ABSTRACT
Introduction
Malaria continues to remain a major global health problem with nearly a quarter of a billion clinical cases and more than 600,000 deaths in 2022. There has been significant progress toward vaccine development, however, poor efficacy of approved vaccines requiring multiple immunizing doses emphasizes the need for continued efforts toward improved vaccines. Progress to date, nonetheless, has provided impetus for malaria elimination.
Areas covered
In this review we will focus on diverse immune mechanisms targeting gametocytes in the human host and gametocyte-mediated malaria transmission via the mosquito vector.
Expert opinion
To march toward the goal of malaria elimination it will be critical to target the process of malaria transmission by mosquitoes, mediated exclusively by the sexual stages, i.e. male, and female gametocytes, ingested from infected vertebrate host. Studies over several decades have established antigens in the parasite sexual stages developing in the mosquito midgut as attractive targets for the development of transmission blocking vaccines (TBVs). Immune clearance of gametocytes in the vertebrate host can synergize with TBVs and directly aid in maintaining effective transmission reducing immune potential.
1. Introduction
Malaria caused by Plasmodium falciparum (P. falciparum) and Plasmodium vivax (P. vivax) accounts for >90% of all malaria cases globally [Citation1]. According to the World Malaria Report there were 247 million new cases and 608,000 malaria deaths reported worldwide in 2022 [Citation1]. Anti-malaria drugs currently in use are gradually losing their therapeutic efficacy due to development of resistance to multiple antimalarial drugs, and the mosquito vectors are becoming resistant to insecticides. These factors underscore the need for developing effective malaria vaccine(s) to help with the long-term goal of malaria elimination and eradication [Citation2]. The malaria vaccine, MosquirixTM (RTS,S/ASO1), based on P. falciparum circumsporozoite protein (PfCSP) advanced through phase III trials and approved by the WHO in 2021 has shown < 40% protective efficacy. More recently, another vaccine targeting infection by sporozoites, R21/Matrix-M, has shown improved efficacy, but both MosquirixTM and R21/Matrix-M require multiple vaccine doses which lead to practical challenges [Citation3–6]. Such challenges highlight the intricacies of vaccine development and stress continued vaccine discovery efforts toward better efficacious products to realize the goal of malaria elimination via transmission reduction. In particular, vaccines directly targeting transmission of malaria are viewed as a valuable tool for malaria elimination. A better understanding of the immunobiology of host-parasite interactions to develop effective vaccines for malaria represents a key research gap that needs to be explored further [Citation7,Citation8].
2. Malaria transmission biology
Malaria transmission represents dynamic exchange of parasites between a vertebrate host and Anopheles mosquito vector (). Sporozoites inoculated by an infected mosquito during blood feeding process invade hepatocytes leading to the production of asexually reproducing erythrocytic forms of the Plasmodium parasites which are responsible for symptoms and pathology of malaria. Transmission of malaria, however, depends on the development and survival in humans of mature intra-erythrocytic sexual stage parasites (male and female gametocytes also known as micro and macro gametocytes, respectively). Sexually differentiated parasites undergo maturation through morphologically distinct stages including round form (stage I), developing half-moon (stage II), elongated with convex ends (stage III), pointed spindle form (stage IV), and finally a crescent form with rounded ends (stage V). Mature stage V male and female gametocytes mediate transmission involving further complex sexual development of parasites in the mosquitoes [Citation9]. Immature stages II-IV are sequestered in the bone marrow and spleen and mature stage V gametocytes are observed in the blood circulation where they are available for ingestion by blood feeding female anopheline mosquitoes [Citation10,Citation11]. Gametocytes ingested by a female anopheline mosquito during a blood meal initiate a cascade of events within the mosquito midgut leading to sexual reproduction and formation of sporozoites which are reintroduced to the human host to complete the transmission cycle.
Figure 1. Malaria transmission cycles.
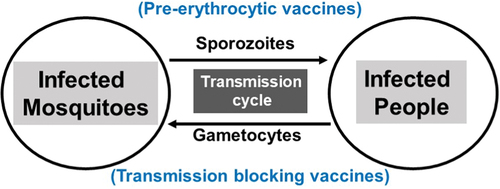
Sexual differentiation of asexually proliferating erythrocytic parasites and developmental maturation of male and female gametocytes is central to overall malaria transmission. Sexual stages (immature and mature gametocytes) and stages in the mosquito midgut present numerous immune targets to effectively reduce malaria transmission, a key goal for elimination of malaria at the population level. The infectiousness of gametocytes is determined by various parasitological (gametocyte density, ratio and maturity of male and female gametocytes, asexual density), entomologic (mosquito species, age, and environmental), and immunologic (innate and adaptive immunity against specific antigens) factors [Citation12,Citation13].
3. Immunity against malaria
Partially effective ‘clinical immunity’ to malaria develops in individuals who have undergone repeated exposure to parasite-derived antigens during malaria infection, but it does not prevent reinfection, and in most cases, it leads to asymptomatic infections [Citation13–17]. Both innate and adaptive immune responses are directed against antigens in the asexual stages and studies have shown the presence of antibodies recognizing stage-specific parasite antigens in the sera of malaria exposed individuals [Citation18,Citation19]. Some of these antigens recognized by antibodies in immune sera are important for invasion of erythrocytes by asexual stage merozoites while others are responsible for sequestration, the cytoadherence of infected erythrocytes containing trophozoites and schizonts (late developmental stages) to the endothelium of capillaries and venules [Citation18–20].
Non-opsonic (direct) phagocytosis and opsonic (antibody and complement-mediated) phagocytosis by macrophages and/or dendritic cells are two innate immune mechanisms involved in the initial clearance of infecting pathogens and for presentation of antigens for the induction of adaptive immune responses [Citation21–23]. Activation of circulating monocytes and tissue resident macrophages by parasite antigens may be critical responses against Plasmodium-infected erythrocytes [Citation24,Citation25]. However, a comprehensive delineation of steps in such early responses in malaria remains to be fully explored, and this knowledge will aid in understanding the development of immunity to the distinct stages of the parasite in the human host. Several studies suggest that opsonization by antibodies produced during natural infection followed by phagocytosis and direct phagocytosis (without antibodies) of various erythrocytic forms (asexual and immature sexual stages) and free merozoite does occur [Citation26–31]. Numerous published studies have established that antigens expressed in P. falciparum infected erythrocyte (represented as ‘A-F’ in ) are important targets of immune responses that function by: (i) opsonizing parasitized erythrocytes and clearance by monocytes and macrophages and (ii) inhibiting sequestration and rosetting of infected erythrocytes [Citation32]. In the blood stage parasites, many surface proteins (PfEMP1, RIFIN, STEVOR and SURFIN, etc.) encoded by multigene family have been identified as mediators of sequestration and antigenic variation [Citation32–35]. Likewise, antigens expressed in gametocytes have also been shown to elicit antibody responses during natural infection and the antibody levels increase with repeat transmission cycles [Citation36,Citation37] and may effectively block parasite development in the mosquito midgut [Citation38–43]. Understanding innate and adaptive immune mechanisms will be critical for the identification of specific targets and for the development of vaccines aimed at reducing malaria infection or clinical disease or parasite transmission through Anopheles mosquitoes [Citation12,Citation44].
