Abstract
Objective: Observational cohort study which aimed to explore the potential of electrohysterogram (EHG) analysis for detecting a uterine rupture during trial of labor after cesarean. The EHG propagation characteristics surrounding the uterine scar of six patients with a previous cesarean section were compared to a control group of five patients without a scarred uterus.
Methods: The EHG was recorded during the first stage of labor using a high-resolution 64-channel electrode grid positioned on the maternal abdomen across the cesarean scar. Based on simulations, the inter-channel correlation and propagation direction were adopted as EHG parameters for evaluating possible disruption of electrical propagation by the uterine scar.
Results: No significant differences in inter-channel correlation or propagation direction were observed between the group of patients with an intact uterine scar and the control group. A strong predominance of vertical propagation was observed in one case, in which scar rupture occurred.
Conclusions: The results support unaffected propagation of electrical activity through the intact uterine scar tissue suggesting that changes in the EHG might only occur in case of rupture.
Introduction
Uterine rupture is a rare but serious complication which is closely related to a previous cesarean section (CS) [Citation1–3]. Establishing a uterine rupture is a very challenging diagnosis since the symptoms can be late signs or even completely absent [Citation4–7]. Currently, intrapartum monitoring during trial of labor is limited to assessing the fetal condition and uterine activity by cardiotocography, which is not reliable for detecting a uterine rupture [Citation2,Citation3,Citation6]. Reducing the risks involved with attempting trial of labor after CS requires improved monitoring methods during trial of labor. A potential alternative modality is the electrohysterogram (EHG), which entails noninvasive abdominal measurement of the depolarizations of uterine smooth muscle cells. Synchronized uterine contraction arises from cell to cell propagation of action potentials through the myometrium [Citation8]. The presence of scar tissue or rupture of this scar could interrupt propagation of action potentials or locally change the propagation direction by disruption of the vertical propagation pathway.
Little is known about the physiological propagation patterns in the unscarred lower uterine segment and the conductive properties of scar tissue in the myometrium. In cardiac muscle, scar tissue created by surgical incisions is used as a method for interrupting propagation, in order to prevent macro reentry circuits [Citation9]. Smooth muscle, on the other hand, is considered to have considerable regenerative capacity and histological studies of scar tissue after CS have found the normal tissue architecture to be mostly restored [Citation10]. The EHG has been demonstrated to have great potential for characterizing electrical propagation [Citation11]. In previous work, we analyzed propagation patterns by means of a grid of 64 closely spaced electrodes [Citation12–14]. The square arrangement of the electrodes in this grid allows estimation in all possible directions [Citation15]. In addition, a small inter-electrode distance permits tracking the course of electrical propagation through the electrode grid.
This study aimed at exploring the potential of EHG propagation analysis for detecting a uterine rupture. The primary goals were to determine the feasibility of EHG recording over the lower uterine segment and to study the baseline propagation characteristics of an intact uterine scar during trial of labor after cesarean in comparison with a control group.
Methods
Study protocol
A prospective observational cohort study was performed at the Máxima Medical Center, Veldhoven, the Netherlands. Approval from the Local Medical Ethical Board was issued on 8 May 2013 under registration number NL43294.015.13. All the included women provided written informed consent for study participation. Six patients with a single previous CS and five patients without uterine scar as control were enrolled. Inclusion criteria were:
first stage of labor: fully effaced cervix, dilatation ≥ 1 cm, ≥ 3 contractions/10 min
singleton pregnancy
epidural analgesia
foley bladder catheter
As an additional inclusion criterion for the group with a previous CS, the gestational age of the previous CS had to be within two weeks of the current delivery in order to avoid differences in position of the uterine scar. Operation techniques other than a horizontal incision in the lower uterine segment were excluded. Furthermore, patients with previous multiple gestations were excluded.
Measurements from all enrolled patients were obtained using a high-density electrode grid of 62 × 62 mm, containing 64 monopolar electrodes. The scar on the skin was the reference to determine the location of the uterine scar underneath. The grid was positioned in the midline of the abdomen and centered over the scar (see ). A reference electrode was positioned on the left hip. Additionally an 3D accelerometer was used to record maternal movement plus an external tocodynamometer for contraction detection. All signals were recorded using a Refa multichannel amplifier (TMS International, Enschede, the Netherlands).
