter baumannii plays a critical role in phage-assisted preparation of glycoconjugate vaccine with high antigenicity. J Am Chem Soc. 2018 July 18;140(28):8639–8643. doi: 10.1021/jacs.8b04078
- Wei R, Yang X, Liu H, et al. Synthetic pseudaminic-acid-based antibacterial vaccine confers effective protection against acinetobacter baumannii infection. ACS Cent Sci. 2021 Sep 22;7(9):1535–1542. doi: 10.1021/acscentsci.1c00656
- Kodali S, Vinogradov E, Lin F, et al. A vaccine approach for the prevention of infections by multidrug-resistant Enterococcus faecium. J Biol Chem. 2015 Aug 7;290(32):19512–19526. doi: 10.1074/jbc.M115.655852
- Gholami SA, Goli HR, Haghshenas MR, et al. Evaluation of polysaccharide intercellular adhesion (PIA) and glycerol teichoic acid (gly-TA) arisen antibodies to prevention of biofilm formation in staphylococcus aureus and Staphylococcus epidermidis strains. BMC Res Notes. 2019 Oct 25;12(1):691. doi: 10.1186/s13104-019-4736-8
- Laverde D, Wobser D, Romero-Saavedra F, et al. Synthetic teichoic acid conjugate vaccine against nosocomial gram-positive bacteria. PLoS One. 2014;9(10):e110953. doi: 10.1371/journal.pone.0110953
- Zhou Z, Ding W, Li C, et al. Synthesis and immunological study of a wall teichoic acid-based vaccine against E. faecium U0317. J Carbohydr Chem. 2017 June 13;36(4–6):205–219. doi: 10.1080/07328303.2017.1390576
- Micoli F, Romano MR, Carboni F, et al. Strengths and weaknesses of pneumococcal conjugate vaccines. Glycoconj J. 2023 Apr;40(2):135–148. doi: 10.1007/s10719-023-10100-3
- Converso TR, Assoni L, André GO, et al. The long search for a serotype independent pneumococcal vaccine. Expert Rev Vaccines. 2020 Jan 2;19(1):57–70. doi: 10.1080/14760584.2020.1711055
- Ali S, Berni F, Enotarpi J, et al. Synthetic teichoic acid chemistry for vaccine applications. In: Rauter A, Christensen B, Somsák L, et al. Recent trends in carbohydrate chemistry 2 . Elsevier; 2020. p. 207–238 doi: 10.1016/B978-0-12-820954-7.00006-2
- Le Mauff F, Razvi E, Reichhardt C, et al. The pel polysaccharide is predominantly composed of a dimeric repeat of α-1,4 linked galactosamine and N-acetylgalactosamine. Commun Biol. 2022 May 26;5(1):502. doi: 10.1038/s42003-022-03453-2
- Franklin M, Nivens D, Weadge J, et al. Biosynthesis of the Pseudomonas aeruginosa Extracellular Polysaccharides, Alginate, Pel, and Psl [Review]. Front Microbiol. 2011 Aug 22;2. doi: 10.3389/fmicb.2011.00167
- van Dalen R, Peschel A, van Sorge NM. Wall teichoic acid in staphylococcus aureus host interaction. Trends Microbiol. 2020;28(12):985–998. doi: 10.1016/j.tim.2020.05.017
- Hsieh SA, Allen PM. Immunomodulatory roles of polysaccharide capsules in the intestine. Front Immunol. 2020;11:690. doi: 10.3389/fimmu.2020.00690
- Caroff M, Novikov A. Lipopolysaccharides: structure, function and bacterial identifications☆☆☆. OCL. 2020;27:31. doi: 10.1051/ocl/2020025
- Zhu H, Rollier CS, Pollard AJ. Recent advances in lipopolysaccharide-based glycoconjugate vaccines.Expert Rev Vaccines. 2021 Dec 2;20(12):1515–1538. doi: 10.1080/14760584.2021.1984889
- Singh R, Capalash N, Sharma P. Vaccine development to control the rising scourge of antibiotic-resistant acinetobacter baumannii: a systematic review. 3 Biotech. 2022 Mar;12(3):85. doi: 10.1007/s13205-022-03148-9
- Giguère D. Surface polysaccharides from acinetobacter baumannii: structures and syntheses. Carbohydr Res. 2015;418:29–43. doi: 10.1016/j.carres.2015.10.001
- Russo TA, Beanan JM, Olson R, et al. The K1 capsular polysaccharide from Acinetobacter baumannii is a potential therapeutic target via passive immunization. Infect Immun. 2013 Mar;81(3):915–922. doi: 10.1128/IAI.01184-12
- Sianturi J, Priegue P, Hu J, et al. Semi-synthetic glycoconjugate vaccine lead against acinetobacter baumannii 17978. Angew Chem Int Ed Engl. 2022 Oct 10;61(41):e202209556. doi: 10.1002/anie.202209556