Figure 2. Schematic representation of immune targets in gametocyte infected red blood cells (GiRBC).
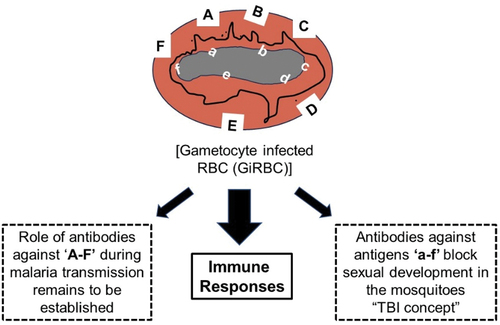
Strategies to interrupt transmission are of unique significance for their direct role in malaria elimination efforts. Several stage-specific antigens produced by circulating gametocyte infected red blood cells (GiRBC) have been identified as targets of immune responses during natural infection. These antibodies recognize well-established antigens (P230, P48/45 and P47) represented as ‘a-f,’ expressed in sexually developing parasites and on the plasma membrane of the intracellular parasite (). Antibodies against these antigens when ingested in the blood meal have been shown to effectively reduce sexual development of parasites in the mosquito midgut [Citation45]. Additionally, proteins represented as ‘A-F’ may also be present on the surface of immature (imGiRBC) and mature (mGiRBC) gametocytes and may be targets of antibodies induced during natural infection (). However, studies are needed to characterize such surface proteins and their potential role in immunity against malaria transmission [Citation46–50]. Unlike asexual stages, protein trafficking mechanisms in erythrocytic sexual stages have not been explored, however it is expected that gametocytes will deploy similar mechanisms to display unique surface proteins represented as ‘A-F’ on GiRBC surface (). In the erythrocytic asexual stages a protein translocation export complex (PTEX) has been identified playing an essential role in the translocation of proteins across parasite plasma membrane and parasitophorous vacuolar membrane [Citation51]. Using flow cytometry, a screen of human serum samples from malaria infected patients provided direct preliminary evidence for recognition of antigens on the surface of GiRBC, termed as gametocyte surface antigens (GSA). Their molecular identity is not known nor is known any functional significance of these GSAs [Citation48,Citation49]. While imGiRBC have been shown to undergo phagocytosis, mGiRBC are otherwise refractory to phagocytosis in vitro as demonstrated [Citation52]. It can be hypothesized that GiRBC surface antigens will be potential targets for opsonization by antibodies elicited during natural infection in people and facilitate phagocytic clearance of GiRBC. Identification of antigens on imGiRBC and mGiRBC surface may provide unique targets for stopping malaria transmission via opsonic phagocytosis of GiRBC even before they are ingested by the mosquito.
4. Gamete surface antigens of TBI
Some of the well-known target antigens (e.g. P230, P48/45 and P47) indicated as ‘a-f’ in are synthesized by GiRBC and expressed on the plasma membrane of male and female parasites enclosed within the parasitophorous vacuole of infected red blood cells. Previous studies have shown that the expression of these antigens begins in imGiRBC and continues through the maturation of intra-erythrocytic gametocytes [Citation53,Citation54]. Furthermore, after ingestion of gametocytes, these antigens persist on the surface of extracellular gametes (both male and female) and early zygotes formed upon fertilization in the mosquito midgut. These proteins belong to a 6-Cys protein family [Citation55,Citation56], form stable membrane bound complex [Citation57,Citation58], and have been shown to be critical for gamete fertility and fertilization between male and female gametes [Citation59–61]. A search for proteins facilitating gamete fusion has identified two proteins HAP2 and HAP2p expressed predominantly in male gametocytes and male gametes, and studies in different Plasmodium species have suggested their potential role in TBI [Citation62–65]. Antibodies against various gamete surface proteins ingested in the blood meal interfere with fertilization of gametes and provide the basis for transmission blocking vaccine (TBV) development. Antibodies against P230, P48/45 and P47 are elicited during natural infection and immuno-epidemiological studies have demonstrated a good correlation between antibodies against P230 and P48/45 and inhibition of sexual development in transmission blocking assays [Citation12,Citation45,Citation66]. These antigens or putative functional sub-fragments have been pursued intensely as targets for the development of a malaria transmission blocking vaccine [Citation67–72]. The fact that these antigens elicit immune responses during natural infection may also lead to boosting of vaccine-induced immune responses.
Additional targets of TBV identified are novel proteins expressed after fertilization between gametes. Development of zygotes into motile ookinetes is accompanied by gradual loss of gamete surface proteins and sequential expression of post-fertilization proteins, P25 and P28. Similar to gamete surface proteins, antibodies against P25 and P28 also interfere with the parasite development and are being pursued for TBV development [Citation73,Citation74]. In contrast to pre-fertilization antigens (P230, P48/45 and P47), the expression of post-fertilization antigens P25 and P28 occurs only in the parasite stages developing after ingestion of gametocytes by mosquitoes. While P25 and P28 are not presented to the immune system during natural infection, the presence of antibodies (monoclonal or elicited by immunization) against these antigens, in particular P25, has been shown to effectively block mosquito midgut stage parasite development, most likely by interfering with the development of ookinetes. Lack of natural immune responses against P25 and P28 during infection, however, implies that a vaccine based on these antigens will require periodic booster immunizations. All the above antigens (pre- and post-fertilization) are being explored individually or in combination for vaccine development using various vaccine platforms including recombinant proteins formulated with adjuvants [Citation12,Citation45,Citation75], DNA plasmids [Citation76–79], and more recently mRNA-LNP formulation [Citation80,Citation81]. Pfs25 and Pfs230 in various adjuvant formulations have entered phase I clinical trials with encouraging functional outcomes [Citation82–86]. Phase I clinical trial of a plant derived Pfs25 virus-like particle in naïve human volunteers demonstrated weak transmission blocking activity [Citation82]. Conjugation of Pfs25 with an ExoProtein A (EPA) of Pseudomonas aeruginosa revealed improved functional activity in malaria naïve volunteers [Citation83]. however, the immunity was short lived and required more than 3 booster doses [Citation84]. More recently, a clinical trial of EPA conjugated Pfs230 (domain D1) revealed stronger transmission blocking activity as compared to EPA conjugated Pfs25, both formulated with alhydrogel as an adjuvant [Citation85].