Figure 1. Positioning of the sensors on the maternal abdomen. The 64-channel electrode grid was positioned in the midline of the abdomen and centered over the scar. An external reference electrode was positioned on the left hip. In addition, an accelerometer was attached to the electrode grid and an external tocodynamometer was used.
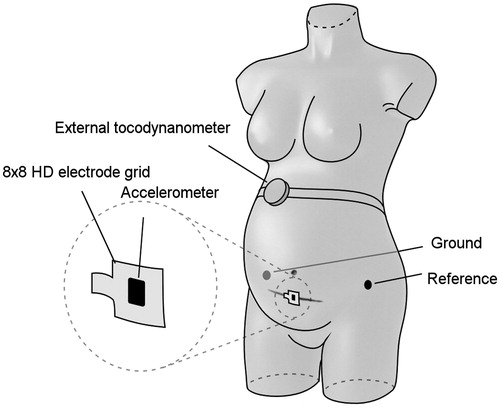
Signal analysis
As a first step, simulated EHG signals were constructed that emulated different scenarios of altered electrical propagation caused by the presence of non-conducting uterine scar tissue. A wave front propagating through a 64-channel electrode grid was simulated, either interrupted midway by the scar tissue or circulating around the uterine scar. In addition, a simulation of a horizontally traveling wave front was included which was not impeded by the virtual scar tissue. These simulated signals served to test multiple EHG parameters in their ability to detect altered propagation caused by a conduction block. Both in simulations of an interrupted or a circulating wave front, the signals of the channels on each side of the simulated scar tissue were less correlated in the time domain. The correlation between vertical pairs of adjacent electrodes was found to be most sensitive for detecting interruption of propagation. However, only estimation of the conduction velocity (CV) vector could correctly identify a horizontal propagation direction. Based on these simulations, two EHG parameters were adopted for signal analysis: inter-channel linear correlation in the time domain and CV analysis based on single spike propagation [Citation12]. We hypothesized that an conduction block caused by the presence of scar tissue would result in the inter-channel correlation to be lower for the middle area of the electrode grid and the propagation direction to be predominantly horizontal rather than variable.
Analysis of propagation direction requires the phase of the propagating EHG signal to be unaffected, necessitating a monopolar electrode configuration. To this end, all 64 monopolar signals were referred to an external reference channel not containing EHG signal. In order to minimize the influence of maternal respiration and ECG, all EHG signals were band-pass filtered between 0.35 and 0.8 Hz and downsampled to 20 Hz [Citation16]. shows an overview of the sensors used, the selection of contraction segments and the multi-channel analysis of the EHG. Segments containing artifacts were identified based on the accelerometer and removed from the analysis. Contractions were manually selected based on either the external tocodynamometer, when available, or the estimated intrauterine pressure (eIUP). The method for deriving the eIUP was a root mean square based method similar to the one described by Jezewski et al. [Citation17,Citation18], only using the frequency band 0.35–0.80 Hz. A minimum of three and a maximum of four contractions were selected for each patient.
Figure 2. Schematic representation of the methods for signal analysis. The EHG was recorded using a 64-channel electrode grid (A. Sensors). The external tocodynamometer was used for identifying contractions, while the accelerometer recorded maternal movement (B. Contraction selection). Within the selected contraction segments, the mean correlation between vertical and horizontal adjacent electrode pairs was calculated for three areas of the grid, shown as the yellow, orange, and red zones. The conduction velocity was calculated in squares of four adjacent electrodes, resulting in 49 vectors (C. Multi-channel EHG analysis). Subsequently, the amplitude and angles of these vectors were averaged and the propagation was categorized as horizontal or vertical.