- Li X, Pan C, Liu Z, et al. Safety and immunogenicity of a new glycoengineered vaccine against acinetobacter baumannii in mice. Microbiol Biotechnol. 2022 Feb;15(2):703–716. doi: 10.1111/1751-7915.13770
- Pantophlet R, Nemec A, Brade L, et al. O-antigen diversity among acinetobacter baumannii strains from the Czech Republic and Northwestern Europe, as determined by lipopolysaccharide-specific monoclonal antibodies. J Clin Microbiol. 2001 Jul;39(7):2576–2580. doi: 10.1128/JCM.39.7.2576-2580.2001
- Whitfield C. Biosynthesis and assembly of capsular polysaccharides in Escherichia coli. Annu Rev Biochem. 2006;75(1):39–68. doi: 10.1146/annurev.biochem.75.103004.142545
- Huttner A, Hatz C, Dobbelsteen G, et al. Safety, immunogenicity, and preliminary clinical efficacy of a vaccine against extraintestinal pathogenic Escherichia coli in women with a history of recurrent urinary tract infection: a randomised, single-blind, placebo-controlled phase 1b trial. Lancet Infect Dis. 2017 Feb 01;17(5):528–537. doi: 10.1016/S1473-3099(17)30108-1
- Nesta B, Valeri M, Spagnuolo A, et al. SslE elicits functional antibodies that impair in vitro mucinase activity and in vivo colonization by both intestinal and extraintestinal Escherichia coli strains. PLOS Pathog. 2014 May;10(5):e1004124. doi: 10.1371/journal.ppat.1004124
- Riaz S, Steinsland H, Thorsing M, et al. Characterization of glycosylation-specific systemic and mucosal IgA antibody responses to Escherichia coli mucinase YghJ (SslE). Front Immunol. 2021;12:760135. doi: 10.3389/fimmu.2021.760135
- Szardenings M, Kern K, Delaroque N, et al. Glycosylation of bacterial antigens changes epitope patterns. Front Immunol. 2023;14:1258136. doi: 10.3389/fimmu.2023.1258136
- Huebner J, Wang Y, Krueger WA, et al. Isolation and chemical characterization of a capsular polysaccharide antigen shared by clinical isolates of Enterococcus faecalis and vancomycin-resistant Enterococcus faecium. Infect Immun. 1999 Mar;67(3):1213–1219. doi: 10.1128/IAI.67.3.1213-1219.1999
- Wang Y, Huebner J, Tzianabos AO, et al. Structure of an antigenic teichoic acid shared by clinical isolates of Enterococcus faecalis and vancomycin-resistant Enterococcus faecium. Carbohydr Res. 1999 Mar 31;316(1–4):155–160. doi: 10.1016/S0008-6215(99)00046-4
- Hufnagel M, Carey VJ, Baldassarri L, et al. Distribution of four capsular serotypes of Enterococcus faecalis among clinical isolates from different geographical origins and infection sites. Infection. 2006 Feb;34(1):22–25. doi: 10.1007/s15010-006-4100-5
- Huebner J, Quaas A, Krueger WA, et al. Prophylactic and therapeutic efficacy of antibodies to a capsular polysaccharide shared among vancomycin-sensitive and -resistant enterococci. Infect Immun. 2000 Aug;68(8):4631–4636. doi: 10.1128/IAI.68.8.4631-4636.2000
- Theilacker C, Kaczyński Z, Kropec A, et al. Serodiversity of opsonic antibodies against Enterococcus faecalis–glycans of the cell wall revisited. PLoS One. 2011 Mar 18;6(3):e17839. doi: 10.1371/journal.pone.0017839
- Ali L, Blum HE, Sakιnç T. Detection and characterization of bacterial polysaccharides in drug-resistant enterococci.Glycoconj J. 2019;36(5):429–438. doi: 10.1007/s10719-019-09881-3
- Palmer KL, Godfrey P, Griggs A, et al. Comparative genomics of enterococci: variation in Enterococcus faecalis, clade structure in E. faecium, and defining characteristics of E. gallinarum and E. casseliflavus. MBio. 2012;3(1):e00318–11. doi: 10.1128/mBio.00318-11
- Ali L, Spiess M, Wobser D, et al. Identification and functional characterization of the putative polysaccharide biosynthesis protein (CapD) of enterococcus faecium U0317. Infect Genet Evol. 2016;37:215–224.
