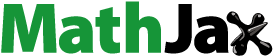
ABSTRACT
Tungsten carbide-based nano-catalysts (Ni-WC, Co-WC and NiCo-WC) and molybdenum carbide-based nano-catalysts (Ni-MoC, Co-MoC and NiCo-MoC) were synthesised using a carbothermal reduction method. X-ray diffraction, scanning electron microscopy coupled with electron dispersive spectroscopy, x-ray photoelectron spectroscopy and cyclic voltammetry were used to evaluate the prepared electrocatalysts. The cyclic voltammetry results showed that Ni-WC coated electrode displayed the best hydrogen evolution reaction catalytic activity with the lowest overpotential of 1.421 V at a current density of 10 mA cm−2, and a Tafel slope of 135 mV dec−1 which is marginally lower than the benchmark Pt/C coated electrodes. Ni-MoC and Co-MoC coated electrocatalysts also produced current more effectively with a change in potential as compared to the Pt/C coated electrode. The durability test showed Co-WC, NiCo-WC and Ni-MoC coated electrodes to have high stability within a wide range of current densities.
Introduction
Clean and sustainable energy alternatives are being explored owing to the limited reserve of fossil fuels. Production of hydrogen (H2) from fossil fuels cannot keep up with the need required by increased social and economic development (Miao et al. Citation2017). Using water splitting to produce H2 can help conserve the limited natural resources (Zhu et al. Citation2020; Li and Fei Citation2022) H2 is environmentally friendly and has a high energy density (Sapountzi et al. Citation2017). Platinum-based catalysts are by far the best electrocatalysts for hydrogen evolution reaction (HER) but their application is restricted by its high cost (Gao et al. Citation2019). The development of efficient electrocatalysts with reduced dynamic overpotential is of great importance in HER (Gao et al. Citation2019). Non-platinum metal based electrocatalysts such as molybdenum (Mo) carbide (Miao et al. Citation2017), and tungsten (W) carbide are used to replace the noble metals (Li and Fei Citation2022; Gong et al. Citation2016). They have attracted interest in electrocatalytic hydrogen production research due to their low cost and availability (Gao et al. Citation2019). Also, their d-band electronic density is identical to that of platinum (Gong et al. Citation2016), which is useful for activating hydrogen (Xiong et al. Citation2015). The contraction of the metal d-band (which mimics that of Pt) of the transition metals, is caused by the incorporation of the carbon which in turn leads to the expansion of a lattice parameter (Gómez-Marín and Ticianelli Citation2017). However, the slow kinetics of desorption of the hydrogen form the carbides limits its usefulness. This restriction can be alleviated by doping the TMC with group VIII transition metal (Michalsky et al. Citation2014; Greeley et al. Citation2006). The effectiveness of the doping of the TMC with group VIII metals has been established by the increase in catalytic activity for a wide variety of different reactions including hydrogenation of carbon monoxide (Tominaga, Aoki, and Nagai Citation2012), low-temperature water-gas shift reactions (Nagai and Matsuda Citation2006), hydrodeoxygenation of small organic molecules (Smirnov et al. Citation2017), photocatalytic decomposition of organic dyes (Mabuea, Swart, and Erasmus Citation2022), dry reforming of methane (Hirose, Ozawa, and Nagai Citation2011), etc. For the application of group VIII metal doped TMC in HER, Chen et al. demonstrated that doping molybdenum carbides with different transitions metals improved the electrocatalytic activity of HER over the parent β-Mo2C (Chen et al. Citation2018). It was shown that doping tungsten carbide with noble transition metals was effective for evolution of hydrogen from seawater at pH = 7 and is more stable than the commercial Pt/C (Lei et al. Citation2018). The introducing of a second metal can modify the crystal structure and enhance the catalytic activity of materials (Yu, Porosoff, and Chen Citation2012). Nickel (Ni) or cobalt (Co) can increase the catalytic activity of metal carbides in terms of reaction rate per site, and their adjacent atoms serve as additional active sites (Darband et al. Citation2019).
Xiong et al. (Citation2015) reported on the structural enhancement of β-Mo2C by doping the Mo-catalyst with Ni. Incorporating Ni atoms into the Mo2C lattice decreased both the Tafel slope and overpotential, making it suitable for HER. Zhu et al. (Citation2020) synthesised β-Mo2C catalysts doped with transition metals (Co, Ni, Cr and Fe) and studied the effects of the dopants on HER activity. Carbon-protected Co-WC was reported by Liu’s group (Liu et al. Citation2015) and their results showed that the exposed active sites during hydrogen production were mainly carbon sites because the carbides were wrapped with a carbonaceous metal during the preparation. The catalyst showed excellent activity (low overpotential of 73 mV) and stability (stable for ∼18 h). Co-doped Mo2C nanowires, prepared from an MoOx-amine precursor, with increased electron density assisted the HER kinetic process (Lin et al. Citation2016a, Citation2016b). In another study, a Ni-doped Mo2C hollow structure was prepared from a Ni-containing metal organic framework (MOF) with Mo-containing polyoxometalate guest, showing good HER reactivity (Xu, Nosheen, and Wang Citation2016).