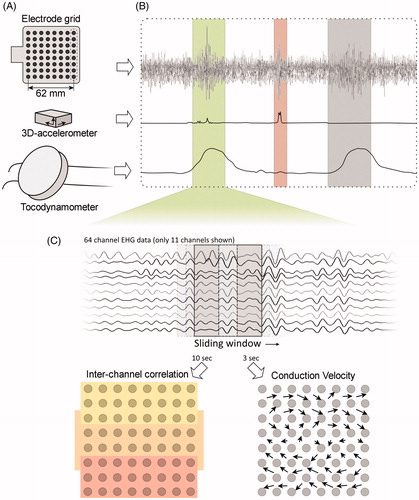
The inter-channel correlation was calculated in a sliding window of 10 s by means of the Pearson product-moment correlation coefficient between horizontal or vertical pairs of two adjacent electrodes, thereby obtaining two sets of 56 values per window. Channels containing large artifacts or with a low signal-to-noise ratio were discarded. In case of one neighboring missing channel, the correlation with the next electrode was calculated. In case of two or more missing channels, the channels were discarded. The electrode grid was divided in three areas, corresponding to the electrodes above, over, and below the uterine scar. The mean inter-channel correlation was calculated for all three areas of the grid, both for the horizontal and vertical pairs of electrodes.
The CV vector was estimated in each square of four adjacent electrodes, resulting in 49 values sampled every 50 ms. The time difference between every channel pair was determined by maximizing the normalized cross-correlation between two offset signals in a sliding window of 3 s. A shorter time window was used to account for a highly variable direction of propagation. The time differences were limited to a range corresponding to a velocity between 3 cm/s and 30 cm/s for propagation within an angle of 120° around the direction of each electrode pair. Along each pair, a vector of observed velocity was calculated from their time difference and electrode positions. A plane wave propagation front was fitted through these individual vectors, resulting in a CV vector. The root mean square error of the fit was used to discard observations with an error exceeding the amplitude of the CV. Fits based on less than three vectors were discarded as well. The CV amplitude and angle were averaged independently over all 49 observations for each time step, preserving the CV amplitude in a noisy signal. Finally, the resulting CV angle was categorized as horizontal or vertical depending whether it was within 45 degrees of the horizontal or vertical axis.
Statistical analysis
Mean values of inter-channel correlation for the three areas of electrodes were derived. Furthermore, the mean CV amplitude and the distribution of horizontal and vertical propagation was calculated for each patient. Levene’s test was applied to test for equal variances in the scar and control group. An one-way ANOVA was used to test for significant differences in the results on inter-channel correlation between groups of electrodes, within the scar and control group. Differences between CV amplitude and propagation direction were also tested for significance, comparing the results from the scar and control group using one-way ANOVA. A sample size of 10 patients resulted in a power (1 − β) of 0.80 to detect an effect size of 1.72, allowing for a type I error rate (α) of 0.05. This effect size was based on the inter-channel correlation of simulated EHG signals circulating around the uterine scar. A worst-case estimate of the standard deviation was assumed, considering the correlation of channels containing white noise only. The alpha was set to 0.05 for all statistical tests.
Results
In total, 11 patients in the first stage of labor were included in the study: six patients with a previous CS and five patients without scarred uterus as control. Supplemental digital content s1 shows a brief outline of the patient characteristics. Rupture of the uterine scar occurred in one patient that was part of five pilot measurements which were recorded just prior to inclusion of the main body of patients. Uterine rupture entailed complete separation of the uterine wall with clinical symptoms [Citation3]. This patient had a history of a CS and labor was induced at term by amniotomy and administration of oxytocin. Two hours prior to the measurement, the medical record states complaints of increased abdominal pain despite previously adequate pain relief by epidural analgesia. Approximately an hour following the measurement, decelerations were noted in the fetal heart rate tracing. The decision was made for a secondary CS for arrest of labor at 7 cm despite an adequate and regular contraction rate of 3–4 contractions per 10 min. Approximately 3 h after the measurement, a uterine rupture was found during the CS: the peritoneal cavity was filled with hemorrhagic amniotic fluid and a 2–3 cm defect was visible at the left side of the location of the uterine scar.
Two examples of recorded EHG signals, in which bursts of activity are visible that correspond to contractions in the tocogram, are shown in supplemental digital content s2. In total, 40 contractions were analyzed. Levene’s test did not show significance in any of the outcomes, thereby supporting equal variances in the intact scar and control group. Based on the observed standard deviation, the post hoc analysis showed sufficient achieved power. In both groups, the inter-channel correlation in the middle area was similar to the upper- and lower areas of electrodes (, upper panel). All observed differences were non-significant.