5. GiRBC surface antigens as potential targets for TBI
Proteins expressed on the surface of asexual stage parasites (PfEMP-1, STEVOR, RIFIN, and SURFIN family) have been shown to play an active role in parasite sequestration via cytoadherence to endothelial cells in microvasculature. Unlike asexual stages, much less is known about protein expression on the surface of GiRBC. This is especially important because imGiRBC remain sequestered in the bone marrow and the spleen, and it is expected that parasite surface antigens must be involved in mediating such sequestration [Citation10,Citation87–91]. It is logical to rationalize that sequestration of imGiRBCs in the bone marrow and spleen facilitates their survival allowing them to evade phagocytic clearance. In their sequestered locations, imGiRBCs successfully complete developmental maturation into mGiRBCs. Upon reaching maturity, transmission competent mature gametocytes (mGiRBC) are freed from their sequestered location into blood circulation to maximize their chances of being ingested by blood seeking Anopheles mosquitoes to mediate transmission.
Previous studies have reported CD36-mediated non-opsonic phagocytosis of imGiRBC by monocytes and macrophages that potentially involves interaction between CD36 on phagocytes and PfEMP1 on imGiRBC surface [Citation31,Citation35,Citation92,Citation93]. In contrast, mGiRBC crucial for transmission were shown to resist direct phagocytosis [Citation52,Citation94], possibly providing a transmission advantage to the parasite. On the other hand, killed mature GiRBC or gametocyte freed by lysing red blood cell membrane by mild saponin treatment were effectively phagocytosed [Citation52]. These observations further emphasize continued efforts to characterize proteins expressed on the surface of mGiRBC, previously described as GSA. Antibodies (elicited during natural infection or by vaccination) targeting GSA on mGiRBCs may promote opsonic phagocytic clearance of transmission competent mature gametocytes. A few studies [Citation49,Citation95] have reported the presence of antibodies in people recognizing proteins on the surface of mGiRBC (stage V gametocytes). However, further studies have been unsuccessful in defining a role for such GSA. It was postulated that these antibodies might account for clearance of gametocytes during repeated malaria exposure [Citation46].
Other studies [Citation96] have described antibodies elicited during natural infection which exhibited binding to live imGiRBC, causing distortion in morphology of developing gametocytes and inhibiting formation of mature gametocytes. It is conceivable that such malformed circulating gametocytes could be readily cleared by phagocytic uptake by macrophages. Antigens recognized by such antibodies are not known, however, they are likely to represent targets of innate and adaptive immunity indicated as ‘A-F’ in . Using biochemical and forward and reverse genetic approaches a set of proteins termed PfGEXPs (P. falciparum gametocyte exported proteins [Citation97–99] have been identified which are expressed during gametocyte host cell remodeling and early sexual differentiation. It has been suggested that these proteins might mediate sequestration of imGiRBC in the bone marrow and the spleen during infection. Previous transcriptomic and proteomic studies using cultured-adapted and a few clinical isolates have identified a few dozen specific genes expressed in developing imGiRBC. The biological role of these stage-specific gene products remains to be explored. In limited comparative analysis of gene expression patterns, the lab adapted parasites were found to be more diverse than clinical isolates and more studies are needed to define gametocyte stage-specific genes for further evaluation as TBI targets [Citation100]. Immune clearance of imGiRBC and mGiRBC will effectively reduce transmission potential (i.e. transmission of parasites to mosquitoes). The identification of potential new targets on imGiRBC and mGiRBC which may be targets of opsonic phagocytosis and immunity targeting transmission stages of Plasmodium remains a gap area in need of further investigation.
6. Multiple targets for immune intervention against malaria transmission
In P. falciparum infection, mature gametocytes circulate for longer periods [Citation101–105], and may continue to infect mosquitoes, unless targeted by cellular and humoral immune responses. Blocking the development and maturation of gametocytes in the vertebrate host, promoting immune clearance by phagocytic mechanisms, and interfering with sexual reproduction in the mosquito midgut all represent targets for intervention of malaria transmission. In addition to antibodies affecting gametocyte maturation, other immune components, e.g. complement factors, IFNγ and TNFα, have also been shown to kill erythrocytic stages [Citation106–109]. Additionally, T cell-mediated mechanisms were shown to effectively reduce gametocytes early during infection in P. yoelii nigeriensis murine malaria model which when combined with specific antibodies resulted in complete transmission blocking immunity [Citation110,Citation111]. The potential role for antibody-independent mechanisms was likewise demonstrated against the sexual stages of P. falciparum [Citation112].
provides a schematic representation of various stages during development and maturation of gametocytes as potential targets for immune intervention leading to reduced malaria transmission. Pre-existing or vaccine-induced antibodies against GSA in imGiRBC and mGiRBC may block entry of immature intracellular sexual stages into bone marrow resulting in reduced sequestration and phagocytic clearance of imGiRBC and mGiRBC contributing to overall transmission blocking immunity. Such immune clearance of gametocytes combined with antibodies targeting sexual development of parasites in the mosquito midgut is expected to effectively reduce overall malaria transmission potential.
Figure 3. Proposed immunologic role for antibodies directed against antigens represented as ‘A-F’ and ‘a-f’.
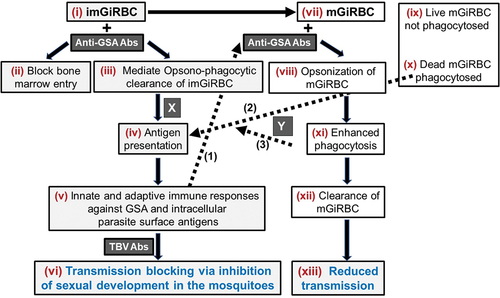
Future research efforts may benefit from a focus on evaluation of the validated parasite molecules recognized by opsonizing antibodies for the development of anti-gametocyte vaccines, effective for reducing malaria transmission. Similarly, antibody-independent immune mechanisms targeting GiRBC survival may also need to be reexamined for effectively targeting malaria transmission. Detailed investigations of immune responses against imGiRBC and mGiRBC will inform the field about the role of opsonic and non-opsonic phagocytosis in the clearance of circulating gametocytes. They will also provide critical data to evaluate malaria exposure-dependent transmission reducing immune potential of antibodies in field studies, and how to increase effective titers of such antibodies.