During the synthesis of TMC using the carbothermal method, a variety of different carbon sources can be used including phenolic resin (Gao et al. Citation2018), acetone (Singla, Singh, and Pandey Citation2015), propane (Wang et al. Citation2006), and carbon black (Ha and Kim Citation2002). It was also shown that the temperature plays a crucial role in the amount of excess carbon present in the sample (Roohi, Alizadeh, and Fatehifar Citation2016). It was reported that when using multi walled carbon nanotubes (MWCNT) as the carbon source during the carbothermal reduction, agglomeration of the particles are prevented, and resulted in particles which exhibited better thermal resistance (Li et al. Citation2007).
This study reports the synthesis (using a carbothermal method and MWCNT as carbon source) and characterisation of both mono- and bi-group VIII metal doped transition metal tungsten-based and molybdenum-based carbides with the purpose of investigating and comparing their effectiveness in H2 production.
Experimental section
Materials
Nickel (II) nitrate hexahydrate [Ni(NO3)2·6H2O, 99.9%], ammonium metatungstate hydrate [(NH4)6H2W12O40]·xH2O, ≥85%], ammonium molybdate tetrahydrate [(NH4)6Mo7O24·4H2O, 83%], cobalt (II) nitrate hexahydrate [Co(NO3)2·6H2O, ≥98%] and multiwalled carbon nanotubes (MWCNTs, >98% carbon basis, O.D. × L 6–13 nm × 2.5–20 μm) were used in this study. These were all analytical grade purchased from Merck (Pty) Ltd. and were used without further purification.
Synthesis procedure
A solid-state reaction (carburisation method) was used to synthesise the bimetallic and trimetallic metal carbides. The process involves heating a mixture of the metals using a carbon-base source as reducing agent. After preparation, all the carbides were passivated in argon gas before exposed to air.
Synthesis of the bimetallic carbides; Ni-WC, Co-WC, Ni-MoC and Co-MoC
0.049 g (0.19 mmol; ∼15 eq) Ni(NO3)2·6H2O, 0.038 g (0.013 mmol; 1 eq) (NH4)6H2W12O40·xH2O and 0.013 g (0.07 mmol; ∼5 eq) MWCNTs were weighed. The powders were mixed homogeneously and ground using pestle and mortar resulting in a fine powder. The fine powder was then placed in a furnace for three hours, heated at 950°C in a H2-N2 mixture atmosphere. This resulted in the isolation of Ni-WC as a dark grey powder.
Furthermore, the other bimetallic carbides were synthesised by substituting ammonium metatungstate hydrate (NH4)6H2W12O40·xH2O with ammonium molybdate tetrahydrate (NH4)6Mo7O24·4H2O to obtain a grey Ni-MoC powder. Nickel (II) nitrate hexahydrate Ni(NO3)2·6H2O was substituted with cobalt (II) nitrate hexahydrate Co(NO3)2·6H2O to obtain a grey Co-MoC powder. All the other synthetic conditions were kept the same.
Synthesis of the trimetallic carbides; NiCo-WC and NiCo-MoC
0.062 g (0.24 mmol; ∼19 eq) Ni(NO3)2·6H2O, 0.028 g (0.096 mmol; ∼7.5 eq) Co(NO3)2·6H2O, 0.038 g (0.013 mmol; 1 eq) (NH4)6H2W12O40·xH2O and 0.013 g (0.07 mmol; ∼5 eq) of MWCNTs were mixed homogeneously in a pestle and mortar. The synthetic conditions kept the same as that of the bimetallic carbides. This resulted in the isolation of NiCo-WC as a black powder. Ammonium metatungstate hydrate (NH4)6H2W12O40·xH2O was substituted with ammonium molybdate tetrahydrate (NH4)6Mo7O24·4H2O to obtain NiCo-MoC as a black powder.