Figure 3. Upper panel: results of inter-channel correlation in the time domain using horizontal and vertical pairs of electrodes. Results are averaged in three areas of the grid, corresponding to the electrodes above, over and below the uterine scar respectively. Observed differences between the different areas, were non-significant within both groups. Lower panel: Results on conduction velocity amplitude and angle. The mean amplitude of the CV vector per group is shown in the left panel. The estimated CV vector angles were categorized as horizontal and vertical and the mean distribution is shown on the right. Results were compared between the scar and control group and the observed differences were non-significant.
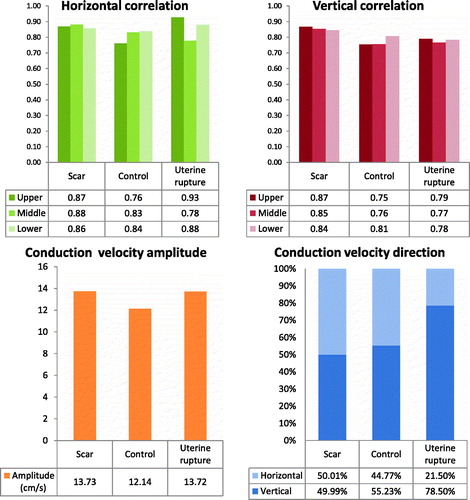
The average amplitude of the CV vector was similar for the intact scar and control group as well as in the case of uterine rupture (, lower panel). The small difference between the intact scar and control group was non-significant. The angle of the CV vector was evenly distributed in patients of both groups between horizontal and vertical direction. In the EHG signal of the patient in which uterine rupture occurred, an abnormal propagation pattern was observed showing a strong predominance of vertical propagation.
Discussion
This study aimed to explore the potential of EHG propagation analysis for detecting a uterine rupture during trial of labor. The baseline propagation characteristics of an intact uterine scar were studied and compared to a control group to understand the effect of scar tissue on electrical propagation. The EHG was recorded using a high-resolution electrode grid positioned over the lower uterine segment across the scar. Based on simulations, inter-channel correlation and the CV vector were adopted as EHG parameters for evaluating possible disruption of electrical propagation. The measurements show that EHG signals can be recorded in the lower uterine segment. No significant differences in inter-channel correlation or propagation direction were observed between the group of patients with an intact uterine scar and the control group. Therefore, the results support unaffected propagation of electrical activity by the intact uterine scar tissue. In contrast, a strong predominance of vertical propagation was observed in the uterine rupture case.
In line with EHG signals recorded at other locations of the uterus during term labor, the signals obtained from the lower uterine segments showed typical bursts of activity alternated by quiescent periods. These bursts clearly corresponded to the contractions visible in the tocogram and showed a good inter-channel correlation. The signal within these bursts was found to propagate through the electrode grid at a velocity that was in the range of values found in electrophysiological [Citation19–22] and previous clinical studies [Citation14,Citation16]. However, recording the EHG from the lower uterine segment is challenging especially considering the gradual thinning of the lower uterine segment during the first stage of labor. This requires extra care for obtaining an optimal electrode-skin contact to ensure optimal signal quality.
The results on inter-channel correlation showed comparable values for the three areas of the grid in all cases. Based on simulations, in case of interruption of electrical activity by the scar, the results on correlation were expected to be lower for the middle area of electrodes representing the electrical activity of the uterine scar tissue. However, this effect on correlation by the scar tissue might be local and therefore sensitive to placement of the electrode grid. In order to achieve an optimal approximation of the location of the uterine scar underneath, the scar on the skin was used as reference for placement of the electrode grid over the uterine scar. Moreover, to avoid differences in scar height, all patients in the scar group had a previous transverse incision in the lower uterine segment at a gestational age within two weeks of the gestational age at the time of recording. Finally, to account for possible remaining inaccuracy in positioning the electrode grid, the middle area of the grid spanned multiple rows of electrodes. A local effect by the scar tissue in inter-channel correlation may have been reduced by using multiple rows. However, also in the individual results of electrode pairs on correlation, no trend indicating lower correlation in the middle was evident.