7. Expert opinion – further considerations for vaccine design
A TBV is expected to play a critical role in realizing the goal of malaria elimination and eradication. In addition to evaluating each identified antigen as a vaccine candidate, it may be important to combine vaccine antigens targeting parasite transmission stages at different life cycle points [Citation78–80]. A vaccine cocktail may comprise antigens eliciting antibodies facilitating phagocytic clearance of GiRBC on one hand and those mediating transmission reduction via blocking pre-and post-fertilization development of parasites in the mosquito midgut ().
Transmission reducing antibodies elicited during natural infection or by immunization have provided the impetus for the development of efficacious vaccine to reduce transmission at the population level. Several studies have also demonstrated the presence of transmission enhancing along with transmission reducing antibodies elicited during natural infection [Citation113]. These reported opposite outcomes were observed with antisera from P. vivax-infected humans and monoclonal antibodies directed against surface proteins in the gametes of P. vivax. Higher concentration of antibodies resulted in blocking whereas lower concentration of the same antibody revealed 2- to 4- fold transmission enhancement [Citation113]. In addition to dose-dependence, an antigen may also present different epitopes which elicit antibodies exhibiting either blocking or enhancing effects [Citation114]. Antibody-mediated enhancement of infection has also been reported for P. falciparum [Citation12,Citation115,Citation116], other animal malaria parasites [Citation117,Citation118] and viruses [Citation119].
The goal of vaccine development is to optimize protective responses and minimize immunopathogenic outcomes. These dual outcomes raise biologically significant questions regarding vaccine design and formulations. More studies exploring the breadth, relative proportions and transmission-blocking versus transmission-enhancing antibodies during immune responses elicited by natural infection as well as during vaccination using different vaccine delivery platforms will have important implications for designing effective vaccines [Citation116]. Systematic evaluation of these features may reveal ways to improve protective efficacy of vaccines by either switching vaccine platforms or by designing vaccine molecules for immune-focusing responses against blocking epitopes by omitting deleterious enhancing epitopes.
Article highlights
Immune targeting of sexual development of malaria parasites in the mosquito by vaccines represents a key approach to reduce malaria transmission.
Antigens expressed on the surface of gametocyte infected red blood cells (GiRBC) can provide novel opportunities to target immune clearance of GiRBC and reduce overall malaria transmission.
Innate and adaptive responses against a combination of antigens on GiRBC and gametes surfaces can offer additive or synergistic mechanisms for optimum efficacy.
Various vaccine platforms including adjuvanted proteins, DNA plasmid and mRNA-LNP can elicit cellular and humoral immune response to target antigens on GiRBC and gametes.
Structural characterization of epitopes can provide a rational approach to design potent protective vaccine while minimizing immune-enhancing and immuno-pathogenic responses.
Declaration of interest
The authors have no relevant affiliations or financial involvement with any organization or entity with a financial interest in or financial conflict with the subject matter or materials discussed in the manuscript. This includes employment, consultancies, honoraria, stock ownership or options, expert testimony, grants, patents received or pending, or royalties.
Reviewer disclosures
Peer reviewers on this manuscript have no relevant financial or other relationships to disclose.
Author contributions
Both the authors contributed equally to the intellectual conception, writing and design of review article and interpreting the relevant literature.
Acknowledgments
We acknowledge many excellent reviews published on immunobiology of gametocytes. For the purpose of this publication, we have cited a few representative references, and it does not represent a comprehensive citation of literature.
Additional information
Funding
References
- WHO-Malaria-Report. World malaria report. 2022; –1.
- Jagannathan P, Kakuru A. Malaria in 2022: increasing challenges, cautious optimism. Nat Commun. 2022 May 13;13(1):2678. doi: 10.1038/s41467-022-30133-w
- Zavala F. RTS, S: the first malaria vaccine. J Clin Invest. 2022 Jan 4;132(1). doi: 10.1172/JCI156588
- Nkomba NN, Cairo C, Laufer MK. RTS, S today and tomorrow’s science. Cell Host & Microbe. 2022;30(5):604–606. doi: 10.1016/j.chom.2022.04.004
- Moorthy V, Binka F. R21/Matrix-M: a second malaria vaccine? Lancet. 2021 May 15;397(10287):1782–1783. doi: 10.1016/S0140-6736(21)01065-5
- Hammershaimb EA, Berry AA. Pre-erythrocytic malaria vaccines: RTS,S, R21, and beyond. Expert Rev Vaccines. 2024 Jan;23(1):49–52. doi: 10.1080/14760584.2023.2292204
- Duffy PE. Making a good malaria vaccine better. Trends Parasitol. 2022 Jan 01;38(1):9–10. doi: 10.1016/j.pt.2021.11.006
- Duffy PE. Current approaches to malaria vaccines. Curr Opin Microbiol. 2022 Dec;70:102227. doi: 10.1016/j.mib.2022.102227
- Carter R, Miller LH. Evidence for environmental modulation of gametocytogenesis inPlasmodium falciparum in continuous culture. Bull WHO. 1979;57(Suppl 1):37–52.