Characterisation
A Bruker X-ray diffraction (XRD) operated at a voltage of 40 kV and a current of 40 mA, using a Cu Kα radiation (λ = 1.546 Å) in the range between 20 and 80° was used for crystal structure evaluation. The average crystallite sizes (D) and lattice strains (ϵ) were determined by Williamson-Hall method
(1)
(1) where β is the is the full width at half maximum (FWHM), θ is the Bragg’s angle, K is the constant dependent on the crystallite shape (0.9), λ is the X-ray wavelength (0.15406 nm), D is the crystallite size, and ϵ is the strain. The graphs of βcosθ versus 4sinθ were plotted to determine ϵ from the slope of the plot and D from the y-intercept and equation (2)
(2)
(2) A JEOL JSM-7800F Field emission scanning electron microscopy (FE-SEM) equipped with an Oxford Aztec energy dispersive X-ray spectroscopy was used to study morphology and elemental composition. The electron beam voltage was maintained at 5 kV.
A PHI 5000 Versa probe-scanning X-ray photoelectron spectroscopy (XPS) equipped with an aluminium X-ray source (monochromatic Al Kα radiation hv = 1486.6 eV) generated by a 25 W, 15 kV electron beam was used to investigate the electronic and chemical states. A low energy neutraliser electron gun was used to minimise the charging of the samples. For high resolution spectra, the Hemi-spherical Analyzer pass energy was maintained at 93.90 eV with 0.1 eV step. The neat samples were held in place on the sample holder by means of carbon tape, and the samples were sputtered varying Ar+ ion beam energies in the range of 0.5–4.0 keV.
The electrochemistry was conducted on a BAS 100 B/W electrochemical workstation interfaced with a personal computer. Cyclic voltammetry (CV) was used to measure the current increase with a change in potential of the electrodes at a scan rate of 100 mV s−1.
Electrocatalytic application (electrode preparation)
A three-electrode electrochemical cell connected to a potentiostat was used to perform electrochemical measurements. The electrochemical cell consisted of a modified TiO2 flag electrode used as the working electrode, a Pt wire as the counter electrode and Ag/AgCl (0.1 M KCl) as the reference electrode. The electrocatalyst was prepared by dispersing 10 mg of the desired metal-WC in de-ionised water followed by ultrasonication. Nafion solution (1 wt%) was added to the electrocatalyst dispersion. The catalyst was coated onto one side of the surface of the TiO2 electrode (5 × 10 mm2), and air-dried. Nafion ensured that the catalyst was firmly kept in place while maintaining electrical conductance for the duration of the electrochemical experiments. A commercial Pt/C catalyst coated onto a TiO2 electrode (using the same procedure) was also measured for comparison.
Results and discussion
Structural analysis
The XRD patterns of the synthesised electrocatalysts are presented in . The patterns match well to their standard ICDD references (shown in ). After loading Co on Ni-WC and Ni-MoC, the patterns of the catalysts showed distinctive peaks which correspond to the characteristics of both Ni and Co carbides. Therefore, the diffraction peaks of NiCo-WC and NiCo-MoC are consistent with those of the bimetallic carbides. The dominant diffraction peaks increased in intensity, remained sharp and narrow indicating good crystallinity. However, the NiCo-WC catalyst has a set of weak peaks which correspond to the Co-WC. The incorporation of Co into Ni to form NiCo-MoC lead to the disappearance of peaks in the larger angles. presents the crystallite sizes and lattice strain caused by the lattice dislocations. These were determined by the Williamson-Hall method.
Table 1. Estimated crystallite sizes and lattice strains of the transition metal carbides.
Morphology and chemical composition analysis
shows SEM images and chemical composition of the electrocatalysts. The surface morphology of Ni-WC is not smooth, small spherical particles are noticeable on the bigger agglomerated particles. Co-WC show big spherically joined shaped liked particles. The trimetallic tungsten carbide catalyst (NiCo-WC) exhibits agglomerate spherical morphology. Sun et al. fabricated mesoporous Ni100-xCox alloys by electroless deposition using the lyotropic liquid crystals, the SEM images revealed spherical particles (Sun et al. Citation2017).
Figure 2. SEM images and EDS spectra of Ni-WC (a, d), Co-WC (b, e), NiCo-WC (c, f), Ni-MoC (g, j), Co-MoC (h, k), NiCo-MoC (i, l).
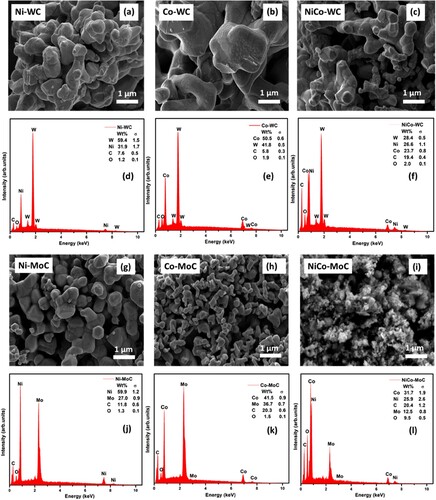
The surface morphology of Ni-MoC shows polygonal shaped particles consisting of smaller spherical particles on top of the larger ones. The catalyst Co-MoC exhibits particles with irregular morphology. The NiCo-MoC electrocatalyst consists of tiny agglomerated, spherical and irregular nanoparticles.