Analysis of the local propagation direction might be less sensitive for misplacement of the electrode grid over the uterine scar and therefore more suitable for a clinical application of detecting failure of a uterine scar during labor. The propagation direction proved to be variable in both the scar and control group and was evenly distributed between a horizontal and vertical direction of propagation. This is in line with previous analysis of propagation direction [Citation16,Citation19,Citation23] and consistent with previous studies on the electrophysiology of the uterus in which no fixed pacemaker location has been identified [Citation13,22,24–26]. This finding, in combination with the results on the inter-channel correlation, does not show any disruption of propagation by an intact uterine scar implicating that EHG changes might only occur in case of rupture. However, in a follow-up study the sample size should be adequate in order to discern possible inter-patient variation in wound healing [Citation27].
In order to explore the propagation pattern preceding failure of a uterine scar, one case of uterine rupture was included. The diagnosis was confirmed over 3 h after the measurement during CS; therefore, the exact status of the uterine scar during the measurement could not be fully ascertained. However, the observed clinical signs (arrest of labor, increased abdominal pain despite epidural analgesia, and decelerations in the fetal heart rate tracing) suggest that the scar might already have ruptured at the time of the measurement. In this recording, a strong predominance of vertical propagation was found. Horizontal propagation is to be expected if the rupture would be located underneath the electrodes. Moreover, no lower values for inter-channel correlation were found in the middle rows using vertical pairs. It should be noted that only 16 channels out of 64 were recorded for this case, resulting in an interelectrode distance of 17.7 mm. The lower spatial resolution may have reduced the sensitivity for detecting interruption of propagation. During the surgery, it was established that the uterine scar had ruptured on the left side and therefore was possibly located entirely next to the electrode grid. This could have resulted in local conduction to be predominantly vertical in this case by propagation fronts circumventing or circulating around the defect in the uterine scar. In general, rupture can occur in any part of the uterine scar and can result in an oval shaped defect. Therefore, any dominant direction of propagation might indicate a uterine rupture. Since the intact uterine scar tissue does not appear to influence the propagation of electrical activity, and an abnormal propagation pattern was found in case of uterine rupture, EHG changes might only occur in case of rupture of the uterine scar.
In order to reliably detect a change in propagation direction caused by failure of the scar during labor, the size of the electrode grid should be adapted to cover a larger part of the uterine scar. This study was limited to the use of linear propagation models of cell to cell propagation. In addition, non-linear models can be considered as these might provide more accurate results [Citation28–30].
Conclusion
The observed propagation patterns in the lower uterine segment support unaffected propagation of electrical activity through the intact uterine scar suggesting that changes in the EHG might only occur in case of failure of the scar. This study motivates further research in a larger group of patients since advanced analysis of the EHG propagation pattern could potentially provide detection of scar rupture during trial of labor after cesarean.
Declaration of interest
The authors report no declarations of interest.
Supplementary material available online
Acknowledgements
We wish to acknowledge the staff at the Maxima Medical Center in the Netherlands and specially Joris Renckens, Olenka Hulsenboom, and Noortje Eijsvoogel for assistance in patient selection and data collection.
References
- Lydon-Rochelle M, Holt VL, Easterling TR, Martin DP. Risk of uterine rupture during labor among women with a prior cesarean delivery. N Engl J Med 2001;345:3–8
- Zwart JJ, Richters JM, Ory F, et al. Uterine rupture in The Netherlands: a nationwide population-based cohort study. BJOG 2009;116:1069–78. Discussion 1078-80
- Kwee A, Bots ML, Visser GH, Bruinse HW. Uterine rupture and its complications in the Netherlands: a prospective study. Eur J Obstet Gynecol Reprod Biol 2006;128:257–61