- De Niz M, Meibalan E, Mejia P, et al. Plasmodium gametocytes display homing and vascular transmigration in the host bone marrow. Sci Adv. 2018;4(5):eaat3775. doi: 10.1126/sciadv.aat3775
- Venugopal K, Hentzschel F, Valkiūnas G, et al. Plasmodium asexual growth and sexual development in the haematopoietic niche of the host. Nature Rev Microbiol. 2020;18(3):177–189. doi: 10.1038/s41579-019-0306-2
- de Jong RM, Tebeje SK, Meerstein-Kessel L, et al. Immunity against sexual stage Plasmodium falciparum and Plasmodium vivax parasites. Immunol Rev. 2020;293(1):190–215. doi: 10.1111/imr.12828
- Drakeley C, Sutherland C, Bousema J, et al. The epidemiology of Plasmodium falciparum gametocytes: weapons of mass dispersion. Trends Parasitol. 2006;22(9):424–430. doi: 10.1016/j.pt.2006.07.001
- Crompton PD, Moebius J, Portugal S, et al. Malaria immunity in man and mosquito: insights into unsolved mysteries of a deadly infectious disease. Annu Rev Immunol. 2014;32(1):157. doi: 10.1146/annurev-immunol-032713-120220
- Baird JK. Host age as a determinant of naturally acquired immunity to Plasmodium falciparum. Parasitol Today. 1995;11(3):105–111. doi: 10.1016/0169-4758(95)80167-7
- Baird JK, Krisin BM, Elyazar IRF, et al. Onset of clinical immunity to Plasmodium falciparum among Javanese migrants to Indonesian Papua. Ann Trop Med Parasitol. 2003;97(6):557–564. doi: 10.1179/000349803225001472
- Doolan DL, Dobaño C, Baird JK. Acquired immunity to malaria. Clinical Microbiology Reviews. 2009;22(1):13–36. doi: 10.1128/CMR.00025-08
- Marsh K, Kinyanjui S. Immune effector mechanisms in malaria. Parasite Immunol. 2006;28(1‐2):51–60. doi: 10.1111/j.1365-3024.2006.00808.x
- Kinyanjui SM, Bull P, Newbold CI, et al. Kinetics of antibody responses to Plasmodium falciparum–infected erythrocyte variant surface antigens. J Infect Dis. 2003;187(4):667–674. doi: 10.1086/373994
- David PH, Hommel M, Miller LH, et al. Parasite sequestration in Plasmodium falciparum malaria: spleen and antibody modulation of cytoadherence of infected erythrocytes. Proc Natl Acad Sci U S A. 1983 Aug;80(16):5075–5079. doi: 10.1073/pnas.80.16.5075
- Brown GC. Cell death by phagocytosis. Nat Rev Immunol. 2023 Aug 21;24(2):91–102. doi: 10.1038/s41577-023-00921-6
- Cohen HB, Mosser DM. Extrinsic and intrinsic control of macrophage inflammatory responses. J Leukoc Biol. 2013;94(5):913–919. doi: 10.1189/jlb.0413236
- Park MD, Silvin A, Ginhoux F, et al. Macrophages in health and disease. Cell. 2022;185(23):4259–4279. doi: 10.1016/j.cell.2022.10.007
- Gowda DC, Wu X. Parasite recognition and signaling mechanisms in innate immune responses to malaria. Front Immunol. 2018;9:3006. doi: 10.3389/fimmu.2018.03006
- Leitner WW, Haraway M, Pierson T, et al. Role of opsonophagocytosis in immune protection against malaria. Vaccines. 2020;8(2):264. doi: 10.3390/vaccines8020264
- Ayi K, Turrini F, Piga A, et al. Enhanced phagocytosis of ring-parasitized mutant erythrocytes: a common mechanism that may explain protection against falciparum malaria in sickle trait and beta-thalassemia trait. Blood. 2004;104(10):3364–3371. doi: 10.1182/blood-2003-11-3820
- Ayi K, Patel SN, Serghides L, et al. Nonopsonic phagocytosis of erythrocytes infected with ring-stage Plasmodium falciparum. Infect Immun. 2005;73(4):2559–2563. doi: 10.1128/IAI.73.4.2559-2563.2005
- Ayi K, Lu Z, Serghides L, et al. CD47-SIRPα interactions regulate macrophage uptake of Plasmodium falciparum-infected erythrocytes and clearance of malaria in vivo. Infect Immun. 2016 Jul 1;84(7):2002–2011. doi: 10.1128/IAI.01426-15
- Kumaratilake LM, Ferrante A. Opsonization and phagocytosis of Plasmodium falciparum merozoites measured by flow cytometry. Clin Diagn Lab Immunol. 2000;7(1):9–13. doi: 10.1128/CDLI.7.1.9-13.2000
- Ferrante A, Kumaratilake L, Rzepczyk C, et al. Killing of Plasmodium falciparum by cytokine activated effector cells (neutrophils and macrophages). Immunol Lett. 1990;25(1–3):179–187. doi: 10.1016/0165-2478(90)90112-4
- Smith TG, Serghides L, Patel SN, et al. CD36-mediated nonopsonic phagocytosis of erythrocytes infected with stage I and IIA gametocytes of Plasmodium falciparum. Infect Immun. 2003;71(1):393–400. doi: 10.1128/IAI.71.1.393-400.2003.
- Bull PC, Berriman M, Kyes S, et al. Plasmodium falciparum variant surface antigen expression patterns during malaria. PLOS Pathogens. 2005;1(3):e26. doi: 10.1371/journal.ppat.0010026
- McRobert L, Preiser P, Sharp S, et al. Distinct trafficking and localization of STEVOR proteins in three stages of the Plasmodium falciparum life cycle. Infect Immun. 2004;72(11):6597–6602. doi: 10.1128/IAI.72.11.6597-6602.2004
- Roberts DJ, Craig BAR, Pinches R, et al. Rapid switching to multiple antigenic and adhesive phenotypes in malaria. Nature. 1992;357(6380):689–692. doi: 10.1038/357689a0
- Rogers NJ, Hall BS, Obiero J, et al. A model for sequestration of the transmission stages of Plasmodium falciparum: adhesion of gametocyte-infected erythrocytes to human bone marrow cells. Infect Immun. 2000;68(6):3455–3462. doi: 10.1128/IAI.68.6.3455-3462.2000
- Stone WJR, Dantzler KW, Nilsson SK, et al. Naturally acquired immunity to sexual stage P. falciparum parasites. Parasitology. 2016;143(2):187–198. doi: 10.1017/S0031182015001341
- Stone WJR, Campo JJ, Ouédraogo AL, et al. Unravelling the immune signature of Plasmodium falciparum transmission-reducing immunity. Nat Commun. 2018 Feb 08;9(1):558. doi: 10.1038/s41467-017-02646-2
- Gwadz R, Green I. Malaria immunization in rhesus monkeys. A vaccine effective against both the sexual and asexual stages of Plasmodium knowlesi. J Exp Med. 1978;148(5):1311–1323. doi: 10.1084/jem.148.5.1311
- Gwadz R. Malaria: successful immunization against the sexual stages of Plasmodium gallinaceum. Science. 1976;193(4258):1150–1151. doi: 10.1126/science.959832
- Carter R, Gwadz RW, McAuliffe FM. Plasmodium gallinaceum: transmission-blocking immunity in chickens: I. Comparative immunogenicity of gametocyte- and gamete-containing preparations. Exp Parasitol. 1979;47(2):185–193. doi: 10.1016/0014-4894(79)90072-9
- Carter R, Gwadz RW, Green I. Plasmodium gallinaceum: transmission-blocking immunity in chickens: II. The effect of antigamete antibodies in vitro and in vivo and their elaboration during infection. Exp Parasitol. 1979;47(2):194–208. doi: 10.1016/0014-4894(79)90073-0
- Carter R, Chen D. Malaria transmission blocked by immunisation with gametes of the malaria parasite. Nature. 1976;263(5572):57–60. doi: 10.1038/263057a0
- Carter R, Miller LH, Culleton R. On malaria transmission and transmission blocking immunity. Am J Trop Med Hyg. 2022;tpmd211319–tpmd211319. doi: 10.4269/ajtmh.21-1319
- Rajneesh TR, Singh VK, Kumar A, et al. Advancements and challenges in developing malaria vaccines: targeting multiple stages of the parasite life cycle. ACS Infect Dis. 2023;9(10):1795–1814. doi: 10.1021/acsinfecdis.3c00332
- Wu Y, Sinden RE, Churcher TS, et al. Chapter three-development of malaria transmission-blocking vaccines: from concept to product. Adv Parasitol. 2015;89:109–152.