The chemical composition of the transition metal carbides shows an evenly distribution of elements on the surface. The spectra show percentages by weight of all the elements detected from EDS. The results reveal that the NiCo-WC and NiCo-MoC are mainly composed of Ni, Co, W, Mo and C indicating that the trimetallic carbides are a well-mixed nanostructure, consistent with the XRD data. Oxygen comes from the environmental conditions, as XRD showed that the structures formed did not contain oxygen.
Surface composition and chemical state analysis
XPS was used to study the surface composition of the NiCo-WC and NiCo-MoC electrocatalysts. The resulting data was analyzed with Multipak software v. 8.2. The XPS high-resolution peaks were fitted and charge corrected to the binding energy of the standard C 1s at 284.5 eV. The carbon is assigned to the adventitious hydrocarbons at 284.8 eV and carbide carbon (WC1-x) at 283.5 eV on the surface (Krasovskii et al. Citation2015; Saeed et al. Citation2021).
The high-resolution spectra of C, Ni, Co, W and Mo are shown in . Ni was deconvoluted into two main peaks, Ni 2p3/2 and Ni 2p1/2. The Ni 2p3/2 peak at a binding energy of 852.9 eV is ascribed to metallic Ni0. The peaks at 855.2 eV and its corresponding satellite structures situated at 859.6 eV are assigned to Ni2+, indicating that Ni was partly oxidised (Hu et al. Citation2021; Gerber and Erasmus Citation2018).
Co was deconvoluted to peaks located at binding energies of 778.7 and 793.7 eV assigned to Co (2p3/2 and 2p1/2, respectively) which represented the presence of metallic Co0. The Co 2p3/2 peak at 781.0 and 786.3 eV is assigned to the main and satellite structure, respectively, of Co2+ and/or Co3+ associated with the oxidised cobalt (Buitendach et al. Citation2016; Erasmus, Niemantsverdriet, and Swarts Citation2012).
The high-resolution spectra of W 4f reveal the 4f7/2–4f5/2 doublet. The W 4f7/2 peaks at a binding energy of 31.5 eV and 35.2 were assigned to WC and WO3, respectively. These binding energy assigned to the WC photoelectron lines are in accordance with the range values of 31.5–31.8 eV reported in literature for the WC1−x phase (Voevodin et al. Citation1999; Czyzniewski Citation2003; Abad et al. Citation2010). Partial surface oxidation occurred as revealed by the presence of the a 4f7/2–4f5/2 doublet between 35 and 38 eV corresponding to WO3 (Moulder et al. Citation1995).
The high-resolution Mo 3d XPS spectra revealed the peaks Mo 3d5/2 and 3d3/2. The Mo 3d5/2 peaks at binding energies of 228.7 and 230.0 eV are of lower valence states Mo2+ and Mo3+, which are assigned to the carbide. This correlates with reported literature for MoC1−x (Lin et al. Citation2016a, Citation2016b; Song et al. Citation2019; Wan, Regmi, and Leonard Citation2014; Wei et al. Citation2018). The Mo 3d3/2 peaks are of higher valence states Mo4+ and Mo6+ located at 231.4 and 232.6 eV (with a ca. ΔBE ≈ 3.1 eV) and are assigned to the partial oxidation (MoO2 and MoO3) of the MoC1−x surface (Lin et al. Citation2016a, Citation2016b; Song et al. Citation2019; Wang et al. Citation2020).
Electrocatalytic activities
The multiple scans of the cyclic voltammetry (CV) of the Ni-WC, Co-WC, NiCo-WC, Ni-MoC, Co-MoC and NiCo-MoC nanopowders coated onto TiO2 electrodes (5 × 10 mm2) in a 1.0 M NaOH solution are presented in . All the WC coated electrodes are bifunctional, implying they can electrocatalytically evolve both oxygen (in the positive potential region) and hydrogen (in the negative potential region). However, it can be seen that the electrocatalysts are more stable towards HER. The potential required to achieve the current to evolve the hydrogen gas is more constant than for OER.
shows the polarisation curves of the transition metal modified molybdenum-tungsten carbides towards HER. As stated above, commercial Pt/C (20 wt. %) was measured for comparison as the reference while the neat TiO2 electrode was measured as the blank. At a current density (j) of −10 mA cm−2, Ni-based carbides (Ni-WC and Ni-MoC) displayed best HER catalytic activity with the lowest overpotential of 1.421 V (see and ), which is marginally lower that the reference Pt/C. Co-WC, NiCo-WC, Co-MoC and NiCo-MoC displayed overpotentials of 1.458, 1.442, 1.508 and 1.473 V at a j of −10 mA cm−2, respectively.