- Bychowska A, Theilacker C, Czerwicka M, et al. Chemical structure of wall teichoic acid isolated from enterococcus faecium strain U0317. Carbohydr Res. 2011 Dec 13;346(17):2816–2819. doi: 10.1016/j.carres.2011.09.026
- Pan Y-J, Lin T-L, Chen C-T, et al. Genetic analysis of capsular polysaccharide synthesis gene clusters in 79 capsular types of klebsiella spp. Sci Rep. 2015 Oct 23;5(1):15573. doi: 10.1038/srep15573
- Edelman R, Taylor DN, Wasserman SS, et al. Phase 1 trial of a 24-valent Klebsiella capsular polysaccharide vaccine and an eight-valent Pseudomonas O-polysaccharide conjugate vaccine administered simultaneously. Vaccine. 1994 Nov;12(14):1288–1294. doi: 10.1016/S0264-410X(94)80054-4
- Granström M, Wretlind B, Markman B, et al. Enzyme-linked immunosorbent assay to evaluate the immunogenicity of a polyvalent Klebsiella capsular polysaccharide vaccine in humans. J Clin Microbiol. 1988 Nov;26(11):2257–2261. doi: 10.1128/jcm.26.11.2257-2261.1988
- Cryz SJ Jr., Mortimer P, Cross AS, et al. Safety and immunogenicity of a polyvalent Klebsiella capsular polysaccharide vaccine in humans. Vaccine. 1986 Mar;4(1):15–20. doi: 10.1016/0264-410X(86)90092-7
- Arato V, Raso MM, Gasperini G, et al. Prophylaxis and treatment against Klebsiella pneumoniae: Current insights on this emerging anti-microbial resistant global threat. Int J Mol Sci. 2021 Apr 14;22(8):4042. doi: 10.3390/ijms22084042
- Alexander JW, Fisher MW, MacMillan BG. Immunological control of pseudomonas infection in burn patients: a clinical evaluation. Arch Surg. 1971 Jan;102(1):31–35. doi: 10.1001/archsurg.1971.01350010033008
- Haghbin M, Armstrong D, Murphy ML. Controlled prospective trial of Pseudomonas aeruginosa vaccine in children with acute leukemia. Cancer. 1973;32(4):761–766. doi: 10.1002/1097-0142(197310)32:4<761:AID-CNCR2820320405>3.0.CO;2-H
- Young LS, Meyer RD, Armstrong D. Pseudomonas aeruginosa vaccine in cancer patients. Ann Intern Med. 1973 Oct;79(4):518–527. doi: 10.7326/0003-4819-79-4-518
- Pennington JE, Reynolds HY, Wood RE, et al. Use of a Pseudomonas Aeruginosa vaccine in patients with acute leukemia and cystic fibrosis. Am J Med. 1975 May;58(5):629–636. doi: 10.1016/0002-9343(75)90498-2
- Hortobagyi GN, Gutterman JU, Snyder RD, et al. Pseudomonas vaccine: a phase I evaluation for cancer research. Cancer Immunol Immunother. 1978;4(3):201–207. doi: 10.1007/BF00204741
- MacIntyre S, McVeigh T, Owen P. Immunochemical and biochemical analysis of the polyvalent Pseudomonas aeruginosa vaccine PEV. Infect Immun. 1986 Feb;51(2):675–686. doi: 10.1128/iai.51.2.675-686.1986
- Jones RJ, Roe EA, Gupta JL. Low mortality in burned patients in a pseudomonas vaccine trial. Lancet. 1978 Aug 19;2(8086):401–403. doi: 10.1016/S0140-6736(78)91868-8
- Langford DT, Hiller J. Prospective, controlled study of a polyvalent pseudomonas vaccine in cystic fibrosis–three year results. Arch Dis Child. 1984 Dec;59(12):1131–1134. doi: 10.1136/adc.59.12.1131
- Döring G, Pier GB. Vaccines and immunotherapy against Pseudomonas aeruginosa. Vaccine. 2008 Feb 20;26(8):1011–1024. doi: 10.1016/j.vaccine.2007.12.007
- Cryz SJ Jr., Fürer E, Cross AS, et al. Safety and immunogenicity of a Pseudomonas aeruginosa O-polysaccharide toxin A conjugate vaccine in humans. J Clin Investig. 1987 July 01;80(1):51–56. doi: 10.1172/JCI113062