- Sutherland C. Surface antigens of Plasmodium falciparum gametocytes—A new class of transmission-blocking vaccine targets? Molecular and Biochemical Parasitology. 2009;166(2):93–98. doi: 10.1016/j.molbiopara.2009.03.007
- Dinko B, Bousema T, Sutherland CJ. Recognition of Plasmodium falciparum gametocyte surface antigens by plasma antibodies in asymptomatic Ghanaian school children. Malar J. 2012;11(S1):1–11. doi: 10.1186/1475-2875-11-S1-P24
- Dinko B, King E, Targett GA, et al. Antibody responses to surface antigens of Plasmodium falciparum gametocyte‐infected erythrocytes and their relation to gametocytaemia. Parasite Immunol. 2016;38(6):352–364. doi: 10.1111/pim.12323
- Saeed M, Roeffen W, Alexander N, et al. Plasmodium falciparum antigens on the surface of the gametocyte-infected erythrocyte. PLoS One. 2008;3(5):e2280. doi: 10.1371/journal.pone.0002280
- Gebru T, Ajua A, Theisen M, et al. Recognition of Plasmodium falciparum mature gametocyte-infected erythrocytes by antibodies of semi-immune adults and malaria-exposed children from Gabon [journal article]. Malaria j. 2017;16(1):176. doi: 10.1186/s12936-017-1827-7
- Haldar K. Protein trafficking in apicomplexan parasites: crossing the vacuolar Rubicon. Curr Opin Microbiol. 2016;32:38–45. doi: 10.1016/j.mib.2016.04.013
- Bansal GP, Weinstein CS, Kumar N. Insight into phagocytosis of mature sexual (gametocyte) stages of Plasmodium falciparum using a human monocyte cell line. Acta tropica. 2016 5;157:96–101. doi: 10.1016/j.actatropica.2016.01.033
- Kumar N, Carter R. Biosynthesis of the target antigens of antibodies blocking transmission of Plasmodium falciparum. Mol Biochem Parasitol. 1984;13(3):333–342. doi: 10.1016/0166-6851(84)90124-5
- Vermeulen AN, Van Deursen J, Brakenhoff RH, et al. Characterization of Plasmodium falciparum sexual stage antigens and their biosynthesis in synchronised gametocyte cultures. Mol Biochem Parasitol. 1986;20(2):155–163. doi: 10.1016/0166-6851(86)90027-7
- Arredondo SA, Cai M, Takayama Y, et al. Structure of the Plasmodium 6-cysteine s48/45 domain. Proc Nat Acad Sci. 2012;109(17):6692–6697. doi: 10.1073/pnas.1204363109
- Arredondo SA, Kappe SH. The s48/45 six-cysteine proteins: mediators of interaction throughout the Plasmodium life cycle. Int J Parasitol. 2017;47(7):409–423. doi: 10.1016/j.ijpara.2016.10.002
- Kumar N. Target antigens of malaria transmission blocking immunity exist as a stable membrane bound complex. Parasite Immunol. 1987 May;9(3):321–335. doi: 10.1111/j.1365-3024.1987.tb00511.x
- Kumar N, Wizel B. Further characterization of interactions between gamete surface antigens of Plasmodium falciparum. Mol Biochem Parasitol. 1992;53(1–2):113–120. doi: 10.1016/0166-6851(92)90013-A
- van Schaijk BC, van Dijk MR, van de Vegte-Bolmer M, et al. Pfs47, paralog of the male fertility factor Pfs48/45, is a female specific surface protein in Plasmodium falciparum. Mol Biochem Parasitol. 2006;149(2):216–222. doi: 10.1016/j.molbiopara.2006.05.015
- Van Dijk MR, Van Schaijk BC, Khan SM, et al. Three members of the 6-cys protein family of Plasmodium play a role in gamete fertility. PLOS Pathog. 2010;6(4):e1000853. doi: 10.1371/journal.ppat.1000853
- van Dijk MR, Janse CJ, Thompson J, et al. A central role for P48/45 in malaria parasite male gamete fertility. Cell. 2001;104(1):153–164. doi: 10.1016/S0092-8674(01)00199-4
- Blagborough AM, Sinden RE. Plasmodium berghei HAP2 induces strong malaria transmission-blocking immunity in vivo and in vitro. Vaccine. 2009;27(38):5187–5194. doi: 10.1016/j.vaccine.2009.06.069
- Angrisano F, Sala KA, Da DF, et al. Targeting the conserved fusion loop of HAP2 inhibits the transmission of plasmodium berghei and falciparum. Cell Rep. 2017;21(10):2868–2878. doi: 10.1016/j.celrep.2017.11.024
- Qiu Y, Zhao Y, Liu F, et al. Evaluation of Plasmodium vivax HAP2 as a transmission-blocking vaccine candidate. Vaccine. 2020;38(13):2841–2848. doi: 10.1016/j.vaccine.2020.02.011
- Kumar S, Valansi C, Haile MT, et al. Malaria parasites utilize two essential plasma membrane fusogens for gamete fertilization. Cell Mol Life Sci. 2022 Oct 14;79(11):549. doi: 10.1007/s00018-022-04583-w
- Duffy PE. Transmission-blocking vaccines: harnessing herd immunity for malaria elimination. Expert Rev Vaccines. 2021 Feb 01;20(2):185–198. doi: 10.1080/14760584.2021.1878028
- Singh SK, Plieskatt J, Chourasia BK, et al. Preclinical development of a Pfs230-Pfs48/45 chimeric malaria transmission-blocking vaccine. NPJ Vaccines. 2021;6(1):120. doi: 10.1038/s41541-021-00383-8
- Singh SK, Plieskatt J, Chourasia BK, et al. A reproducible and scalable process for manufacturing a Pfs48/45 based Plasmodium falciparum transmission-blocking vaccine. Front Immunol. 2021;11:606266. doi: 10.3389/fimmu.2020.606266
- Tachibana M, Suwanabun N, Kaneko O, et al. Plasmodium vivax gametocyte proteins, Pvs48/45 and Pvs47, induce transmission-reducing antibodies by DNA immunization. Vaccine. 2015;33(16):1901–1908. doi: 10.1016/j.vaccine.2015.03.008
- Tachibana M, Miura K, Takashima E, et al. Identification of domains within Pfs230 that elicit transmission blocking antibody responses. Vaccine. 2019 Mar 22;37(13):1799–1806. doi: 10.1016/j.vaccine.2019.02.021
- Inklaar MR, de Jong RM, Bekkering ET, et al. Pfs230 Domain 7 is targeted by a potent malaria transmission-blocking monoclonal antibody. NPJ Vaccines. 2023 Dec 12;8(1):186. doi: 10.1038/s41541-023-00784-x
- Lee S-M, Wu Y, Hickey JM, et al. The Pfs230 N-terminal fragment, Pfs230D1+: expression and characterization of a potential malaria transmission-blocking vaccine candidate. Malaria j. 2019;18(1):1–13. doi: 10.1186/s12936-019-2989-2
- Carter R, Miller L, Rener J, et al. Target antigens in malaria transmission blockling immunity. Philos Trans R Soc London B. 1984;307(1131):201–213.