Table 2. Electrocatalytic parameters of the studied electrocatalysts for HER.
Tafel analysis gives insight on the rate of the reaction on a surface. A Tafel plot is a logarithm of the current density vs potential. We then obtain Tafel parameters from the resulting linear portions of the curve as shown in . EquationEquation 3(3)
(3) is the Tafel equation used to fit the plots.
(3)
(3) where η is the overpotential, j is the current density, j0 is the exchange current density and b the Tafel slope also known as the Tafel coefficient.
The Tafel slopes of the studied catalyst are shown in . Lin et al. (Citation2016a, Citation2016b) and Sun et al. (Citation2017) studied catalysts and generated Tafel slopes for Pt/C as 31 mV dec−1. The Tafel slope of Pt/C in this work is 179 mV dec−1 with an overpotential of 1.425 V (the difference is due to type and size of the electrode used as well as the fact that only one side of the electrode was covered with the active species). The blank electrode, TiO2, has a Tafel slope of 168 mV dec−1. The Tafel slope of Ni-WC (135 mV dec−1) was smaller than Pt/C as well as the other prepared electrocatalysts, further confirming that it has good HER activity. The Tafel slopes for Co-WC and NiCo-WC are 156 and 161 mV dec−1, respectively.
The Tafel slope of the modified MoC electrodes are summarised in . Ni-MoC (161 mV dec−1) and Co-MoC (162 mV dec−1) displays the lowest Tafel slope, implying that these catalysts produce hydrogen the more effectively as compared to the Pt/C (179 mV dec−1).
The continuous stability of the catalysts over time at a set potential (half-wave potential) is shown in . Except for Co-WC and NiCo-WC, the current density of the modified electrodes at the applied potential decreased and stabilised with a slight decrease in current density. This indicates the modified electrodes showed reasonably good long-term durability towards HER.
Conclusion
The formation of the studied tungsten- and molybdenum based electrocatalysts was confirmed using XRD. The bimetallic transition metal doped carbides’ XRD patterns displayed peaks corresponding to both Ni and Co carbides, again affirming the bimetallic TMC was prepared. The presence of both metallic and 2+/3 + oxidation state deconvoluted peaks present in the XPS of the Ni, and Co implied that oxidation of the metals occurred. The XPS of Mo and W also showed the presence of the oxised and carbide peaks. All catalysts displayed HER catalytic activity. The Ni-based carbides (Ni-WC and Ni-MoC) displayed the best HER catalytic activity, while the Ni-WC electrocatalyst displayed better performance than the other WC. Its overpotential and Tafel slope were lower, even outperforming commercial Pt/C catalysts. It can be concluded that doping Mo and W carbides with Ni is more benficial towards HER than doping with Co or even bimetallic doping. During the stability test over 18 h, all the modified electrodes displayed reasonable stability towards HER.
Disclosure statement
No potential conflict of interest was reported by the author(s).
Additional information
Funding
References
- Abad, M. D., M. A. Muñoz-Márquez, S. El Mrabet, A. Justo, and J. C. Sánchez-López. 2010. “Tailored Synthesis of Nanostructured WC/a-C Coatings by Dual Magnetron Sputtering.” Surface and Coatings Technology 204 (21–22): 3490–3500. doi:10.1016/j.surfcoat.2010.04.019.
- Buitendach, B., E. Erasmus, J. Niemantsverdriet, and J. Swarts. 2016. “Properties of Manganese(III) Ferrocenyl-β-Diketonato Complexes Revealed by Charge Transfer and Multiplet Splitting in the Mn 2p and Fe 2p X-Ray Photoelectron Envelopes.” Molecules 21 (11): 1427. doi:10.3390/molecules21111427.
- Chen, M., Y. Ma, Y. Zhou, C. Liu, Y. Qin, Y. Fang, G. Guan, X. Li, Z. Zhang, and T. Wang. 2018. “Influence of Transition Metal on the Hydrogen Evolution Reaction Over Nano-Molybdenum-Carbide Catalyst.” Catalysts 8 (7): 294. doi:10.3390/catal8070294.
- Czyzniewski, A. 2003. “Deposition and Some Properties of Nanocrystalline WC and Nanocomposite WC/a-C:H Coatings.” Thin Solid Films 433 (1–2): 180–185. doi:10.1016/S0040-6090(03)00324-9.