- Cryz SJ, Sadoff JC, Fürer E. Octavalent Pseudomonas aeruginosa O-polysaccharide-toxin A conjugate vaccine.Microb Pathog. 1989 Jan 1;6(1):75–80. doi: 10.1016/0882-4010(89)90010-7
- Lang AB, Rüdeberg A, Schöni MH, et al. Vaccination of cystic fibrosis patients against Pseudomonas aeruginosa reduces the proportion of patients infected and delays time to infection. Pediatr Infect Dis J. 2004 Jun;23(6):504–510. doi: 10.1097/01.inf.0000129688.50588.ac
- Cryz SJ Jr., Wedgwood J, Lang AB, et al. Immunization of noncolonized cystic fibrosis patients against Pseudomonas aeruginosa. J Infect Dis. 1994;169(5):1159–1162. doi: 10.1093/infdis/169.5.1159
- Killough M, Rodgers AM, Ingram RJ. Pseudomonas aeruginosa: Recent Advances in Vaccine Development. Vaccines (Basel). 2022 Jul 8;10(7). doi: 10.3390/vaccines10071100
- Cairns CM, Michael FS, Jamshidi M, et al. Structural characterization and evaluation of an epitope at the tip of the A-Band rhamnan polysaccharide of Pseudomonas aeruginosa. ACS Infect Dis. 2022;8(7):1336–1346. doi: 10.1021/acsinfecdis.2c00183
- Nanra JS, Buitrago SM, Crawford S, et al. Capsular polysaccharides are an important immune evasion mechanism for Staphylococcus aureus. Hum Vaccin Immunother. 2013 Mar;9(3):480–487. doi: 10.4161/hv.23223
- Lockhart SP, Scott DA, Jansen KU, et al. Glycoconjugate vaccines: the clinical journey. Carbohydrate-based vaccines: from concept to clinic. ACS symposium series. Vol. 1290. USA: American Chemical Society; 2018. p. 7–59 doi: 10.1021/bk-2018-1290.ch002
- Fattom AI, Horwith G, Fuller S, et al. Development of StaphVAX, a polysaccharide conjugate vaccine against S. aureus infection: from the lab bench to phase III clinical trials. Vaccine. 2004 Feb 17;22(7):880–887. doi: 10.1016/j.vaccine.2003.11.034
- Fattom A, Fuller S, Propst M, et al. Safety and immunogenicity of a booster dose of staphylococcus aureus types 5 and 8 capsular polysaccharide conjugate vaccine (StaphVAX) in hemodialysis patients. Vaccine. 2004 Dec 16;23(5):656–663. doi: 10.1016/j.vaccine.2004.06.043
- Fattom A, Matalon A, Buerkert J, et al. Efficacy profile of a bivalent staphylococcus aureus glycoconjugated vaccine in adults on hemodialysis: phase III randomized study. Hum Vaccin Immunother. 2015;11(3):632–641. doi: 10.4161/hv.34414
- Levy J, Licini L, Haelterman E, et al. Safety and immunogenicity of an investigational 4-component staphylococcus aureus vaccine with or without AS03B adjuvant: results of a randomized phase I trial. Hum Vaccin Immunother. 2015;11(3):620–631. doi: 10.1080/21645515.2015.1011021
- Nissen M, Marshall H, Richmond P, et al. A randomized phase I study of the safety and immunogenicity of three ascending dose levels of a 3-antigen staphylococcus aureus vaccine (SA3Ag) in healthy adults. Vaccine. 2015 Apr 8;33(15):1846–1854. doi: 10.1016/j.vaccine.2015.02.024
- Frenck RW Jr., Creech CB, Sheldon EA, et al. Safety, tolerability, and immunogenicity of a 4-antigen staphylococcus aureus vaccine (SA4Ag): results from a first-in-human randomised, placebo-controlled phase 1/2 study. Vaccine. 2017 Jan 5;35(2):375–384. doi: 10.1016/j.vaccine.2016.11.010
- Fattom A, Li X, Cho YH, et al. Effect of conjugation methodology, carrier protein, and adjuvants on the immune response to staphylococcus aureus capsular polysaccharides. Vaccine. 1995 Oct;13(14):1288–1293. doi: 10.1016/0264-410X(95)00052-3