- Ayres AW, Johnson TR, Hayashi R. Characteristics of fetal heart rate tracings prior to uterine rupture. Int J Gynaecol Obstet 2001;74:235–40
- Kwee A, Bots ML, Visser GH, Bruinse HW. Obstetric management and outcome of pregnancy in women with a history of caesarean section in the Netherlands. Eur J Obstet Gynecol Reprod Biol 2007;132:171–6
- Ridgeway JJ, Weyrich DL, Benedetti TJ. Fetal heart rate changes associated with uterine rupture. Obstet Gynecol 2004;103:506–12
- Tutschek B, Bender HG, Henrich W. Silent uterine rupture during vaginal delivery successfully managed conservatively. Ultrasound Obstet Gynecol 2005;26:199–200
- Lammers WJ. The electrical activities of the uterus during pregnancy. Reprod Sci 2013;20:182–9
- Cox JL, Schuessler RB, D’Agostino HJ, Jr. et al. The surgical treatment of atrial fibrillation. III. Development of a definitive surgical procedure. J Thorac Cardiovasc Surg 1991;101:569–83
- Schwarz OH, Paddock R. The cesarean scar. Am J Obstet Gynecol 1925;10:153–71
- Rabotti C, Mischi M. Propagation of electrical activity in uterine muscle during pregnancy: a review. Acta Physiol (Oxf) 2015;213:406–16
- de Lau H, Rabotti C, Bijloo R, et al. Automated conduction velocity analysis in the electrohysterogram for prediction of imminent delivery: a preliminary study. Comput Math Methods Med 2013;2013:627976
- Rabotti C, Mischi M, Oei SG, Bergmans JW. Noninvasive estimation of the electrohysterographic action-potential conduction velocity. IEEE Trans Biomed Eng 2010;57:2178–87
- van ‘t Hooft J, Rabotti C, Oei SG. Electrohysterographic evaluation of preterm contractions in a patient with a unicornuate uterus. Acta Obstet Gynecol Scand 2013;92:730–3
- Rabotti C, Oei SG, van ‘t Hooft J, Mischi M. Electrohysterographic propagation velocity for preterm delivery prediction. Am J Obstet Gynecol 2011;205:e9–10; author reply e10
- de Lau H, Rabotti C, Bijlo R, et al. Automated conduction velocity analysis in the electrohysterogram for prediction of imminent delivery: a preliminary study. Comput Mathemat Methods Med 2013;2013:7
- Jezewski J, Horoba K, Matonia A, Wrobel J. Quantitative analysis of contraction patterns in electrical activity signal of pregnant uterus as an alternative to mechanical approach. Physiol Meas 2005;26:753–67
- Rooijakkers MJ, Rabotti C, Oei SG, et al. Low-complexity intrauterine pressure estimation using the Teager energy operator on electrohysterographic recordings. Physiol Meas 2014;35:1215–28
- Lammers WJ, Arafat K, el-Kays A, el-Sharkawy TY. Spatial and temporal variations in local spike propagation in the myometrium of the 17-day pregnant rat. Am J Physiol 1994;267:C1210–23
- Lammers WJ, Mirghani H, Stephen B, et al. Patterns of electrical propagation in the intact pregnant guinea pig uterus. Am J Physiol Regul Integr Comp Physiol 2008;294:R919–28
- Miller SM, Garfield RE, Daniel EE. Improved propagation in myometrium associated with gap junctions during parturition. Am J Physiol 1989;256:C130–41
- Parkington HC, Harding R, Sigger JN. Co-ordination of electrical activity in the myometrium of pregnant ewes. J Reprod Fertil 1988;82:697–705
- Mikkelsen E, Johansen P, Fuglsang-Frederiksen A, Uldbjerg N. Electrohysterography of labor contractions: propagation velocity and direction. Acta Obstet Gynecol Scand 2013;92:1070–8
- Wolfs G, van Leeuwen M, Rottinghuis H, Boeles JT. An electromyographic study of the human uterus during labor. Obstet Gynecol 1971;37:241–6
- Kao CY. Long-term observations of spontaneous electrical activity of the uterine smooth muscle. Am J Physiol 1959;196:343–50
- Wolfs G, Rottinghuis H. Electrical and mechanical activity of the human uterus during labour. Arch Gynakol 1970;208:373–85
- Buhimschi CS, Zhao G, Sora N, et al. Myometrial wound healing post-Cesarean delivery in the MRL/MpJ mouse model of uterine scarring. Am J Pathol 2010;177:197–207
- Diab A, Hassan M, Marque C, Karlsson B. Performance analysis of four nonlinearity analysis methods using a model with variable complexity and application to uterine EMG signals. Med Eng Phys 2014;36:761–7
- Diab A, Hassan M, Boudaoud S, et al. Nonlinear estimation of coupling and directionality between signals: application to uterine EMG propagation. Conf Proc IEEE Eng Med Biol Soc 2013;2013:4366–9
- Hassan M, Terrien J, Muszynski C, et al. Better pregnancy monitoring using nonlinear correlation analysis of external uterine electromyography. IEEE Trans Biomed Eng 2013;60:1160–6