- Darband, G. B., M. Aliofkhazraei, A. S. Rouhaghdam, and M. A. Kiani. 2019. “Three-Dimensional Ni-Co Alloy Hierarchical Nanostructure as Efficient Non-Noble-Metal Electrocatalyst for Hydrogen Evolution Reaction.” Applied Surface Science 465: 846–862. doi:10.1016/j.apsusc.2018.09.204.
- Erasmus, E., J. W. (Hans) Niemantsverdriet, and J. C. Swarts. 2012. “Preparation and Characterization of Supported Bimetallic PdIV–CoIII Model Catalyst from Organometallic Single Source Precursor for Aerobic Oxidation of Alcohols.” Langmuir 28 (47): 16477–16484. doi:10.1021/la3032978.
- Gao, S., H. Chen, Y. Liu, G.-D. Li, R. Gao, and X. Zou. 2019. “Surface-Clean, Phase-Pure Multi-Metallic Carbides for Efficient Electrocatalytic Hydrogen Evolution Reaction.” Inorganic Chemistry Frontiers 6 (4): 940–947. doi:10.1039/C8QI01360H.
- Gao, L., Y. Shi, Z. Yao, H. Gao, Y. Sun, F. Liang, and B. Jiang. 2018. “Phenolic Resin as a Carbon Source for the Synthesis of Monometallic Mo and Bimetallic CoMo Carbides via Carbothermal Reduction Route.” Phosphorus, Sulfur, and Silicon and the Related Elements 193 (5): 267–272. doi:10.1080/10426507.2017.1418740.
- Gerber, S. J., and E. Erasmus. 2018. “Surfactant-Stabilized Nano-Metal Hexacyanoferrates with Electrocatalytic and Heterogeneous Catalytic Applications.” Transition Metal Chemistry 43 (5): 409–420. doi:10.1007/s11243-018-0228-2.
- Gómez-Marín, A. M., and E. A. Ticianelli. 2017. “Effect of Transition Metals in the Hydrogen Evolution Electrocatalytic Activity of Molybdenum Carbide.” Applied Catalysis B: Environmental 209: 600–610. doi:10.1016/j.apcatb.2017.03.044.
- Gong, Q., Y. Wang, Q. Hu, J. Zhou, R. Feng, P. N. Duchesne, P. Zhang, et al. 2016. “Ultrasmall and Phase-Pure W2C Nanoparticles for Efficient Electrocatalytic and Photoelectrochemical Hydrogen Evolution.” Nature Communications 7 (1): 13216. doi:10.1038/ncomms13216.
- Greeley, J., T. F. Jaramillo, J. Bonde, I. Chorkendorff, and J. K. Nørskov. 2006. “Computational High-Throughput Screening of Electrocatalytic Materials for Hydrogen Evolution.” Nature Materials 5 (11): 909–913. doi:10.1038/nmat1752.
- Ha, G. H., and B. K. Kim. 2002. “Synthesis of Ultrafine WC/Co Powder by Mechanochemical Process.” Powder Metallurgy 45 (1): 29–32. doi:10.1179/003258902225001506.
- Hirose, T., Y. Ozawa, and M. Nagai. 2011. “Preparation of a Nickel Molybdenum Carbide Catalyst and Its Activity in the Dry Reforming of Methane.” Chinese Journal of Catalysis 32 (5): 771–776. doi:10.1016/S1872-2067(10)60185-4.
- Hu, Z., L. Zhang, J. Huang, Z. Feng, Q. Xiong, Z. Ye, Z. Chen, X. Li, and Z. Yu. 2021. “Self-Supported Nickel-Doped Molybdenum Carbide Nanoflower Clusters on Carbon Fiber Paper for an Efficient Hydrogen Evolution Reaction.” Nanoscale 13 (17): 8264–8274. doi:10.1039/D1NR00169H.
- Krasovskii, P. V., O. S. Malinovskaya, A. V. Samokhin, Y. V. Blagoveshchenskiy, VА Kazakov, and AА Ashmarin. 2015. “XPS Study of Surface Chemistry of Tungsten Carbides Nanopowders Produced Through DC Thermal Plasma/Hydrogen Annealing Process.” Applied Surface Science 339: 46–54. doi:10.1016/j.apsusc.2015.02.152.
- Lei, D., M. Nie, Y. Cao, W. Zuo, X. Tian, Z. Zhao, and Q. Li. 2018. “Properties of AuPdPt-WC/C Nanocomposite Catalyst in Simulated Seawater Solution for Hydrogen Evolution.” Materials Research Innovations 22 (4): 183–186. doi:10.1080/14328917.2017.1287491.