- Bagnoli F, Bertholet S, Grandi G. Inferring reasons for the failure of staphylococcus aureus vaccines in clinical trials [opinion]. Front Cell Infect Microbiol. 2012 Feb 22;2. doi: 10.3389/fcimb.2012.00016
- Pier GB. Will there ever be a universal Staphylococcus aureus vaccine?Hum Vaccines Immunother. 2013;9(9):1865–1876. doi: 10.4161/hv.25182
- Elli S, Alekseeva A, Ramakrishnan B, et al. Characterization of an antibody recognizing the conserved inner core of Pseudomonas aeruginosa lipopolysaccharides. Biochemistry. 2020;59(43):4202–4211. doi: 10.1021/acs.biochem.0c00642
- van Dalen R, Molendijk MM, Ali S, et al. Do not discard Staphylococcus aureus WTA as a vaccine antigen. Nature. 2019;572(7767):E1–E2. doi: 10.1038/s41586-019-1416-8
- Di Carluccio C, Soriano-Maldonado P, Berni F, et al. Antibody recognition of different staphylococcus aureus wall teichoic acid glycoforms. ACS Cent Sci. 2022;8(10):1383–1392. doi: 10.1021/acscentsci.2c00125
- Berni F, Kalfopoulou E, Gimeno Cardells AM, et al. Epitope recognition of a Monoclonal antibody raised against a synthetic glycerol phosphate based teichoic acid. ACS Chem Biol. 2021;16(8):1344–1349. doi: 10.1021/acschembio.1c00422
- van der Es D, Hogendorf WFJ, Overkleeft HS, et al. Teichoic acids: synthesis and applications [10.1039/C6CS00270F]. Chem Soc Rev. 2017;46(5):1464–1482. doi: 10.1039/C6CS00270F
- Theilacker C, Kropec A, Hammer F, et al. Protection against staphylococcus aureus by antibody to the polyglycerolphosphate backbone of heterologous lipoteichoic acid. J Infect Dis. 2012;205(7):1076–1085. doi: 10.1093/infdis/jis022
- Yi X-Y, Huang Z-X, Hou X-R, et al. Immunization with a peptide mimicking lipoteichoic acid protects mice against staphylococcus aureus infection. Vaccine. 2019;37(31):4325–4335. doi: 10.1016/j.vaccine.2019.06.024
- Balducci E, Papi F, Capialbi DE, et al. Polysaccharides’ structures and functions in biofilm architecture of antimicrobial-resistant (AMR) pathogens. Int J Mol Sci. 2023 Feb 17;24(4):4030. doi: 10.3390/ijms24044030
- Jennings LK, Storek KM, Ledvina HE, et al. Pel is a cationic exopolysaccharide that cross-links extracellular DNA in the Pseudomonas aeruginosa biofilm matrix. Proc Natl Acad Sci, USA. 2015 Sep 8;112(36):11353–11358. doi: 10.1073/pnas.1503058112
- Colvin KM, Irie Y, Tart CS, et al. The pel and Psl polysaccharides provide Pseudomonas aeruginosa structural redundancy within the biofilm matrix. Environ Microbiol. 2012 Aug;14(8):1913–1928. doi: 10.1111/j.1462-2920.2011.02657.x
- Mishra M, Byrd MS, Sergeant S, et al. Pseudomonas aeruginosa psl polysaccharide reduces neutrophil phagocytosis and the oxidative response by limiting complement-mediated opsonization. Cell Microbiol. 2012 Jan;14(1):95–106. doi: 10.1111/j.1462-5822.2011.01704.x
- DiGiandomenico A, Warrener P, Hamilton M, et al. Identification of broadly protective human antibodies to Pseudomonas aeruginosa exopolysaccharide psl by phenotypic screening. J Exp Med. 2012 Jul 2;209(7):1273–1287. doi: 10.1084/jem.20120033
- Ali SO, Yu XQ, Robbie GJ, et al. Phase 1 study of MEDI3902, an investigational anti-Pseudomonas aeruginosa PcrV and psl bispecific human monoclonal antibody, in healthy adults. Clin Microbiol Infect. 2019 May;25(5):.e629.1–.e629.6. doi: 10.1016/j.cmi.2018.08.004