- Kumar N, Carter R. Biosynthesis of two stage-specific membrane proteins during transformation of Plasmodium gallinaceum zygotes into ookinetes. Mol Biochem Parasitol. 1985;14(2):127–139. doi: 10.1016/0166-6851(85)90032-5
- Singh SK, Roeffen W, Andersen G, et al. A Plasmodium falciparum 48/45 single epitope R0.6C subunit protein elicits high levels of transmission blocking antibodies. Vaccine. 2015 Apr 15;33(16):1981–1986. doi: 10.1016/j.vaccine.2015.02.040
- Kumar R, Ledet G, Graves R, et al. Potent functional immunogenicity of Plasmodium falciparum transmission-blocking antigen (Pfs25) delivered with nanoemulsion and porous polymeric nanoparticles [journal article]. Pharm Res. 2015;32(12):3827–3836. doi: 10.1007/s11095-015-1743-x
- Datta D, Bansal GP, Kumar R, et al. Evaluation of the impact of codon optimization and N-Linked glycosylation on functional immunogenicity of Pfs25 DNA vaccines delivered by in vivo electroporation in preclinical studies in mice. Clin Vaccin Immunol. 2015 Sep 1;22(9):1013–1019. doi: 10.1128/CVI.00185-15
- Datta D, Bansal GP, Gerloff DL, et al. Immunogenicity and malaria transmission reducing potency of Pfs48/45 and Pfs25 encoded by DNA vaccines administered by intramuscular electroporation. Vaccine. 2017 Jan 5;35(2):264–272. doi: 10.1016/j.vaccine.2016.11.072
- Cao Y, Hayashi CT, Zavala F, et al. Effective functional immunogenicity of a DNA vaccine combination delivered via in vivo electroporation targeting malaria infection and transmission. Vaccines. 2022;10(7):1134. doi: 10.3390/vaccines10071134
- Hayashi CTH, Cao Y, Clark LC, et al. mRNA-LNP expressing PfCSP and Pfs25 vaccine candidates targeting infection and transmission of Plasmodium falciparum. NPJ Vaccines. 2022 Dec 01;7(1):155. doi: 10.1038/s41541-022-00577-8
- Kunkeaw N, Nguitragool W, Takashima E, et al. A Pvs25 mRNA vaccine induces complete and durable transmission-blocking immunity to Plasmodium vivax. NPJ Vaccines. 2023 Dec 14;8(1):187. doi: 10.1038/s41541-023-00786-9
- Chichester JA, Green BJ, Jones RM, et al. Safety and immunogenicity of a plant-produced Pfs25 virus-like particle as a transmission blocking vaccine against malaria: a phase 1 dose-escalation study in healthy adults. Vaccine. 2018;36(39):5865–5871. doi: 10.1016/j.vaccine.2018.08.033
- Talaat KR, Ellis RD, Hurd J, et al. Safety and immunogenicity of Pfs25-EPA/Alhydrogel®, a transmission blocking vaccine against Plasmodium falciparum: an open label study in malaria naïve adults. PLoS One. 2016;11(10):e0163144. doi: 10.1371/journal.pone.0163144
- Sagara I, Healy SA, Assadou MH, et al. Malaria transmission-blocking vaccines Pfs230D1-EPA and Pfs25-EPA in Alhydrogel in healthy Malian adults; a phase 1, randomised, controlled trial. Lancet Infect Dis. 2023;23(11):1266–1279. doi: 10.1016/S1473-3099(23)00276-1
- Healy SA, Anderson C, Swihart BJ, et al. Pfs230 yields higher malaria transmission–blocking vaccine activity than Pfs25 in humans but not mice. J Clin Invest. 2021 Apr 01;131(7). doi: 10.1172/JCI146221
- De Graaf H, Payne RO, Taylor I, et al. Safety and immunogenicity of ChAd63/MVA Pfs25-IMX313 in a Phase I first-in-human trial. Front Immunol. 2021;12:2823. doi: 10.3389/fimmu.2021.694759
- Duez J, Holleran JP, Ndour PA, et al. Splenic retention of Plasmodium falciparum gametocytes to block the transmission of malaria. Antimicrob Agents Chemother. 2015 Jul 1;59(7):4206–4214. doi: 10.1128/AAC.05030-14
- Joice R, Nilsson SK, Montgomery J, et al. Plasmodium falciparum transmission stages accumulate in the human bone marrow. Sci, trans med. 2014;6(244):re2445–re2445. doi: 10.1126/scitranslmed.3008882
- Aguilar R, Magallon-Tejada A, Achtman AH, et al. Molecular evidence for the localization of Plasmodium falciparum immature gametocytes in bone marrow. Blood. 2014;123(7):959–966. doi: 10.1182/blood-2013-08-520767
- Stone WJ, Dantzler KW, Nilsson SK, et al. Naturally acquired immunity to sexual stage P. falciparum parasites. Parasitology. 2016;143(2):187–198. doi: 10.1017/S0031182015001341
- Dantzler KW, Ravel DB, Brancucci NMB, et al. Ensuring transmission through dynamic host environments: host–pathogen interactions in Plasmodium sexual development. Curr Opin Microbiol. 2015 8;26:17–23. doi: 10.1016/j.mib.2015.03.005
- Rogers NJ, Targett G, Hall BS. Plasmodium falciparum gametocyte adhesion to C32 cells via CD36 is inhibited by antibodies to modified band 3. Infect Immun. 1996;64(10):4261–4268. doi: 10.1128/iai.64.10.4261-4268.1996
- Rogers NJ, Daramola O, Targett G, et al. CD36 and intercellular adhesion molecule 1 mediate adhesion of developing Plasmodium falciparum gametocytes. Infect Immun. 1996;64(4):1480–1483. doi: 10.1128/iai.64.4.1480-1483.1996
- Bansal GP, Kumar N. Immune responses in malaria transmission. Curr Clin Microbiol Rep. 2018;5(1):1–7. doi: 10.1007/s40588-018-0078-x
- Saeed M, Fivelman Q, Drakeley CJ, et al. Introducing GSA: surface antigens of P-falciparum gametocyte-infected erythrocytes. Int J Parasitol. 2008;38:S81–S81.