- Li, S., and B. Fei. 2022. “Two-Dimensional Transition Metal-Based Electrocatalyst and Their Application in Water Splitting.” Materials Science and Technology 38 (9): 535–555. doi:10.1080/02670836.2022.2062644.
- Li, X., D. Ma, L. Chen, and X. Bao. 2007. “Fabrication of Molybdenum Carbide Catalysts Over Multi-Walled Carbon Nanotubes by Carbothermal Hydrogen Reduction.” Catalysis Letters 116 (1–2): 63–69. doi:10.1007/s10562-007-9093-x.
- Lin, H., N. Liu, Z. Shi, Y. Guo, Y. Tang, and Q. Gao. 2016a. “Cobalt-Doping in Molybdenum-Carbide Nanowires Toward Efficient Electrocatalytic Hydrogen Evolution.” Advanced Functional Materials 26 (31): 5590–5598. doi:10.1002/adfm.201600915.
- Lin, H., Z. Shi, S. He, X. Yu, S. Wang, Q. Gao, and Y. Tang. 2016b. “Heteronanowires of MoC–Mo2C as Efficient Electrocatalysts for Hydrogen Evolution Reaction.” Chemical Science 7 (5): 3399–3405. doi:10.1039/C6SC00077K.
- Liu, Y., G.-D. Li, L. Yuan, L. Ge, H. Ding, D. Wang, and X. Zou. 2015. “Carbon-Protected Bimetallic Carbide Nanoparticles for a Highly Efficient Alkaline Hydrogen Evolution Reaction.” Nanoscale 7 (7): 3130–3136. doi:10.1039/C4NR06295G.
- Mabuea, B. P., H. C. Swart, and E. Erasmus. 2022. “Photocatalytic Decomposition of an Azo Dye Using Transition-Metal-Doped Tungsten and Molybdenum Carbides.” ACS Omega 7 (27): 23401–23411. doi:10.1021/acsomega.2c01727.
- Miao, M., J. Pan, T. He, Y. Yan, B. Y. Xia, and X. Wang. 2017. “Molybdenum Carbide-Based Electrocatalysts for Hydrogen Evolution Reaction.” Chemistry – A European Journal 23 (46): 10947–10961. doi:10.1002/chem.201701064.
- Michalsky, R., Y.-J. Zhang, A. J. Medford, and A. A. Peterson. 2014. “Departures from the Adsorption Energy Scaling Relations for Metal Carbide Catalysts.” The Journal of Physical Chemistry C 118 (24): 13026–13034. doi:10.1021/jp503756g.
- Moulder, J. F., W. F. Stickle, P. E. Sobol, and K. D. Bomben. 1995. Handbook of X-Ray Photoelectron Spectroscopy: A Reference Book of Standard Spectra for Identification and Interpretation of XPS Data. Chigasaki: ULVAC-PHI, Inc.
- Nagai, M., and K. Matsuda. 2006. “Low-Temperature Water–Gas Shift Reaction Over Cobalt–Molybdenum Carbide Catalyst.” Journal of Catalysis 238 (2): 489–496. doi:10.1016/j.jcat.2006.01.003.
- Roohi, P., R. Alizadeh, and E. Fatehifar. 2016. “Dry Reforming of Methane Over Nano-Mo2C/Al2O3 Catalyst: Effect of Carburization Conditions on Excess Carbon Deposition.” Energy Sources, Part A: Recovery, Utilization, and Environmental Effects 38 (24): 3565–3571. doi:10.1080/15567036.2016.1198848.
- Saeed, N. A. M., E. Coetsee, R. E. Kroon, M. Bettinelli, and H. C. Swart. 2021. “Photoluminescence of Bi3+ Doped in YOF Phosphor as an Activator.” Optical Materials 119: 111291. doi:10.1016/j.optmat.2021.111291.
- Sapountzi, F. M., J. M. Gracia, C. J. (Kees-J.) Weststrate, H. O. A. Fredriksson, and J. W. (Hans) Niemantsverdriet. 2017. “Electrocatalysts for the Generation of Hydrogen, Oxygen and Synthesis Gas.” Progress in Energy and Combustion Science 58: 1–35. doi:10.1016/j.pecs.2016.09.001.
- Singla, G., K. Singh, and O. P. Pandey. 2015. “Effect of Processing Variables on WC Nanoparticles Synthesized by Solvothermal Route.” Particulate Science and Technology 33 (1): 47–52. doi:10.1080/02726351.2014.933147.