- Ray VA, Hill PJ, Stover CK, et al. Anti-psl targeting of Pseudomonas aeruginosa biofilms for neutrophil-mediated disruption. Sci Rep. 2017 Nov 22;7(1):16065. doi: 10.1038/s41598-017-16215-6
- Skurnik D, Cywes-Bentley C, Pier GB. The exceptionally broad-based potential of active and passive vaccination targeting the conserved microbial surface polysaccharide PNAG. Expert Rev Vaccines. 2016 Aug;15(8):1041–1053. doi: 10.1586/14760584.2016.1159135
- Maira-Litrán T, Kropec A, Abeygunawardana C, et al. Immunochemical properties of the staphylococcal poly-N-acetylglucosamine surface polysaccharide. Infect Immun. 2002 Aug;70(8):4433–4440. doi: 10.1128/IAI.70.8.4433-4440.2002
- Maira-Litrán T, Kropec A, Goldmann DA, et al. Comparative opsonic and protective activities of staphylococcus aureus conjugate vaccines containing native or deacetylated staphylococcal poly-N-acetyl-beta-(1-6)-glucosamine. Infect Immun. 2005 Oct;73(10):6752–6762. doi: 10.1128/IAI.73.10.6752-6762.2005
- Maira-Litran T, Kropec A, Goldmann D, et al. Biologic properties and vaccine potential of the staphylococcal poly-N-acetyl glucosamine surface polysaccharide. Vaccine. 2004;22(7):872–879. doi: 10.1016/j.vaccine.2003.11.033
- Pozzi C, Waters EM, Rudkin JK, et al. Methicillin resistance alters the biofilm phenotype and attenuates virulence in staphylococcus aureus device-associated infections. PLOS Pathog. 2012;8(4):e1002626. doi: 10.1371/journal.ppat.1002626
- Gening ML, Pier GB, Nifantiev NE. Broadly protective semi-synthetic glycoconjugate vaccine against pathogens capable of producing poly-β-(1→6)-N-acetyl-d-glucosamine exopolysaccharide. Drug Discov Today Technol. 2020;35-36:13–21. doi: 10.1016/j.ddtec.2020.09.002
- Soliman C, Walduck AK, Yuriev E, et al. Structural basis for antibody targeting of the broadly expressed microbial polysaccharide poly-N-acetylglucosamine. J Biol Chem. 2018;293(14):5079–5089. doi: 10.1074/jbc.RA117.001170
- Bentancor LV, O’Malley JM, Bozkurt-Guzel C, et al. Poly-N-acetyl-β-(1-6)-glucosamine is a target for protective immunity against acinetobacter baumannii infections. Infect Immun. 2012 Feb;80(2):651–656. doi: 10.1128/IAI.05653-11
- McDonald ND, Boyd EF. Structural and biosynthetic diversity of Nonulosonic acids (NulOs) that decorate surface structures in bacteria. Trends Microbiol. 2021;29(2):142–157. doi: 10.1016/j.tim.2020.08.002
- Berti F, Adamo R. Recent mechanistic insights on glycoconjugate vaccines and future perspectives.ACS Chem Biol. 2013;8(8):1653–1663. doi: 10.1021/cb400423g
- Kenyon JJ, Kasimova AA, Notaro A, et al. Acinetobacter baumannii K13 and K73 capsular polysaccharides differ only in K-unit side branches of novel non-2-ulosonic acids: di-N-acetylated forms of either acinetaminic acid or 8-epiacinetaminic acid. Carbohydr Res. 2017 Nov 27;452:149–155. doi: 10.1016/j.carres.2017.10.005
- Weiner LM, Webb AK, Limbago B, et al. Antimicrobial-resistant pathogens associated with healthcare-associated infections: summary of data reported to the national healthcare Safety network at the Centers for Disease Control and Prevention, 2011–2014. Infect Control Hosp Epidemiol. 2016;37(11):1288–1301. doi: 10.1017/ice.2016.174