- Tonwong N, Sattabongkot J, Tsuboi T, et al. Natural infection of Plasmodium falciparum induces inhibitory antibodies against gametocyte development in human hosts. Jpn J Infect Dis. 2012;65(2):152–156. doi: 10.7883/yoken.65.152
- Silvestrini F, Lasonder E, Olivieri A, et al. Protein export marks the early phase of gametocytogenesis of the human malaria parasite Plasmodium falciparum*. Mol & Cell Proteomics. 2010 Jul 01;9(7):1437–1448. doi: 10.1074/mcp.M900479-MCP200
- Delrieu I, Waller CC, Mota MM, et al. PSLAP, a protein with multiple adhesive motifs, is expressed in Plasmodium falciparum gametocytes. Mol Biochem Parasitol. 2002;121(1):11–20. doi: 10.1016/S0166-6851(02)00016-6
- Dantzler KW, Ma S, Ngotho P, et al. Naturally acquired immunity against immature Plasmodium falciparum gametocytes. Sci Transl Med. 2019;11(495):eaav3963. doi: 10.1126/scitranslmed.aav3963
- Kengne-Ouafo JA, Bah SY, Kemp A, et al. The global transcriptome of Plasmodium falciparum mid-stage gametocytes (stages II–IV) appears largely conserved and gametocyte-specific gene expression patterns vary in clinical isolates. Microbiol Spectr. 2023;11(5):e03820–22. doi: 10.1128/spectrum.03820-22
- Ouédraogo AL, Bousema T. Transmission-blocking immunity in malaria. In: Kremsner P, Krishna S, editors. Encyclopedia of malaria. New York, NY: Springer; 2018. p. 1–7.
- Eichner M, Diebner H, Molineaux L, et al. Genesis, sequestration and survival of Plasmodium falciparum gametocytes: parameter estimates from fitting a model to malariatherapy data. Trans R Soc Trop Med Hyg. 2001;95(5):497–501. doi: 10.1016/S0035-9203(01)90016-1
- Tadesse FG, Slater HC, Chali W, et al. The relative contribution of symptomatic and asymptomatic Plasmodium vivax and Plasmodium falciparum infections to the infectious reservoir in a low-endemic setting in Ethiopia. Clinical Infectious Diseases. 2018;66(12):1883–1891. doi: 10.1093/cid/cix1123
- Felger I, Maire M, Bretscher MT, et al. The dynamics of natural Plasmodium falciparum infections. PLoS One. 2012;7(9):e45542. doi: 10.1371/journal.pone.0045542
- Bousema T, Okell L, Felger I, et al. Asymptomatic malaria infections: detectability, transmissibility and public health relevance. Nature Rev Microbiol. 2014;12(12):833–840. doi: 10.1038/nrmicro3364
- Naotunne TDS, Karunaweera ND, Del Giudice G, et al. Cytokines kill malaria parasites during infection crisis: extracellular complementary factors are essential. J Exp Med. 1991;173(3):523–529. doi: 10.1084/jem.173.3.523
- Naotunne TS, Karunaweera ND, Mendis K, et al. Cytokine-mediated inactivation of malarial gametocytes is dependent on the presence of white blood cells and involves reactive nitrogen intermediates. Immunology. 1993;78(4):555.
- Mendis K, Naotunne T, Karunaweera N, et al. Anti-parasite effects of cytokines in malaria. Immunol Lett. 1990;25(1–3):217–220. doi: 10.1016/0165-2478(90)90118-A
- Karunaweera N, Grau G, Gamage P, et al. Dynamics of fever and serum levels of tumor necrosis factor are closely associated during clinical paroxysms in Plasmodium vivax malaria. Proc Natl Acad Sci, USA. 1992;89(8):3200–3203. doi: 10.1073/pnas.89.8.3200
- Targett G, Harte P, Eida S, et al. Plasmodium falciparum sexual stage antigens: immunogenicity and cell-mediated responses. Immunol Lett. 1990;25(1–3):77–81. doi: 10.1016/0165-2478(90)90095-8
- Harte P, Rogers N, Targett G. Role of T cells in preventing transmission of rodent malaria. Immunology. 1985;56(1):1–7. doi: 10.1111/j.1365-3024.1985.tb00104.x
- Good MF, Quakyi IA, Saul A, et al. Human T clones reactive to the sexual stages of Plasmodium falciparum malaria. High frequency of gamete-reactive T cells in peripheral blood from nonexposed donors. J Immunol. 1987 Jan 1;138(1):306–311. doi: 10.4049/jimmunol.138.1.306
- Peiris JS, Premawansa S, Ranawaka MB, et al. Monoclonal and polyclonal antibodies both block and enhance transmission of human Plasmodium vivax malaria. Am J Trop Med Hyg. 1988 Jul;39(1):26–32. doi: 10.4269/ajtmh.1988.39.26
- Bansal GP, Araujo M, Cao Y, et al. Transmission-reducing and -enhancing monoclonal antibodies against Plasmodium vivax gamete surface protein Pvs48/45. Infect Immun. 2024;92(3):e00374–23. doi: 10.1128/iai.00374-23
- Paul NH, Vengesai A, Mduluza T, et al. Prevalence of Plasmodium falciparum transmission reducing immunity among primary school children in a malaria moderate transmission region in Zimbabwe. Acta tropica. 2016;163:103–108. doi: 10.1016/j.actatropica.2016.07.023
- Stone W, Bousema T, Sauerwein R, et al. Two-faced immunity? The evidence for antibody enhancement of malaria transmission. Trends Parasitol. 2019;35(2):140–153. doi: 10.1016/j.pt.2018.11.003
- de Arruda-Mayr M, Cochrane AH, Nussenzweig RS. Enhancement of a simian malarial infection (Plasmodium cynomolgi) in mosquitoes fed on rhesus (Macaca mulatta) previously infected with an unrelated malaria (Plasmodium knowlesi). Am J Trop Med Hyg. 1979;28(4):627–633. doi: 10.4269/ajtmh.1979.28.627
- Jerusalem C, Weiss ML, Poels L. Immunologic enhancement in malaria infection (plasmodium berghei). J Immunol. 1971;107(1):260–268. doi: 10.4049/jimmunol.107.1.260
- Yang X, Zhang X, Zhao X, et al. Antibody-dependent enhancement: ″Evil″ antibodies favorable for viral infections. Viruses. 2022;14(8):1739. doi: 10.3390/v14081739