- Smirnov, A. A., Z. Geng, S. A. Khromova, S. G. Zavarukhin, O. A. Bulavchenko, A. A. Saraev, V. V. Kaichev, D. Y. Ermakov, and V. A. Yakovlev. 2017. “Nickel Molybdenum Carbides: Synthesis, Characterization, and Catalytic Activity in Hydrodeoxygenation of Anisole and Ethyl Caprate.” Journal of Catalysis 354: 61–77. doi:10.1016/j.jcat.2017.07.009.
- Song, H. J., M. Sung, H. Yoon, B. Ju, and D. Kim. 2019. “Ultrafine Α-Phase Molybdenum Carbide Decorated with Platinum Nanoparticles for Efficient Hydrogen Production in Acidic and Alkaline Media.” Advanced Science 6 (8): 1802135. doi:10.1002/advs.201802135.
- Sun, T., J. Cao, J. Dong, H. Du, H. Zhang, J. Chen, and L. Xu. 2017. “Ordered Mesoporous Ni Co Alloys for Highly Efficient Electrocatalytic Hydrogen Evolution Reaction.” International Journal of Hydrogen Energy 42 (10): 6637–6645. doi:10.1016/j.ijhydene.2017.01.071.
- Tominaga, H., Y. Aoki, and M. Nagai. 2012. “Hydrogenation of CO on Molybdenum and Cobalt Molybdenum Carbides.” Applied Catalysis A: General 423–424: 192–204. doi:10.1016/j.apcata.2012.02.041.
- Voevodin, A. A., J. P. O’Neill, S. V. Prasad, and J. S. Zabinski. 1999. “Nanocrystalline WC and WC/a-C Composite Coatings Produced from Intersected Plasma Fluxes at Low Deposition Temperatures.” Journal of Vacuum Science & Technology A: Vacuum, Surfaces, and Films 17 (3): 986–992. doi:10.1116/1.581674.
- Wan, C., Y. N. Regmi, and B. M. Leonard. 2014. “Multiple Phases of Molybdenum Carbide as Electrocatalysts for the Hydrogen Evolution Reaction.” Angewandte Chemie 126 (25): 6525–6528. doi:10.1002/ange.201402998.
- Wang, X.-H., H.-L. Hao, M.-H. Zhang, W. Li, and K.-Y. Tao. 2006. “Synthesis and Characterization of Molybdenum Carbides Using Propane as Carbon Source.” Journal of Solid State Chemistry 179 (2): 538–543. doi:10.1016/j.jssc.2005.11.009.
- Wang, J., H. Wei, X. Chen, C. Chen, and X. Chen. 2020. “Facile Preparation of N, P Co-Doped Molybdenum Carbide / Porous Carbon Rough Microspheres for Efficient Electrocatalytic Hydrogen Evolution.” International Journal of Hydrogen Energy 45 (1): 595–604. doi:10.1016/j.ijhydene.2019.10.241.
- Wei, H., Q. Xi, X. Chen, D. Guo, F. Ding, Z. Yang, S. Wang, J. Li, and S. Huang. 2018. “Molybdenum Carbide Nanoparticles Coated Into the Graphene Wrapping N-Doped Porous Carbon Microspheres for Highly Efficient Electrocatalytic Hydrogen Evolution Both in Acidic and Alkaline Media.” Advanced Science 5 (3): 1700733. doi:10.1002/advs.201700733.
- Xiong, K., L. Li, L. Zhang, W. Ding, L. Peng, Y. Wang, S. Chen, S. Tan, and Z. Wei. 2015. “Ni-Doped Mo2C Nanowires Supported on Ni Foam as a Binder-Free Electrode for Enhancing the Hydrogen Evolution Performance.” Journal of Materials Chemistry A 3 (5): 1863–1867. doi:10.1039/C4TA05686H.
- Xu, X., F. Nosheen, and X. Wang. 2016. “Ni-Decorated Molybdenum Carbide Hollow Structure Derived from Carbon-Coated Metal–Organic Framework for Electrocatalytic Hydrogen Evolution Reaction.” Chemistry of Materials 28 (17): 6313–6320. doi:10.1021/acs.chemmater.6b02586.
- Yu, W., M. D. Porosoff, and J. G. Chen. 2012. “Review of Pt-Based Bimetallic Catalysis: From Model Surfaces to Supported Catalysts.” Chemical Reviews 112 (11): 5780–5817. doi:10.1021/cr300096b.
- Zhu, J., L. Hu, P. Zhao, L. Y. S. Lee, and K.-Y. Wong. 2020. “Recent Advances in Electrocatalytic Hydrogen Evolution Using Nanoparticles.” Chemical Reviews 120 (2): 851–918. doi:10.1021/acs.chemrev.9b00248.