- Weiner-Lastinger LM, Abner S, Edwards JR, et al. Antimicrobial-resistant pathogens associated with adult healthcare-associated infections: summary of data reported to the national healthcare Safety network, 2015-2017. Infect Control Hosp Epidemiol. 2020 Jan;41(1):1–18. doi: 10.1017/ice.2019.296
- Bonten M, Johnson JR, van den Biggelaar AHJ, et al. Epidemiology of Escherichia coli Bacteremia: A Systematic Literature Review. Clin Infect Dis. 2021 Apr 8;72(7):1211–1219. doi: 10.1093/cid/ciaa210
- Liu Y, Zhu M, Fu X, et al. Escherichia coli causing neonatal meningitis during 2001-2020: a study in Eastern China. Int J Gen Med. 2021;14:3007–3016. doi: 10.2147/IJGM.S317299
- Zhang W, Wang Q, Zhang L, et al. Comparison of epidemiological characteristics between ESBL and non-ESBL isolates of clinically isolated Escherichia coli from 2014 to 2022: a single-center study. Infect Drug Resist. 2023;16(null):5185–5195. doi: 10.2147/IDR.S414079
- Kumar CK, Sands K, Walsh TR, et al. Global, regional, and national estimates of the impact of a maternal Klebsiella pneumoniae vaccine: a bayesian modeling analysis. PLOS Med. 2023;20(5):e1004239. doi: 10.1371/journal.pmed.1004239
- Russo TA, Beanan JM, Olson R, et al. Capsular polysaccharide and the O-specific antigen impede antibody binding: a potential obstacle for the successful development of an extraintestinal pathogenic Escherichia coli vaccine. Vaccine. 2009 Jan 14;27(3):388–395. doi: 10.1016/j.vaccine.2008.10.082
- Wantuch PL, Knoot CJ, Robinson LS, et al. Capsular polysaccharide inhibits vaccine-induced O-antigen antibody binding and function across both classical and hypervirulent K2: O1 strains of Klebsiella pneumoniae. PLOS Pathog. 2023;19(5):e1011367. doi: 10.1371/journal.ppat.1011367
- Wells TJ, Whitters D, Sevastsyanovich YR, et al. Increased severity of respiratory infections associated with elevated anti-LPS IgG2 which inhibits serum bactericidal killing. J Exp Med. 2014;211(9):1893–1904. doi: 10.1084/jem.20132444
- Thaden JT, Keller AE, Shire NJ, et al. Pseudomonas aeruginosa bacteremic patients exhibit nonprotective antibody titers against therapeutic antibody targets PcrV and Psl Exopolysaccharide. J Infect Dis. 2015;213(4):640–648. doi: 10.1093/infdis/jiv436
- Hendriks A, Kerkman PF, Varkila MRJ, et al. Glycan-specific IgM is critical for human immunity to staphylococcus aureus. bioRxiv. 2023; 548956 doi: 10.1242/prelights.35297
- Mbaeyi SAB, Catherine H, Duffy J, et al. Meningococcal vaccination: recommendations of the advisory committee on immunization practices, United States, 2020. MMWR Recomm Rep. 2020;69(No. RR–9):1–41. doi: 10.15585/mmwr.rr6909a1
- Buonsanti C, Balocchi C, Harfouche C, et al. Novel adjuvant Alum-TLR7 significantly potentiates immune response to glycoconjugate vaccines. Sci Rep. 2016 Jul 21;6(1):29063. doi: 10.1038/srep29063
- Avci F, Berti F, Dull P, et al. Glycoconjugates: what it would take to master these well-known yet little-understood immunogens for vaccine development. mSphere. 2019 Sep 25;4(5). doi: 10.1128/mSphere.00520-19
- Pichichero ME. Protein carriers of conjugate vaccines.Hum Vaccines Immunother. 2013;9(12):2505–2523. doi: 10.4161/hv.26109
- Pöllabauer EM, Petermann R, Ehrlich HJ. The influence of carrier protein on the immunogenicity of simultaneously administered conjugate vaccines in infants. Vaccine. 2009 Mar 10;27(11):1674–1679. doi: 10.1016/j.vaccine.2009.01.005